- 1Translational Medicine Center, Beijing Chest Hospital, Capital Medical University, Beijing, China
- 2Beijing Key Laboratory in Drug Resistant Tuberculosis Research, Beijing Tuberculosis and Thoracic Tumor Research Institute, Beijing, China
Tumor immunotherapy mainly relies on activating the immune system to achieve antitumor treatment. However, the present tumor immunotherapy used in the clinic showed low treatment efficacy with high systematic toxicity. To overcome the shortcomings of traditional drugs for immunotherapy, a series of antitumor immunotherapies based on nanomaterials have been developed to enhance the body’s antitumor immune response and reduce systematic toxicity. Due to the noninvasiveness, remote controllability, and high temporal and spatial resolution of light, photocontrolled nanomaterials irradiated by excitation light have been widely used in drug delivery and photocontrolled switching. This review aims to highlight recent advances in antitumor immunotherapy based on photocontrolled nanomaterials. We emphasized the advantages of nanocomposites for antitumor immunotherapy and highlighted the latest progress of antitumor immunotherapy based on photoactivated nanomaterials. Finally, the challenges and future prospects of light-activated nanomaterials in antitumor immunity are discussed.
1 Introduction
Cancer is one of the main causes of human death (Zaimy et al., 2017). Statistics show that there will be an estimated 19.3 million new cancer cases and nearly 10 million cancer deaths worldwide in 2020 (Siegel et al., 2021; Sung et al., 2021). Therefore, the exploration of early diagnosis and effective treatment methods of cancer have attracted much attention. The traditional clinical methods of tumor treatment are mainly surgery, chemotherapy and radiotherapy (Burugu et al., 2017; Hojman et al., 2018), which have defects, such as poor efficacy and high toxicity (Zeng et al., 2021). In recent years, immunotherapy has become a promising method for the treatment of malignant tumors (Riley et al., 2019; Abbott & Ustoyev, 2019; Igarashi & Sasada, 2020; Yang F. et al., 2020). Immunotherapy is the artificial enhancement or suppression of the body’s immune function in the presence of hypo- or hyperfunctioning organisms for the purpose of treating disease. Tumor immunotherapy aims to improve the overall adaptability of the immune system by modulating key immune mechanisms (Topalian, 2017) and redirecting adaptive immune cells to destroy tumor-specific targets (June et al., 2018). To date, a variety of tumor immunotherapy methods have been discovered (Figure 1) (Chauhan et al., 2021; Kumar et al., 2021; Lesch & gill, 2021; Guo et al., 2022), including immune checkpoint blockade (Ribas & Wolchok, 2018; Havel et al., 2019; He & Xu, 2020), cancer vaccines (Duong et al., 2018; DeMaria & Bilusic, 2019; Shemesh et al., 2021), cell therapy (Fry et al., 2018; Mohanty et al., 2019; Wang et al., 2020), immunomodulatory small molecules (Cukier et al., 2017; Berraondo et al., 2019; Gracia, et al., 2019), etc. However, emerging tumor immunotherapy methods still face enormous challenges, such as the low efficacy of targeted drug therapy and the inherent toxicity of immunotherapy drugs, which may lead to severe inflammatory responses and autoimmune diseases (Emens et al., 2017; Kroschinsky et al., 2017). Therefore, it is essential to find a safer and more controllable method for tumor immunotherapy.
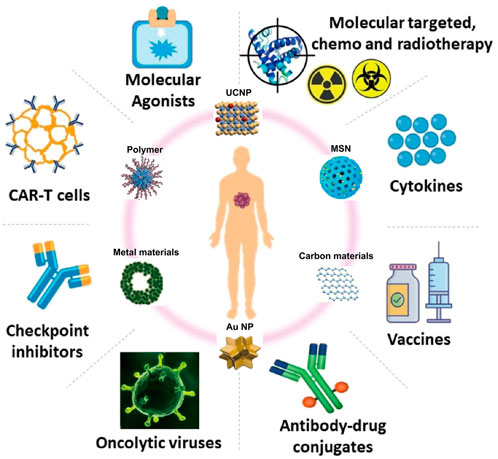
FIGURE 1. Tumor immunotherapy. (Reproduced from Chauhan et al., 2021, International Journal of Molecular Science; Guo et al., 2022, Biomaterials).
With the rapid development of nanotechnology, the clinical application of nanomaterials is also increasing (Ulbrich et al., 2016; Zang et al., 2017; Cheng et al., 2021). Due to their special physical and chemical properties, nanoparticles have significant potential therapeutic effects in tumor immunotherapy (Velpurisiva et al., 2017; Park et al., 2018; Irvine & Dane, 2020; Muluh et al., 2021). Tumor immunotherapy mainly relies on efficient drug delivery and targeted tumor therapy. Nanomaterials are used as transport carriers to form stable nanocomplexes through encapsulation or combination, which can improve the efficacy of tumor immunotherapy and reduce drug toxicity (Gonçalves et al., 2020). Furthermore, nanomaterials can enhance the drug delivery efficiency in an active or passive manner (enhanced permeability and retention (EPR) effect) (Liu et al., 2018; Kang et al., 2020; Li et al., 2022), enabling the delivery of drugs, antibodies or other immunotherapeutic agents to preferentially accumulate at the tumor site (Jain & Stylianopoulos, 2010), which can minimize side effects and improve therapeutic efficacy (Duong et al., 2018).
To date, phototherapy has attracted extensive attention in clinical treatment due to its remote controllability, high temporal and spatial resolution, noninvasiveness and high selectivity (Li J. et al., 2019; Wang M. et al., 2019; Zhao et al., 2019). At present, light-responsive nanomaterials mainly include organic materials (photosensitizers, fluorophores and carbon-based nanoparticles) and inorganic-based nanoparticles (quantum dots, upconverting nanoparticles and gold nanoparticles) (Choi & Frangioni, 2010; Son et al., 2019). Light-activated therapy is less invasive, much more precise and safer than traditional treatments such as chemotherapy, surgery and radiation (Riley et al., 2018).
UV light is most commonly used light for photocontrolled drug delivery, release or response owing to its capability to trigger a structural change in light-responsive systems. These photochemical reaction processes then lead to nanoparticle disassembly and the subsequently controllable release of payloads. Most UV light responsive nanomaterials have been modified with photocleavable terminal groups, photocleavable side chains or multiphotocleavable linkers (Yan et al., 2013; Sun et al., 2018), and the most commonly used photocleavable protecting groups are o-nitrobenzyl and coumarin derivatives. According to the photochemical reaction mechanisms, light-induced structural changes are often divided into three major processes: 1) photocleavage of light-responsive units, 2) photoisomerization, and 3) photocrosslinking/-decrosslinking (Zhao et al., 2019). However, light in the ultraviolet‒visible region has poor penetration ability in biological tissues and is harmful to the skin, while near-infrared (NIR) light has deeper tissue penetration and low toxicity, so the application of NIR light to trigger tumor therapy has stronger application potential (Wang et al., 2013; Yan & Li, 2016). The mechanism of converting NIR light irradiation into UV light is discussed in the section on upconversion nanoparticles.
For immune activation triggered by light-activated nanomaterials, the strategies mainly include using light to activate cancer vaccines, chimeric antigen, receptor (CAR)-T-cell therapy, immune checkpoint blockade (ICB) therapy, cytokine therapy, and immune adjuvant therapy (Chu et al., 2021). Phototherapy can enhance the therapeutic effect by amplifying antitumor immunity, reversing the tumor immunosuppressive microenvironment (TIME) and enhancing the effect of immunotherapy by producing an extremely immunogenic tumor microenvironment (TME) (Li H. et al., 2020; Shi et al., 2020). Phototherapy can be combined with immunotherapy to eliminate metastatic tumors. Moreover, when used in combination with conventional immunotherapy, phototherapy can promote the maturation of APCs to initiate immune responses (Jiang et al., 2020).
In recent years, a variety of photocontrolled nanomaterials have been developed, such as gold nanoparticles, carbon nanomaterials, and upconversion nanoparticles (Boyer et al., 2010; Zhang et al., 2016). Here, we mainly review the application of photocontrolled nanomaterials in antitumor immunotherapy.
2 Light-activated nanomaterials for tumor immunotherapy
2.1 Polymer nanomaterial-based antitumor immunity
Polymer-based nanoparticles can serve as excellent carriers for delivering biomolecules, drugs, genes and vaccines to tumor sites in vivo (Wei et al., 2021). Among them, conjugated polymers (CPs) have strong light absorption ability, good stability and biocompatibility in the NIR region. Xuan et al. reported an optogenetic system mediated by conjugated polymer nanoparticles (CPNs), which could activate immunotherapy in situ under NIR irradiation (Fu et al., 2021). Illumination of CPNs with NIR drives the heat shock promoter (HSP70) to trigger gene transcription of the interferon-γ (IFN-γ) cytokine. IFN-γ secreted by tumor cells induces the activation of surrounding tumor-associated macrophages through the IFN-γ-JAK-STA1 signaling pathway, which induces cancer cell killing through immunotherapy (Figure 2A).
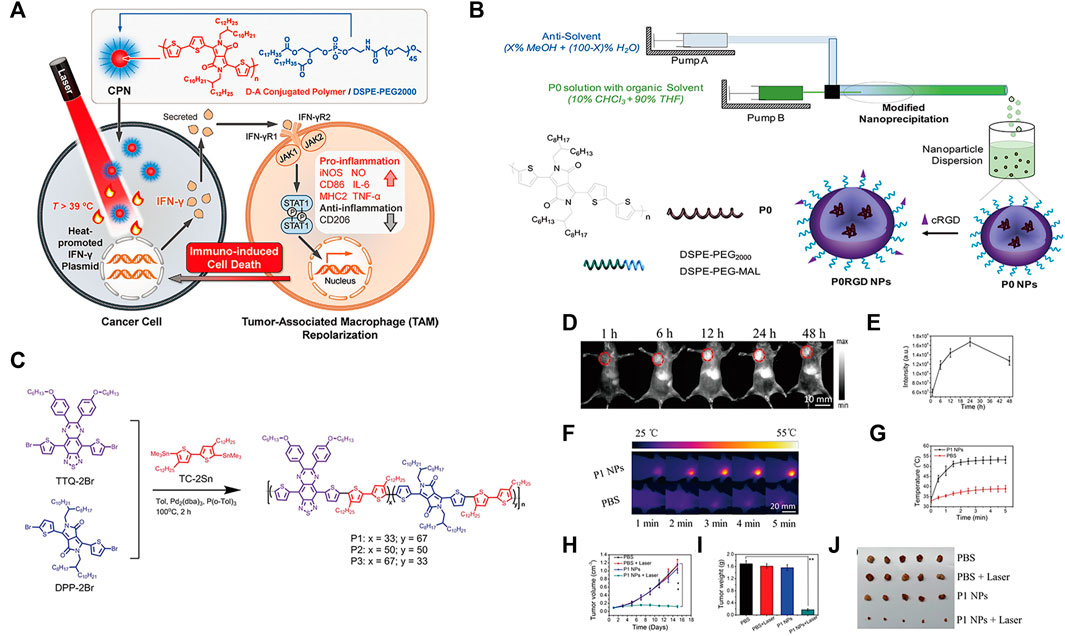
FIGURE 2. (A) Schematic illustration of photothermal conjugated polymer nanoparticles (CPN) for remote control cancer immunotherapy (Reproduced from Fu et al., 2021, Advance Materials). (B) Schematic illustration of a microfluidic glass capillary mixer for the synthesis of PORGD NPs. (Reproduced from Wang M. et al., 2019, ACS Applied Materials & Interfaces). (C) Synthetic route and chemical structure of three conjugated polymers. (D) NIR-II fluorescence images of mice with 4T1 tumors at different time points after injection with P1 NPs under 808 nm illumination. (E) Quantitative NIR-II fluorescence signal intensity corresponding to the tumor sites at different time points in (D). (F) Corresponding infrared photothermal images of mice with 4T1 tumors after injection with PBS and P1 NPs under 1,064 nm laser excitation (G) Corresponding temperature changes at the tumor sites. (H) Tumor volumes and (I) weight growth curves of mice with 4T1 tumors treated with the four treatment groups at different time points. (J) Photo of excised tumors after 15 days of therapy. (Reproduced from Chen C. et al., 2021, Journal Materials Chemistry B).
Moreover, CPs can be facilely designed by using molecular engineering to possess certain electrical and optical properties for optimal photothermal therapy (PTT) performance (Tuncel & Demir, 2010; Guo et al., 2017; Qian et al., 2017). Wang et al. first demonstrated the synthesis of conjugated polymer nanoparticles (CP NPs) with a uniform diameter of 52 nm as PTT agents by using a modified nanoprecipitation process (Wang S. et al., 2019). Under 808 nm laser illumination, the thiolated cyclo (Arg-Gly-Asp-D-Phe-Lys (mpa)) peptide (c-RGD)-functionalized CP NPs exhibited high photothermal conversion efficiency, which activated a proinflammatory immune response and induced effective cancer cell death (Figure 2B). Furthermore, studies have found that NIR-Ⅱ light reduces light scattering and photon absorption in biological tissues, so it has better spatial resolution and lower autofluorescence intensity than traditional NIF-FI (700–900 nm) (Zhang D. X. et al., 2019; Hu et al., 2020a; Zhang et al., 2020a; Hu et al., 2020b; Yang J. et al., 2020). Thus, CPs are designed for NIR-Ⅱ because of their changeable chemical structures, adjustable NIR absorption, large Stokes shift, high extinction coefficient, and superior biocompatibility (Lin et al., 2017; Yin et al., 2017; Li T. et al., 2019; Li X. et al., 2019; Cui et al., 2020). Chen et al. designed and developed nanoparticles based on double-acceptor conjugated polymers (P1 NPs) for application in NIR-Ⅱ FI and NIR-Ⅱ PTT (Chen C. et al., 2021) (Figure 2C). The in vivo experiments demonstrated that P1 NPs not only exhibited high accumulation and a high sign-to background ratio (SBR) of vascular imaging at the tumor sites but also showed excellent NIR-II PTT efficiency for tumor treatment (Figures 2D–J).
Tumor cells can escape T-cell-mediated cytotoxicity using the programmed cell death protein 1 (PD-1)/programmed cell death 1 ligand 1 (PD-L1) immune checkpoint (Goodman et al., 2017), so blocking the PD-1/PD-L1 checkpoint has been extensively studied in antitumor immunity (Akinleye & Rasool, 2019). Yu et al. designed a synthetic nanoparticulated PD-L antagonist consisting of poly (ethylene glycol)-poly (lactic acid-coglycolic acid) (PEG-PLGA) nanoparticles decorated with a PD-L1 binding peptide. Nanoparticles can accumulate in the tumor site and mediate strong photothermal effects, eliminate primary tumors treated by near infrared radiation and cause strong antitumor immunity by inducing immunogenic cell death (ICD) (Yu et al., 2022).
Semiconducting polymer nanoparticles (SNPs) are transformed from semiconducting polymers (SPs), which are composed of highly π-conjugated backbones (Li W. et al., 2020). Compared with most semiconductor inorganic nanoparticles, SNPs have good biocompatibility and optical properties, as well as excellent optical stability (Jiang B. P. et al., 2019; Li & Pu, 2020; Zhou et al., 2020; Zhen et al., 2021). Zhang et al. reported a semiconducting polymer nano-PROTAC (SPNpro) with phototherapy and activatable protein degrading capabilities for photoimmunometabolic cancer therapy (Zhang et al., 2021). Under NIR light irradiation, SPNpro can generate singlet oxygen to eliminate tumor cells and induce immunogenic cell death (ICD) to enhance tumor immunogenicity. In addition, cathepsin B, a cancer biomarker, can specifically activate the PROTAC of SPNpro, triggering the targeted proteolysis of immunosuppressant indoleamine 2,3-dioxygenase (IDO) in tumors. Sustained IDO degradation blocked the catabolic process of tryptophan (Trp) and promoted the activation of effector T cells (Figures 3A,B).
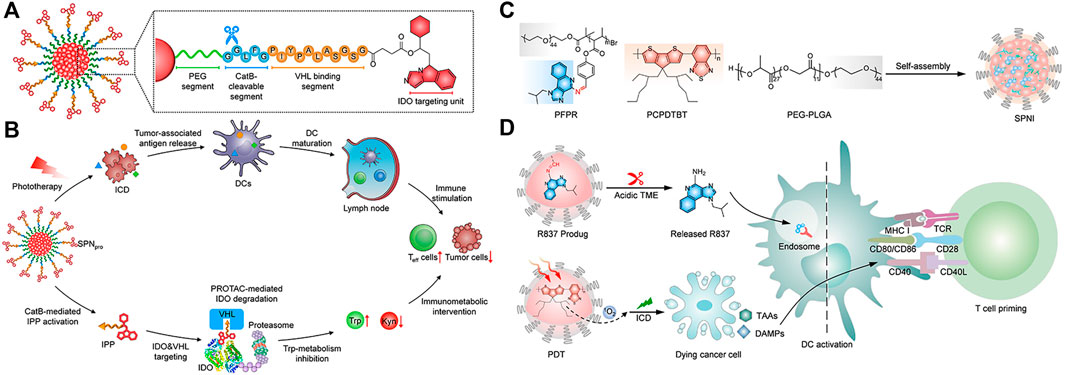
FIGURE 3. (A) Structure and cathepsin B (CatB)-specific activation mechanism of SPN pro. (B) SPN-mediated activation of two processes of photoimmunometabolic therapy. (Reproduced from Zhang et al., 2021, Nature Communitions). (C) Chemical structures of PFPR, PEG-PLGA, PCPDTBT, and the preparation of SPNI. (D) The process of immune activation mediated by SPNI-based precision photodynamic immunotherapy. (Reproduced from Liu et al., 2022, Advance Materials).
Liu et al. reported an amphipathic semiconductor polymer nanoimmunomodulator (SPNI) that absorbed NIR light to achieve PDT (Zhu et al., 2017) and conjugated with a Toll-like receptor 7 (TLR7) agonist (imiquimod: R837) via an acid-liable Schiff base linker (Figure 3C) (Liu et al., 2022). Introduction of R837 triggers ligation of TLR7 in endosomal membrane localization to promote DC maturation and secretion of proinflammatory cytokines (Lee et al., 2003). Under NIR light, SPNI has the photodynamic effect of direct tumor killing and death of immunogenic cancer cells. The synergistic action of the released immunogenic factor and TLR7 agonist activated by the acidic tumor microenvironment (TME) can be used as a tumor vaccine in situ with strong antitumor activity (Figure 3D) (Liu et al., 2022). Lyu et al. utilized the enzymatic oxidation properties of vinylidene bonds in combination with polymers to synthesize biodegradable semiconductor polymers (DPPV) and convert them into water-soluble nanoparticles (SPNV), which can enhance PA and PTT efficiencies for cancer therapy (Lyu et al., 2018). Wei et al. designed and synthesized a novel diketopyrrolopyrrole polymer nanoparticle [P(AcIID-DPP)], which exhibited strong light absorption and excellent photothermal conversion in the NIR-I to NIR-II optical region. capacity, high biocompatibility and photostability (Wei et al., 2018). In addition, nanoparticles can be efficiently absorbed by cancer cells and thermally ablated under NIR-II laser irradiation, exhibiting excellent anticancer effects. Jiang et al. synthesized an amphiphilic semiconductor polymer (PEG-PCB) that can not only be used as a diagnostic component in NIR fluorescence and PA imaging but also enable effective NIR fluorescence/PA imaging-guided photothermal therapy (Jiang et al., 2017).
Polylactic glycolic acid (PLGA) has controlled and sustained-release properties, low toxicity, and good biocompatibility and can be used for drug delivery, cancer imaging and therapy (Clawson et al., 2010; Danhier et al., 2012; Sadat Tabatabaei Mirakabad et al., 2014; Jia et al., 2018). Chen et al. discovered a kind of PLGA-IGG-R837 nanoparticle coated with the photothermal agent indocyanine green (IGG) and TLR7 ligand R837 (Chen et al., 2016). Under NIR light irradiation, PLGA-IGG-R837 ablated tumors by photothermal action and released tumor-associated antigens. Nanoparticle adjuvants loaded with R837 showed vaccine-like function, leading to immune responses. Luo et al. prepared biodegradable PLGA nanoparticles coloaded with hollow gold nanoshells (HAuNS) and anti-PD-1 peptide (APP) (AA@PN). NIR irradiation can not only trigger the release of APP and maintain a long-term immune response in vivo but also enable HAuNS to produce a photothermal effect to ablate tumors. The combined effect of NIR and HAuNS can produce a stronger antitumor effect (Luo et al., 2018).
2.2 Small molecule nanomedicine-based antitumor immunity
Small molecule nanomedicines (SMNs) refer to nanoscale drug delivery systems assembled from small molecule drugs (Ma et al., 2016; Wang Y. et al., 2017; Cheetham et al., 2017). Compared with traditional nanomedicines with complex preparation and possible toxicity of carrier materials, small molecule nanomedicines have been extensively studied (Luo et al., 2016; Li G. et al., 2021). All-drug small-molecule nanomedicines show excellent antitumor effects due to the synergistic effect of different drugs, but they have untraceable and undetermined defects (Xue et al., 2020). Adding photosensitizers to small-molecule nanomedicines can not only achieve light control but also enhance the effect of antitumor immunotherapy in combination with photodynamic therapy or photothermal therapy (Xue et al., 2019).
Li et al. self-assembled small-molecule nanoparticles by the interaction of photosensitizer ICG and epirubicin (EPI) in aqueous solution. ICG-EPI NPs exerted an excellent photothermal effect to ablate tumors under NIR laser irradiation and combined with chemotherapy drugs to further enhance the antitumor effect (Li et al., 2017). Zhang et al. assembled nanoparticles (DINP) using the hydrophobic drugs doxorubicin (DOX) and ICG and coated their surface with ruptured cancer cell membranes to form novel NIR-responsive and highly targeted small-molecule nanoparticles (DOX NPs@ICG@CCCM, DICNPs) (Zhang H. et al., 2018). The cancer cell membrane can enable DICNPs to target the tumor site. After reaching the tumor site, the cancer cell membrane was destroyed under NIR light irradiation to rapidly release DOX and ICG, thereby producing efficient chemical and photothermal effects to achieve antitumor immunotherapy. Zhang et al. assembled amphiphilic amino acids (9-fluorenylmethoxycarbonyl-L-leucine, Fmoc-ll) and photosensitive drugs (Ce6) with metal ions (Mn2+) to form an amino acid-porphyrin-Mn complex nanoplatform (FMCNPs) (Zhang N. et al., 2018). FMCNPs had high drug-loading capacity, good biocompatibility and MRI function and showed excellent tumor accumulation and photodynamic effects under NIR irradiation, which can effectively ablate tumors.
2.3 Porous silicon nanoparticle-based antitumor immunity
Due to its unique optical properties and biodegradability, porous silicon has been widely used in biomedical fields, such as drug delivery, biosensors and imaging (Martín-Palma et al., 2014; Li et al., 2018; Zhang R. et al., 2019; Tieu et al., 2021). Li et al. designed a selective photothermal and weak immunostimulatory nanovaccine based on porous silicon composite nanomaterials (Li J. et al., 2021). Porous silicon nanoparticles (PSiNPs) had a significant immunostimulatory effect on immune cells after special treatment and were coated with the cancer cell membrane (CCM) to obtain the CCM@PSiNPs@Au nanovaccine (Figure 4). Under irradiation with NIR light, immunostimulatory vaccines are released, which can induce the antitumor immune response of the body and control the overproduction of cytokines by immune cells, further enhancing the therapeutic effect of PTT.
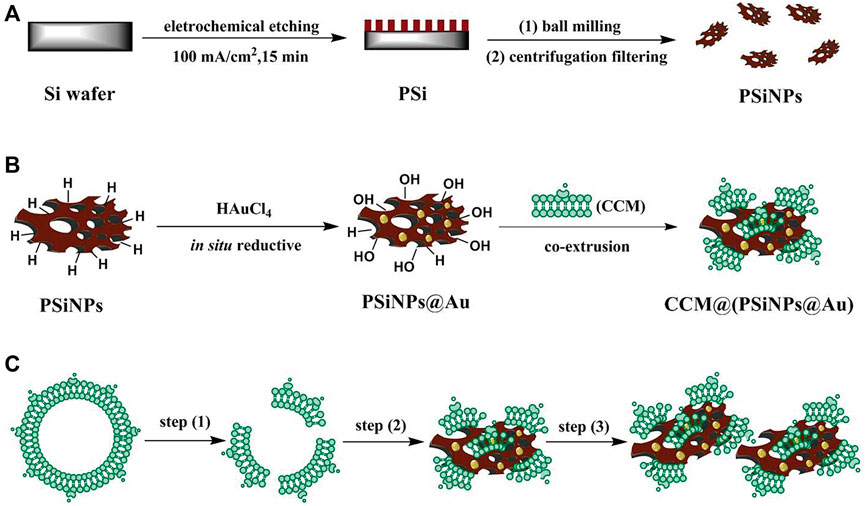
FIGURE 4. (A–C) The schematic simulation of (A) the fabrication of PSiNPs, (B) PSiNPs@Au and CCM@(PSiNPs@Au) samples; and (C) the aggregation mechanism of particles induced by CCM coating during their coextrusion. (Reproduced from Li G. et al., 2021, Advance Materials).
NIR dye IR780 is a biodegradable photothermal and imaging agent that can be loaded into mesoporous silica nanoparticles (MSNs) to form biodegradable cores (Jiang et al., 2015; Zhan et al., 2017). Ma et al. coated IR780-loaded MSNs (IMs) with a prefabricated CAR-T membrane to prepare tumor-specific CAR-T membrane-wrapped nanoparticles (CIMSs) (Ma et al., 2020). Experiments in vitro and vivo show that CIMS has stronger tumor targeting and antitumor ability.
MSNPs, with higher intrinsic stability, higher drug loading and larger surface area, can deliver effective concentrations of drugs to tumor sites, which provides a new research direction for targeted drug delivery (Moradipour et al., 2020; Barkat et al., 2021; Ghaferi et al., 2021; Wang et al., 2021; Sheng et al., 2022; Xie et al., 2022), such as the delivery of anti-miR therapeutics (Zhang et al., 2014; Bertucci et al., 2015; Yu et al., 2016; Khatami et al., 2021). Yue et al. developed a multifunctional nanoplatform (MPSNs@R837) formed by mesoporous hexagonal core-shell zinc porphyrin silica nanoparticles (MPSNs) loaded with R837 (Toll-like receptor 7 agonist) (Yue et al., 2022). In the presence of light sources, MPSNs@R837 can effectively destroy primary tumors through PTT and PDT. In addition, the loaded immune adjuvant R837 can be functionalized with tumor-associated antigens, promote the maturation of DCs and trigger a strong immune response (Figure 5A).
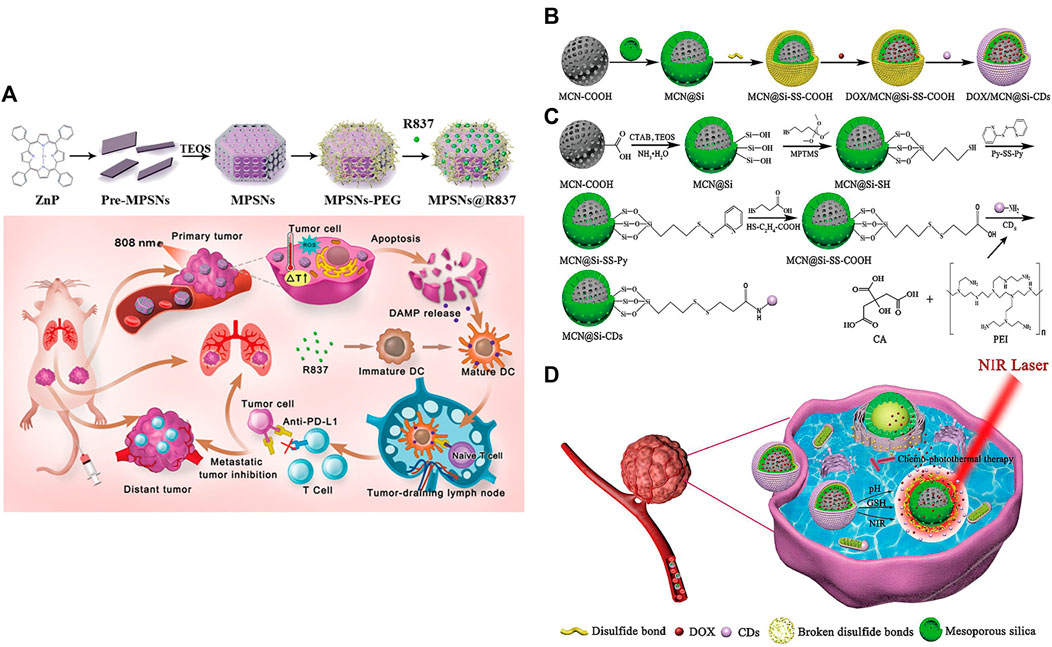
FIGURE 5. (A) Schematic illustration of the preparation of the core-shell zinc porphyrin nanoplatform (MPSNs@R837) and its use for synergistic antitumor immunity. (Reproduced from Yue et al., 2022, Journal of Nanobiotechnology). (B–D) Synthesis process of (B) DOX/MCN@Si-CDs and (C) MCN@Si-CDs. (D) Schematic illustration of chemo-photothermal synergistic therapy against tumors. (Reproduced from Lu et al., 2020, Colloids and Surfaces B: Biointerfaces).
However, MSNPs suffer from low biocompatibility and dispersibility, premature drug release, and interaction with erythrocyte membranes, leading to hemolysis (Bharti et al., 2015; Zhang et al., 2017). Lu et al. constructed multistimuli-responsive mesoporous silica-coated carbon nanoparticles (DOX/MCN@Si-Cd) with high drug loading capacity and high photothermal conversion efficiency (Lu et al., 2020). The appropriate size of carbon dots (Cd) prevented the premature release of DOX (Hu et al., 2016); DOX was released rapidly at low pH and high glutathione (GSH) concentrations (Cheng et al., 2011; Cui et al., 2012; Duan et al., 2019). Local high temperature generated under NIR radiation can not only directly kill the cells but also accelerate the release of DOX and improve the sensitivity and permeability of cells. The DOX/MCN@Si-Cd compound achieved accurate drug delivery, controlled drug release and synergistic chemo-photothermal antitumor therapy (Figures 5B–D) (Lu et al., 2020).
2.4 Carbon nanomaterial-based antitumor immunity
Carbon nanomaterials (carbon nanotubes, carbon quantum dots, graphene oxide, carbon nanohorns (Karousis et al., 2016), etc.) are widely used in medical research due to their ideal biocompatibility, unique photothermal conversion efficiency and other physiochemical and chemical properties (Jiang Y. et al., 2019; Giordani et al., 2019; Wiehe et al., 2019; Liu et al., 2020; Lee et al., 2021; Sainz-Urruela et al., 2021). Graphene quantum dots (GQDs) have been shown to produce singlet oxygen and other ROS under specific light activation, which is the key to the phototoxicity of PDT (Ge et al., 2014). Zhang et al. proposed a hybrid photosensitizer (GQD-PEG) based on the connection of the original GQDs to polyethylene glycol (PEG), which showed significant ROS generation efficiency and excellent biocompatibility under 560 nm laser irradiation (Zhang et al., 2020b). In addition, GQD-PEG showed a strong ablative effect under irradiation and a significant increase in antitumor immune-associated cytotoxic T lymphocytes (CTLs) and proinflammatory cytokines. Liu et al. found that water-soluble C (60)(OH)(20) nanoparticles have effective antitumor activity in vivo and can increase the production of T helper cell type 1 (Th1) cytokines and decrease the production of Th2 cytokines (Liu et al., 2009).
Single-walled carbon nanotubes (SWNTs) are characterized by strong absorbance in the NIR region (Zhou et al., 2009; Lin et al., 2022) and are able to cross cell membranes without causing cytotoxicity (Porter et al., 2007; Tajabadi, 2019). Some carbon-based nanomaterials can mature DCs and then stimulate an immune response, suggesting that they have potential immunoadjuvant properties in cancer immunotherapy (Wang et al., 2014). Zhou et al. designed a multifunctional SWNT system that can absorb NIR light to destroy tumor cells and carry immune stimulants into tumor cells to enhance tumor immunogenicity (Zhou et al., 2012). However, given the degradability of carbon nanomaterials in vivo (Chong et al., 2015), biodegradable carbon nanotubes or graphene oxide (GO) that have been reported thus far tend to have an inhomogeneous size or morphology, which may lead to uncertain side effects in vivo (Bianco, 2013). Thus, Wang et al. designed a degradable carbon-silica nanocomposite (CSN) with immunoadjuvant properties that could be degraded into small particles (∼5 nm) (Figure 6) (Wang et al., 2020). In vivo, the tumor inhibition efficiency of CSN was above 90% in the 4T1 tumor model and the PDX tumor model.
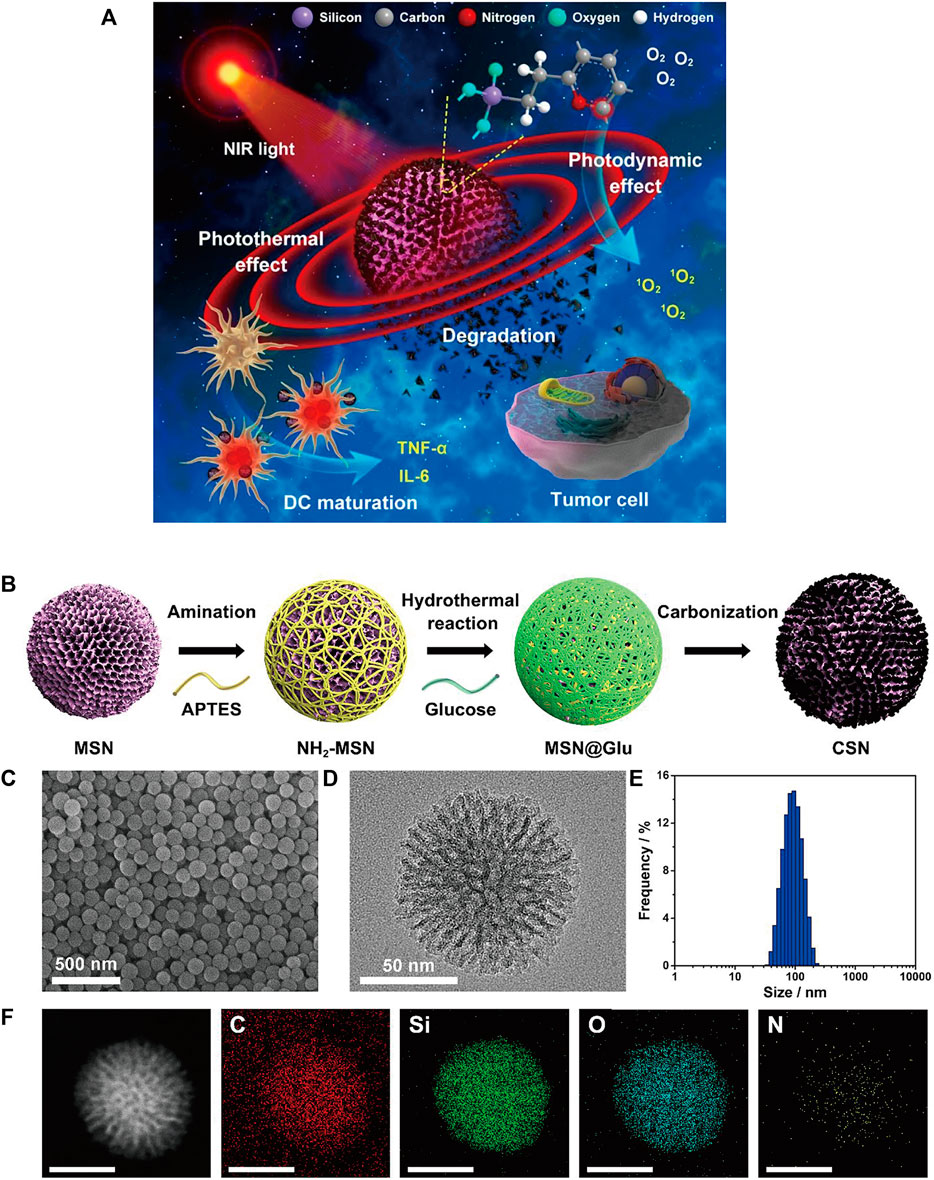
FIGURE 6. (A) Schematic illustration of degradable CSNs with immunoadjuvant properties for photothermal and photodynamic cancer therapy. (B) Schematic illustration of CSN synthesis. (C) SEM and (D) high-resolution transmission electron microscopy (HR-TEM) images of CSN. (E) Size distribution of CSN. (F) High-angle annular dark-field scanning TEM (HAADF-STEM) image and element mapping of CSN. (Reproduced from Wang et al., 2020, ACS Nano).
GO is considered a promising nanomaterial for NIR drug delivery systems due to its two-dimensional film structure, biocompatibility and near infrared absorption spectroscopy (Daniyal et al., 2020). Tao et al. applied a GO-PEG-PEI nanosystem to efficiently deliver CpG, and its NIR light absorbance can control the immune stimulation activity of CpG ODNs (Tao et al., 2014). Under irradiation with NIR light, the intracellular transport of nanocarriers was accelerated due to PTT, and the immune stimulation response was significantly enhanced. Zhou et al. constructed a nanosystem (rGO/MTX/SB) that loaded the chemotherapy agent mitoxantrone (MTX) and transforming growth factor-β (TGF-β) inhibitor SB-431542 (SB) onto reduced graphene oxide (rGO) (Zhou B. et al., 2021). Under noninvasive NIR light irradiation, MTX-induced ICD effectively activated systemic antitumor immune responses, and SB helped to alter the tumor microenvironment to enhance reduced graphene oxide (rGO) (Figure 7). This synergistic therapy induced superior antitumor immunity, tumor killing and immune processes and triggered effective CTL control of metastasis.
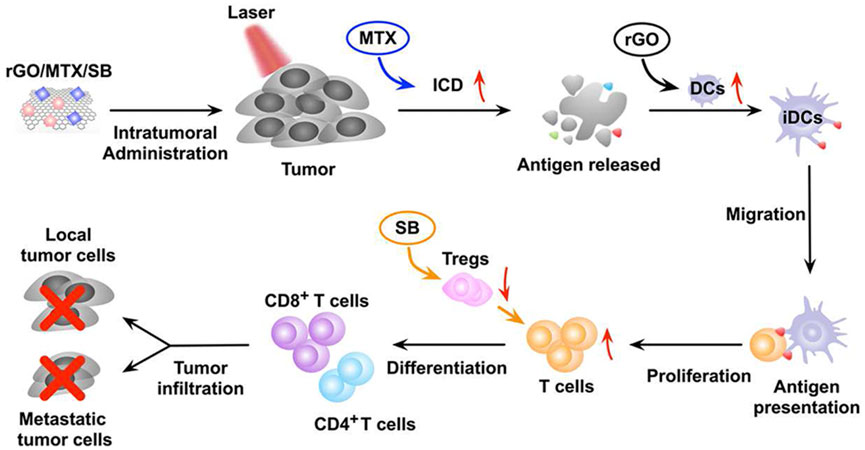
FIGURE 7. The mechanism of the antitumor immune response induced by rGO/MTX/SB-based PTT. (Reproduced from Zhou B. et al., 2021, Biomaterials).
2.5 Metal nanomaterial-based antitumor immunity
Metal nanomaterials have been widely used in biomedical fields due to their good physicochemical properties (Popescu, et al., 2015; Vimbela, et al., 2017). Among them, gold nanomaterials have the advantages of photocontrol ability, chemical inertness and minimal toxicity (Kohout et al., 2018; Zhou F. et al., 2021) and are widely used in the diagnosis and treatment of tumors (Singh et al., 2018; Ding et al., 2020; Guinart et al., 2020; Essawy et al., 2021). Upconversion nanoparticles are also in the category of metal nanoparticles, but they are a relatively special metal rare earth element that can be used for more effective and safer cancer treatment (Li H. et al., 2020; Liu X. et al., 2021). In addition, there are other metal nanomaterials, such as Pt, Cu and Fe, which are used in cancer therapy due to their unique physicochemical properties.
2.5.1 Gold nanomaterial-based antitumor immunity
Gold nanorods (AuNRs) with tunable and strong NIR absorption are considered one of the most promising drugs for tumor therapy and diagnosis (Lee & Gaharwar, 2020). Yata et al. designed a composite immunostimulatory DNA hydrogel, mixing appropriately designed hexapods with CpG-modified gold nanoparticles to form a composite gold nanoparticle-DNA hydrogel (Yata et al., 2017). Under laser irradiation, the hydrogel released hexapods, effectively stimulating immune cells and releasing proinflammatory cytokines. Ahn et al. reported an AuNP-based therapeutic cancer vaccine carrying endogenous EDB autoantigens (Ahn et al., 2014). Gold nanoparticles can effectively deliver antigens to dendritic cells and induce antigen-specific cytotoxic T lymphocyte responses for effective cancer therapy. Khoobchandani et al. designed a novel nanodrug MGF-AuNP formed by encapsulating mangiferin (MGF) with gold nanoparticles, which can provide an effective immunomodulatory intervention by targeting the tumor microenvironment (Khoobchandani et al., 2021).
The tumor microenvironment is an indispensable part of tumors (Pitt et al., 2016) and is one of the key factors affecting immunotherapy effects (Osipov et al., 2019; Xiao & Yu, 2021). Tian et al. designed a multifunctional nanoparticle (HA-AuNR/M-M2pep NP) to overcome the limitations of the tumor microenvironment on immunotherapy efficiency. It is composed of gold nanorods (HA-AuNR) modified with M2pep melt peptide (M-M2pep) in response to hyaluronic acid (HA) and matrix metalloproteinase-2 (MMP2). Precise PTT can be achieved under NIR light irradiation, triggering tumor immunogenic cell death and antitumor immunity irradiation (Figure 8A). Meanwhile, the nanoparticles release M2pep by cleaving MMP2-sensitive peptide, which can improve the immune activity of the TME and further enhance the antitumor efficacy (Tian et al., 2021).
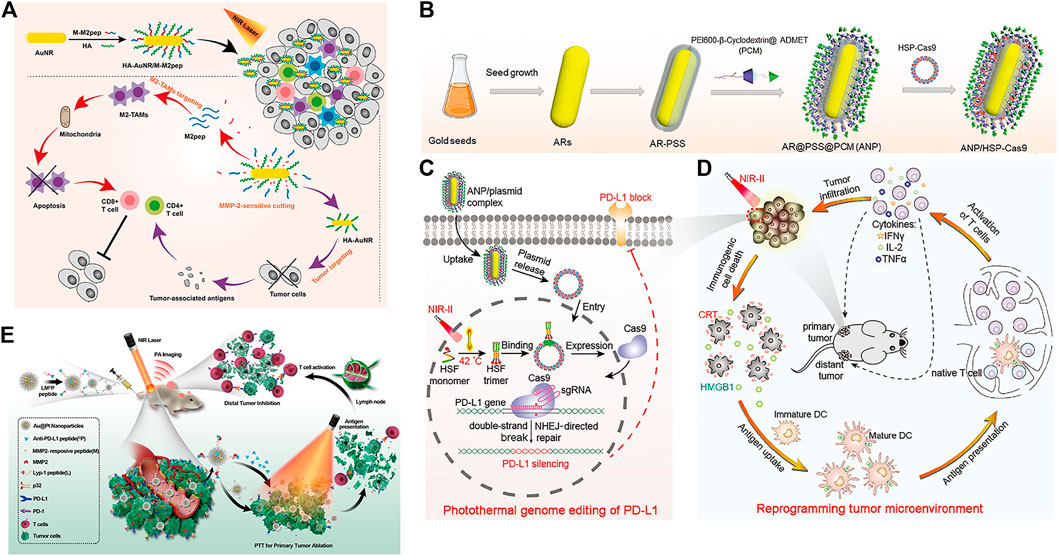
FIGURE 8. (A) Schematic illustration of enhanced photoimmunotherapy by the combined effect of PTT-induced immune activation and M2-TAM depletion. (Reproduced from Tian et al., 2021, Colloids and Surfaces B: Biointerfaces). (B–D) Schematic illustration of the photothermal genome-editing strategy for cancer immunotherapy. (B) Process of preparation of the ANP/HSP-Cas9 plasmid complex. (C) Illustration of photothermal activation for PD-L1 genome editing in tumor cells. (D) Photoactivable CRISPR/Cas9 strategy reprograms the immunosuppressive tumor environment. (Reproduced from Tang et al., 2021, Advance Materials). (E) Schematic illustration of the combination of photothermal and immunotherapy by Au@Pt-LMDP. (Reproduced from Yang et al., 2019, Journal of Controlled release).
Tang et al. used supramolecular gold nanorods to target and block the immune checkpoint (PD-L1-CRISPR/Cas9), which blocked the gene expression of PD-L1 under NIR light irradiation to improve the transformation of dendritic cells into T cells, promote T-cell infiltration and enhance antitumor immunity in the body (Figures 8B–D) (Tang et al., 2021). In addition, the gold nanorods can produce mild hyperthermia to induce immunogenic cell death after NIR light irradiation and further enhance tumor immunotherapy. Yang et al. reported a Au@Pt-LMDP nanosystem conjugated by Au@Pt with a reasonably designed peptide (LYP-1-PLGVRG-DPPA-1, LMDP) (Yang et al., 2019). The system can effectively eliminate primary tumors through PTT and can also act as a tumor-targeting agent activated by MMPs, releasing D-peptide antagonists of PD-L1 and stimulating the activation of cytotoxic T lymphocytes, thereby inhibiting distant tumor growth and reducing tumor metastasis (Figure 8E).
2.5.2 Upconversion nanoparticle-based antitumor immunity
Upconverted nanoparticles (UCNPs) are a class of lanthanide-doped optical nanocrystals that have broad application prospects in light-controlled tumor therapy owing to their low toxicity, good chemical stability, and good photostability (Qiu et al., 2018; Wen et al., 2018). UCNPs can convert near infrared (NIR) light to UV or visible light via the sequential absorption of two or more low-energy photons, together with their deep penetrating ability, making UCNPs hot materials (Zhou et al., 2015; Wang et al., 2018; Zhou et al., 2018; Liu Y. Q. et al., 2021). Xiang et al. designed UCNPs loaded with dendritic cell (DC) vaccine antigen to label and stimulate DCs to achieve precise tracking and induce antigen-specific immune responses in vivo, thereby exerting antitumor immunity (Xiang et al., 2015). Our team has developed a remote-controlled antitumor immunotherapy device based on UCNPs, constructed by combining UCNPs, immunotherapeutic CpG oligonucleotides (ODN), and complementary ssDNA (PcDNA) containing a photocleavable (PC) bond (Chu et al., 2019). Under irradiation with NIR light, UCNPs can convert NIR light into high-energy UV light, which can photolytically break the PC bond and decompose PcDNA into DNA fragments, thereby releasing CpG ODNs to activate and control the body’s immune activity. Ding et al. reported biodegradable K3ZrF7:Yb/Er UCNPs (ZrNPs) as pyroptosis inducers for cancer immunotherapy (Ding et al., 2021). Sensitizer ions (Yb3+) absorb low-energy infrared radiation and effectively transfer excitation energy to activator ions (Er3+, TM3+, or HO3+), which emit high-energy ultraviolet (UV), visible, and NIR light through a multiphoton process. ZrNP-like ion banks dissolve in cancer cells and release large amounts of K+ and [ZrF7]3− ions, further inducing an increase in oxidative pressure and reactive oxygen species (ROS). In addition, the results confirmed that ZrNPs can increase dendritic cell (DC) maturity and effector memory T-cell frequency, thereby inhibiting tumor growth and metastasis in vivo (Figure 9) (Ding et al., 2021).
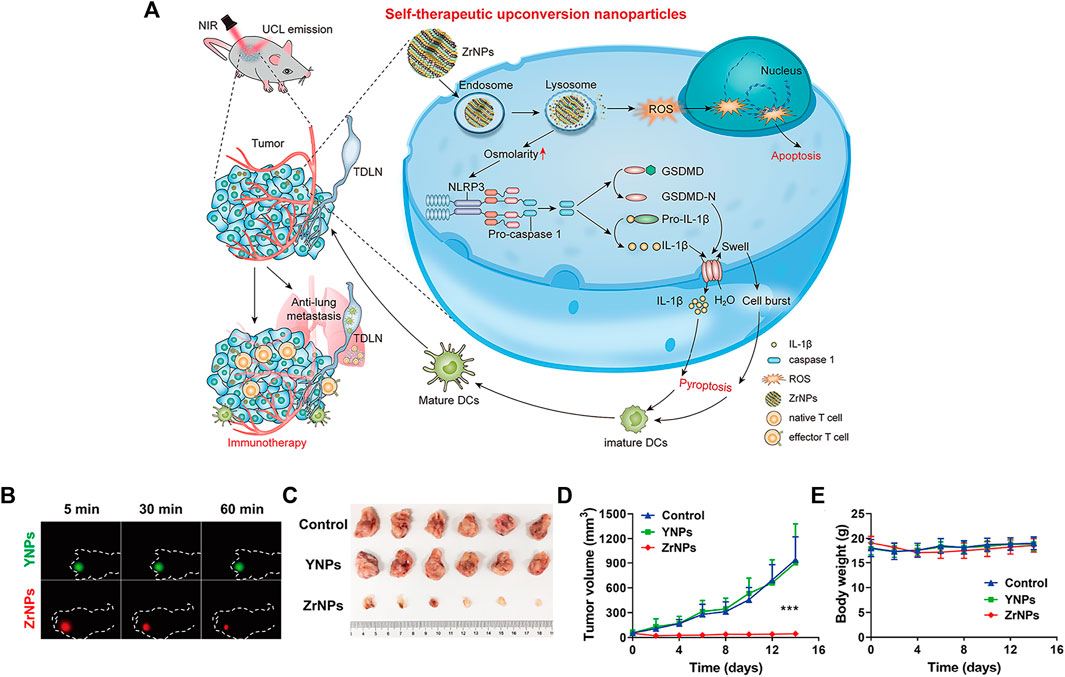
FIGURE 9. Scheme and therapeutic effects in vivo for antitumor therapy. (A) Schematic illustration of K3ZrF7:Yb/Er upconversion nanoparticles (ZrNPs) to induce pyroptosis for cancer immunotherapy. (B) In vivo UCL images of YNPs or ZrNPs at different time points. (C) Digital photographs of excised tumors. (D) Tumor growth and (E) body weight curves. (Reproduced from Ding et al., 2021, Nano Letters).
Mao et al. reported a nanoscale immune stimulator loaded with the aggregation-induced emission (AIE) photosensitizer TPEBTPy on UCNPs (Figure 10A) (Mao et al., 2020). TPEBTPy with AIE characteristics showed strong fluorescence and ROS generation in the aggregation state (Hu et al., 2018). The combination of TPEBTPy and UCNPs can improve light penetration and have a strong interaction. The nanomaterial enhanced the adaptive immune response to solid tumors by modulating ROS production while simultaneously activating tumor immunogenic cell death (ICD) and dendritic cells to prevent local tumor recurrence and metastasis (Figures 10B–E) (Mao et al., 2020).
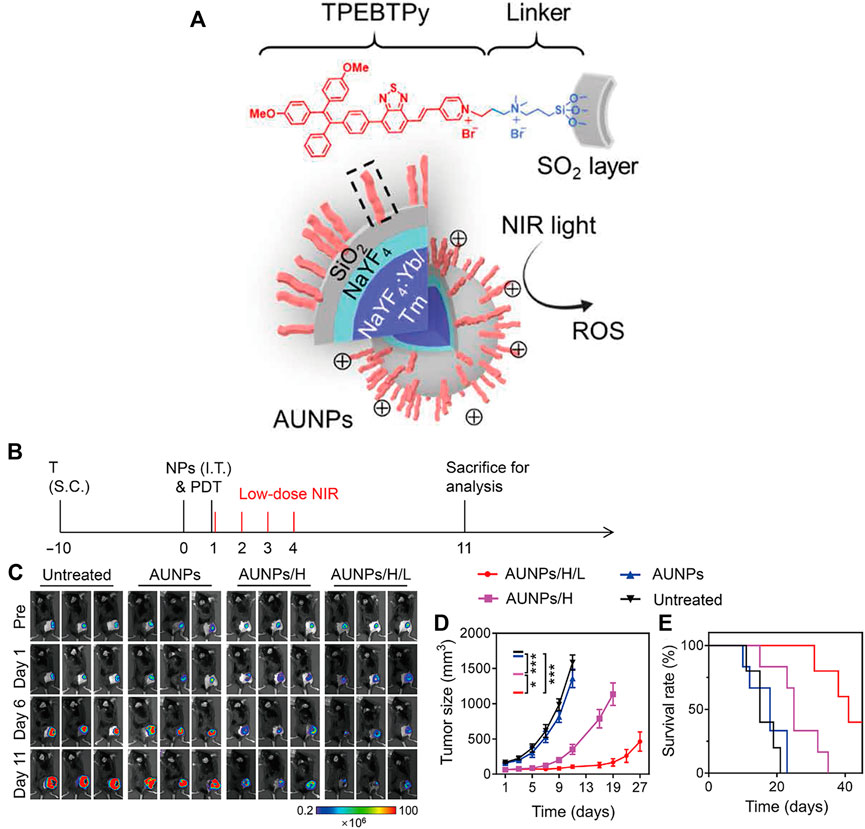
FIGURE 10. Antitumor immunotherapy with AUNP to inhibit B16F10 tumor growth. (A) Structure of a nanoscale immune stimulator. The dashed box indicates a linked TPEBTPy molecule on the AUNPs. (B) Schematic illustration of the treatment schedule. T, tumor inoculation; S.C., subcutaneous injections. (C) Bioluminescence images of the B16F10 tumor-bearing mice receiving different treatments. (D) Tumor growth curve and (E) survival curve of B16F10 tumor-bearing mice in the control and treated groups (n = 5). (Reproduced from Mao et al., 2020, Science Advances).
Chen et al. reported a tumor-associated macrophage membrane (TAMM) derived from primary tumors, which was coated with a conjugated photosensitizer (NPR@TAMM) on UCNPs (Chen Y. et al., 2021). The TAMM has unique antigen-homing affinity and immune compatibility and can consume CSF1 secreted by tumor cells in the tumor microenvironment (TME), thus blocking the interaction between TAMs and cancer cells. NPR@TAMM-mediated photodynamic immunotherapy transformed macrophage activation from an immunosuppressive M2-like phenotype to a more inflammatory M1-like state and induced immunogenic cell death, thus stimulating antitumor immune efficiency by activating antigen-presenting cells (Figure 11).
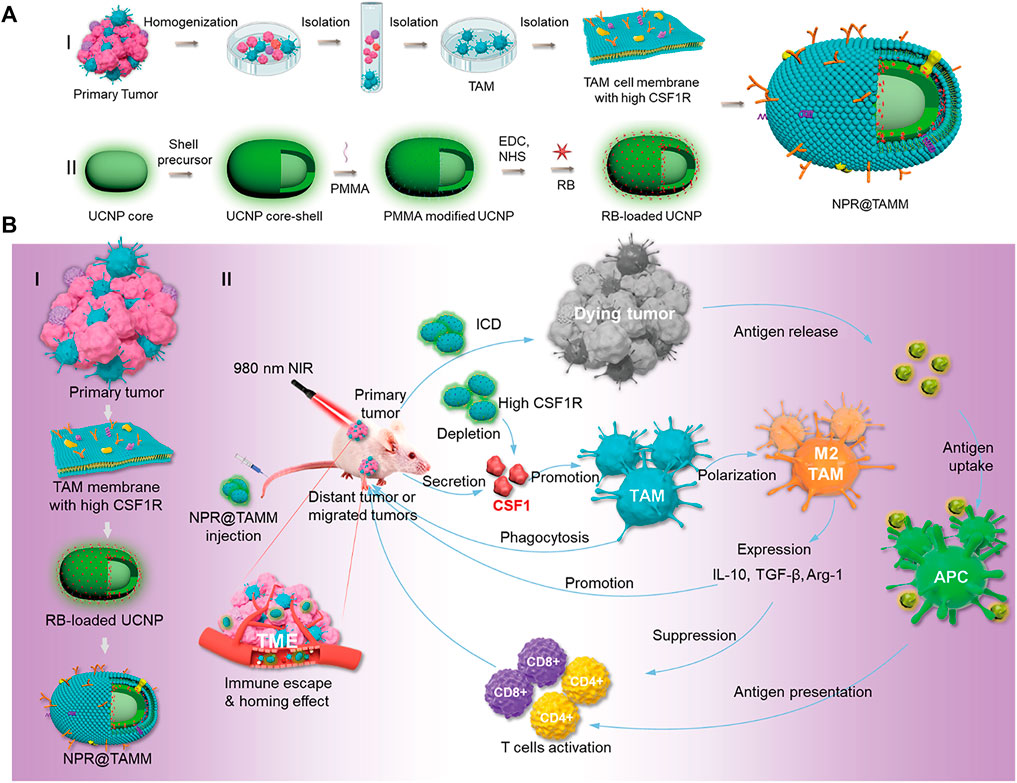
FIGURE 11. Schematic illustration of the design and mechanism of tumor-associated macrophage membrane-coated upconverting nanoparticles. (A) Schematic illustration of the preparation of TAMM-coated NPR@TAMMs. (B) Mechanism illustration of TAMM-coated NPR@TAMMs for photodynamic immunotherapy. (Reproduced from Chen Y. et al., 2021, Nano Letters).
However, the therapeutic effect of single immunotherapy is still poor, and synergistic immunotherapy has a better antitumor immune effect (Sang et al., 2019; Guo et al., 2022). As shown in Figure 12A, photothermal therapy (PTT) can induce deep tissue immunogenic cell death and enhance antitumor immunotherapy (Chen et al., 2001; Chen et al., 2020; Li W. et al., 2020). Similarly, photodynamic therapy can induce immunogenic cell death and activate adaptive immune responses to tumor-associated antigens (Figure 12B) (Castano et al., 2006). Therefore, the application of synergistic immunotherapy based on light-controlled nanomaterials has more potential for clinical application.
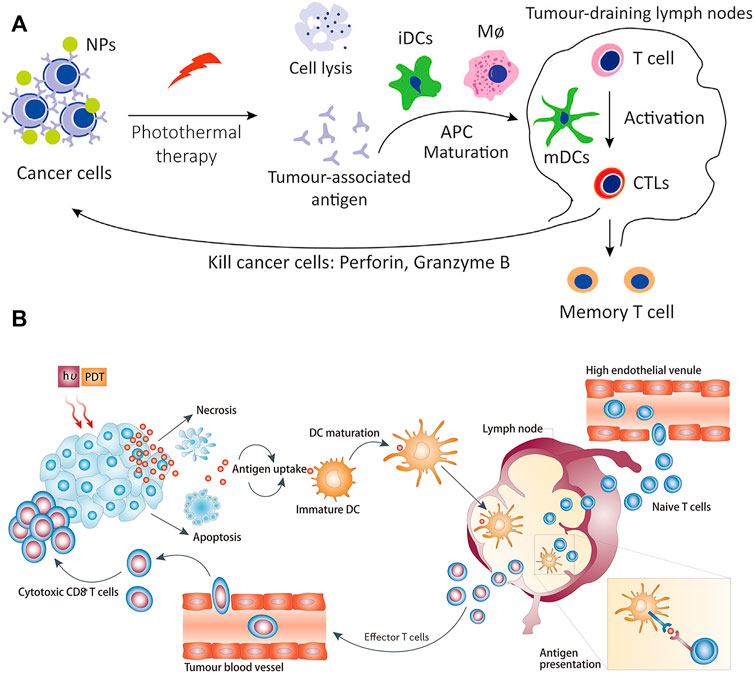
FIGURE 12. Antitumor immunotherapy based on photothermal therapy and photodynamic therapy. (A) Photothermal therapy increases immunogenic cell death and releases antigens that are delivered to T cells, enhancing the recognition and killing of tumor cells. (Reproduced from Li W. et al., 2020, Frontiers in Immunology). (B) Photodynamic therapy induces the activation of antigen-specific T cells. (Reproduced from Castano et al., 2006, Nature Reviews Cancer).
Xu et al. designed a nanoplatform that combined UCNPs triggered by PDT with checkpoint blockade (Xu et al., 2017). The UCNPs were simultaneously loaded with photosensitizer e6 (Ce6) and toll-like receptor 7 agonist imiquimod (R837) to form UCNP-Ce6-R837, which was then combined with cytotoxic T lymphocyte-associated protein (CTLA-4) checkpoint blocker. The release of tumor-associated antigens through PDT under NIR irradiation also enhances antitumor immune responses with long-term immune memory function (Figure 13A). Wang et al. reported an NIR-triggered antigen nanoplatform for synergistic immunotherapy, which is a combination of lipid molecules (DSPE-PEG-mal), light absorber indocyanine green (ICG) and photosensitizer rose bengal (RB) assembled in UCNPs (Wang Z. et al., 2019). Tumor cells irradiated with NIR can release tumor-derived protein antigen (TDPA), triggering immunogenic cell death. In addition, TAPDs can be captured by the platform to induce tumor-specific immune responses (Figure 13B). Ding et al. prepared upconversion nanoparticles (UCMS) coated with mesoporous silica as an immune adjuvant for antitumor immunotherapy (Ding et al., 2018). UCMS was simultaneously loaded with the photosensitizers merocyanine 540 (MC540), chicken OVA or tumor antigens. NIR light irradiation can activate MC540, which produces ROS and releases TAA to stimulate DCs, resulting in T-cell activation and proliferation and the release of cytokines to kill tumor cells (Figure 13C).
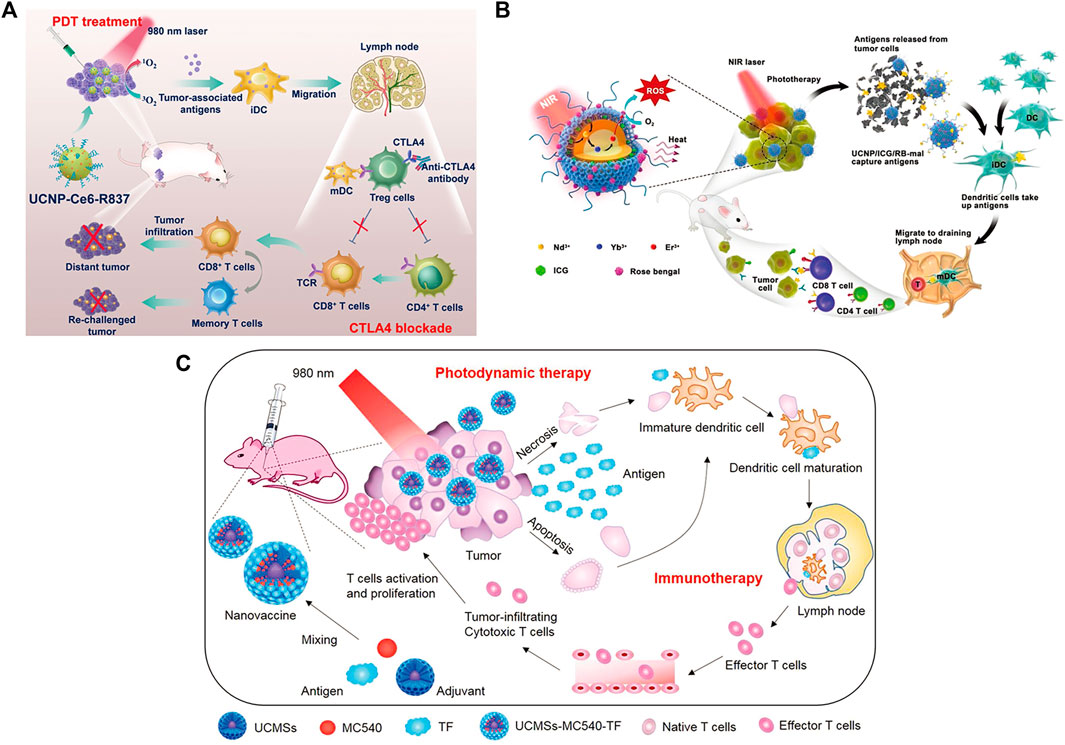
FIGURE 13. (A) Schematic illustration of NIR-triggered PDT with multitasking UCNPs in combination with checkpoint blockade for immunotherapy of cancer. (Reproduced from Xu et al., 2017, ACS Nano). (B) Schematic illustration of both the fabrication and mechanism of a near-infrared (NIR)-triggered antigen-capturing nanoplatform. (Reproduced from Wang S. et al., 2019, Advance Science). (C) Schematic illustration of the fabrication and mechanism of UCMSs-MC540-TF nanovaccines for PDT and immunotherapy. (Reproduced from Ding et al., 2018, Advance Materials).
2.5.3 Other metal nanoparticle-based antitumor immunity
Zero-valent iron (ZVI) nanoparticles (NPs) have a strong reduction potential (Zou et al., 2016) and can produce a large number of reactive oxygen species (ROS) (Yang L. X. et al., 2020; He et al., 2022). Hsieh et al. utilized ZVI-NP to enhance phosphorylation-dependent ubiquitination and degradation of nuclear factor E2-related factor 2 (NRF2), resulting in excessive oxidative stress and lipid peroxidation (Hsieh et al., 2021). Furthermore, ZVI-NPs reprogrammed the polarization of tumor-associated macrophages into an antitumor M1 phenotype, increased the cytotoxic function of CD8+ T cells and decreased the proportion of regulatory T cells to enhance antitumor immunity. Cobalt oxide nanoparticles (CoO NPs) are promising tools for delivering antigens to antigen-presenting cells and have induced antitumor immune responses. Chattopadhyay et al. found that CoO NPs modified with N-phosphonylmethyliminodiacetic acid (PMIDA) bound lysate-promoting antigens, which are cancer antigens derived from cancer cell lysis, to form cancer cell lysate antigen-conjugated PMIDA-CoO NPs (Ag-PMIDA-CoO NPs) (Chattopadhyay et al., 2016). The nanoparticles can activate macrophages (M φ) to improve the anticancer immune response, increase serum IFN-γ and TNF-α levels and act as an adjuvant to balance proinflammatory and anti-inflammatory immune responses.
Platinum nanoparticles (Pt NPs) are selectively toxic to cancer cells (Xia et al., 2016; Shoshan et al., 2019) and enable photothermal conversion through NIR irradiation (Yang et al., 2015), leading to targeted hyperthermia (Zhou et al., 2016) and antigen release (Ma et al., 2019). Yu et al. constructed Pt NPs that conjugated PD-L1 inhibitor (BMS-1) to Mal-modified polyethylene glycol (PEG) via thermosensitive bonds (Yu et al., 2021). Under NIR irradiation, Pt NPs ablated tumors by PTT and released BMS-1 to alleviate T-cell depletion and induce effector T cells to infiltrate into tumor tissues and acted as immune adjuvants to stimulate the maturation of DCs. Mal exposed to the surface of nanoparticles captured the antigens released by tumor cells and enhanced antigen internalization and presentation (Figure 14).
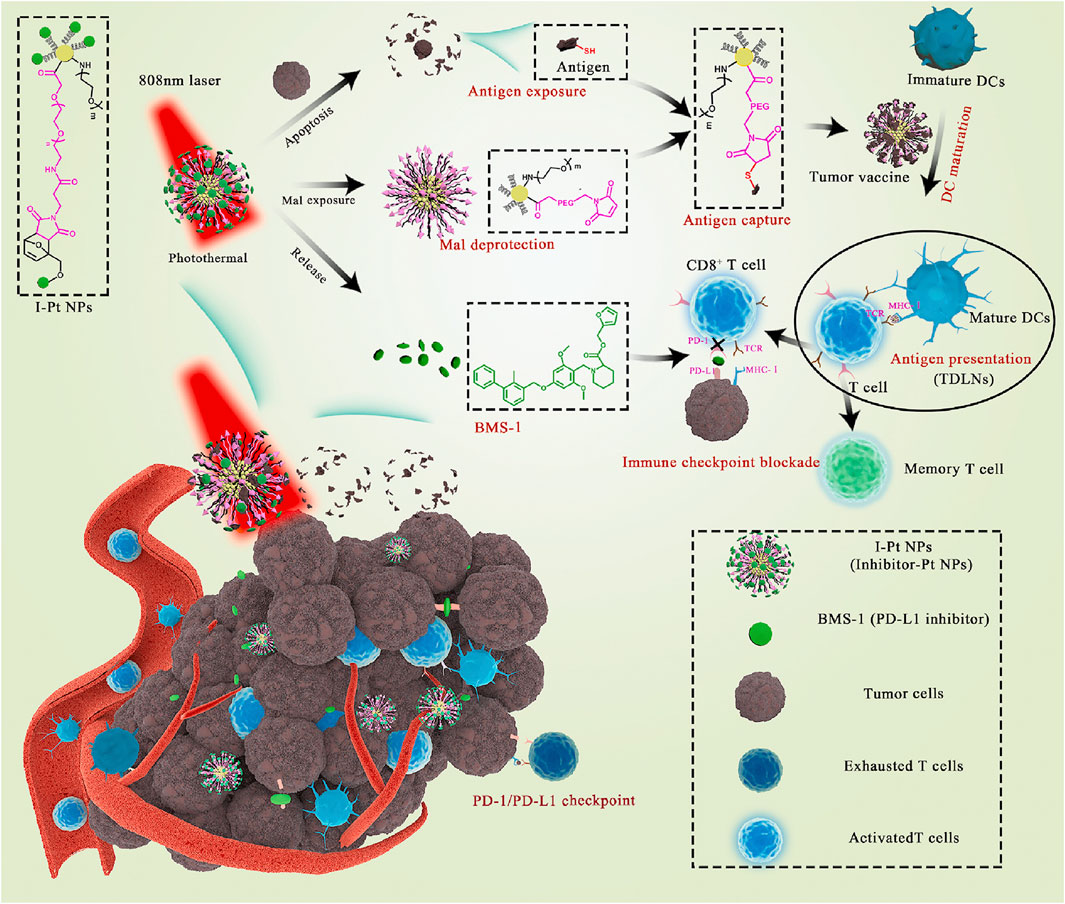
FIGURE 14. Schematic illustration of Pt NPs conjugated with BMS-1 for NIR-controlled release of inhibitor and exposure to Mal. (Reproduced from Yu et al., 2021, Bioactive Materials).
Hollow copper sulfide nanoparticles (HCuSNPs) are biodegradable photothermal coupling agents that can be excreted from the liver and kidney with low toxicity (Guo et al., 2013). Guo et al. reported a CuS-based transformational nano-CPG system (HCuSNPs-CpG) induced by NIR light (Guo et al., 2014). Upon NIR light irradiation, HCuSNPs-CpG structures were decomposed, reassembled and transformed into chitosan-CPG nanocomplexes, which increased the stability, tumor retention, and internalization of CpG by plasmacytoid dendritic cells and initiated effective systemic antitumor immunity by activating Toll-like receptor 9 signaling (Figure 15).
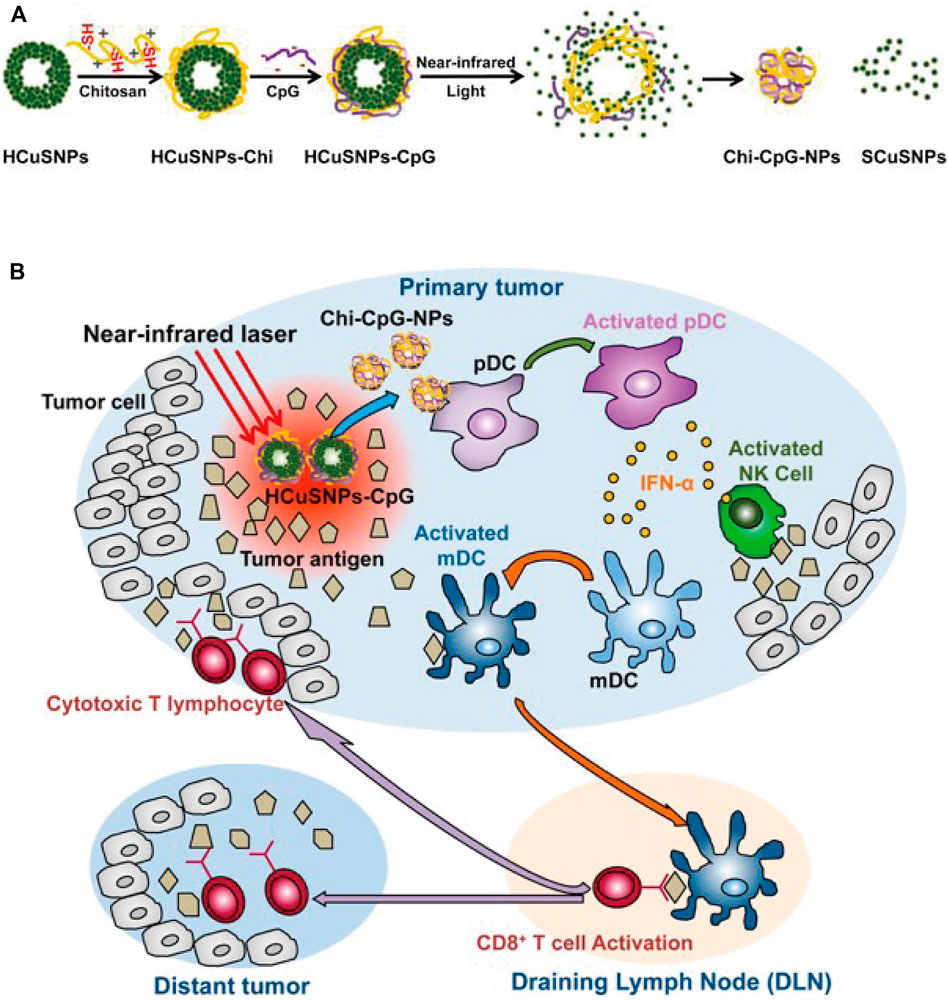
FIGURE 15. Schematic illustration of the preparation of HCuSNPs-CpG for photothermal immunotherapy. (A) Schematic illustration of the assembly and decomposition of the HCuSNPs-CpG conjugate. “HCuSNPs-Chi” represents chitosan-coated HCuSNPs. “Chi-CpG-NPs” represents chitosan-CpG nanocomplexes. “SCuSNPs” represents small CuS nanoparticles. (B) Schematic illustration of HCuSNPs-CpG-mediated photothermal immunotherapy of both primary treated and distant untreated tumors. (Reproduced from Guo et al., 2014, ACS Nano).
Titanium nanosheets (Ti NSs), as novel and economical two-dimensional nanomaterials, have strong NIR light absorption ability, high photothermal conversion efficiency and good biosafety (Xie et al., 2019; Yuan et al., 2022). However, Ti NSs are prone to oxidation in vivo, and their application in medical materials is also limited. Moreover, polyethylene glycol (PEG) can improve Ti NS stability, increase the retention time of NSs in blood circulation, and enhance the drug delivery capacity of the tumor site (Yang Y. et al., 2020).
In addition, previous studies showed that some transition-metal ions (including Fe3+, Cu2+ and Mn2+) can be bound to the PDA structure by coordination (Li et al., 2016; Wang Z. X. et al., 2017; Ge et al., 2017). Xu et al. prepared Fe (III) chelated PDA nanoparticles with high loading and response to release iron ions, which can improve the light absorption behavior of PDA in the NIR spectrum and endow PDA with better photothermal conversion ability (Xu et al., 2022). The in vivo and in vitro results showed that Fe-PDA could significantly inhibit tumor growth and effectively promote the repolarization of tumor-associated macrophages to the M1 mode compared with PDA. Fe-PDA combined with PTT effectively improved the efficacy of immunotherapy.
A new class of nanophotosensitizers (nPSs) based on nanoscale metal-organic frameworks (nMOFs) have attracted extensive attention in the application of PDT (Shao et al., 2020; Song et al., 2021). Lan et al. reported a novel nanophotosensitizer nanoscale metal-organic framework Fe-TBP, which can overcome tumor hypoxia and enhance the sensitivity of effective PDT, thereby initiating noninflammatory tumors for cancer immunotherapy (Lan et al., 2018). When Fe-TBP is irradiated under anoxic conditions, it can catalyze a cascade reaction to produce O2 through a Fenton-like reaction, and O2 is further converted to singlet oxygen with cytotoxicity by photoexcited porphyrins (O2) to produce PDT effects. In addition, the PDT-induced systemic antitumor response ameliorates α-PD-L1 ICB, leading to the regression of primary and distant tumors through a distant effect.
3 Future and prospects
In summary, this review discusses recent advances in light-activated nanomaterials and their applications in antitumor immunotherapy. With the progress of nanotechnology, the application of nanomaterials in antitumor immunotherapy cannot be ignored. The clinical efficiency of laser treatments is limited by the low penetration of UV, visible light or visible light and makes light-activated imaging or therapy in a dilemma. To achieve deeper tissue penetration ability, near infrared (NIR) light with low energy and long wavelength is a good choice. NIR frequency bands present an optical window for deeper penetration into biological tissue. Materials such as upconversion nanoparticles have the unique capability to efficiently convert NIR light irradiation into UV or visible light via the sequential absorption of two or more low-energy photons. This approach achieves the same goal as UV or visible light with deeper tissue penetration. Despite the described promise, there still exist challenges based on light-activated nanomaterials that need to be overcome to meet the demand in clinics. First, the toxicity of light-activated nanomaterials which is also a general concern for all nanomaterials. To date, research on light-activated nanomaterials has mainly focused on constructing new light-activated activation strategies, and the metabolism and toxicity of materials are not deeply understood. Moreover, most of the models used for nanomaterial exploration are restricted to small animals, and few studies have used large animals. Furthermore, the synthesis standards of nanomaterials, the loading content of drugs, poor solubility in the physiological environment, how to effectively preserve them, etc., as well as industry consensus, are also obstacles to the clinical application of nanomaterials. Since the mechanism of tumorigenesis varies from person to person, a single immunotherapy may not achieve satisfactory antitumor therapeutic effects, and synergistic immunotherapy is becoming an important method of antitumor therapy. It is also a great challenge to combine different therapeutic mechanisms and different materials in the same nanosystem in a rational, compatible and synergistic way to achieve efficient synergistic immunotherapy. In addition, the potential risks of photoactivated nanomaterials in clinical applications, such as systemic toxicity, complexity of clearance, and long-term effects on the human body, must also be considered. The above issues may activate future exploration in the development and improvement of light-activated nanomaterials, providing better opportunities for antitumor immunotherapy for future patients.
Author contributions
FW and HD wrote the manuscript after discussion with; WX and GS: conceptualization, supervision, validation; ZS and HC supervision, writing—review and editing. All authors gave final approval for publication and agreed to be held accountable for the work performed therein.
Funding
This work was supported by the Natural Science Foundation of China (No. 82001946) and Beijing Municipal Natural Science Foundation (No. 7214300).
Conflict of interest
The authors declare that the research was conducted in the absence of any commercial or financial relationships that could be construed as a potential conflict of interest.
Publisher’s note
All claims expressed in this article are solely those of the authors and do not necessarily represent those of their affiliated organizations, or those of the publisher, the editors and the reviewers. Any product that may be evaluated in this article, or claim that may be made by its manufacturer, is not guaranteed or endorsed by the publisher.
References
Abbott, M., and Ustoyev, Y. (2019). Cancer and the immune system: The history and background of immunotherapy. Semin. Oncol. Nurs. 35, 150923. doi:10.1016/j.soncn.2019.08.002
Ahn, S., Lee, I. H., Kang, S., Kim, D., Choi, M., Saw, P. E., et al. (2014). Gold nanoparticles displaying tumor-associated self-antigens as a potential vaccine for cancer immunotherapy. Adv. Healthc. Mat. 3, 1194–1199. doi:10.1002/adhm.201300597
Akinleye, A., and Rasool, Z. (2019). Immune checkpoint inhibitors of PD-L1 as cancer therapeutics. J. Hematol. Oncol. 12, 92. doi:10.1186/s13045-019-0779-5
Barkat, A., Beg, S., Panda, S. K., S Alharbi, K., Rahman, M., and Ahmed, F. J. (2021). Functionalized mesoporous silica nanoparticles in anticancer therapeutics. Semin. Cancer Biol. 69, 365–375. doi:10.1016/j.semcancer.2019.08.022
Berraondo, P., Sanmamed, M. F., Ochoa, M. C., Etxeberria, I., Aznar, M. A., Pérez-Gracia, J. L., et al. (2019). Cytokines in clinical cancer immunotherapy. Br. J. Cancer 120, 6–15. doi:10.1038/s41416-018-0328-y
Bertucci, A., Prasetyanto, E. A., Septiadi, D., Manicardi, A., Brognara, E., Gambari, R., et al. (2015). Combined delivery of temozolomide and anti-miR221 PNA using mesoporous silica nanoparticles induces apoptosis in resistant glioma cells. Small 11, 5687–5695. doi:10.1002/smll.201500540
Bharti, C., Nagaich, U., Pal, A. K., and Gulati, N. (2015). Mesoporous silica nanoparticles in target drug delivery system: A review. Int. J. Pharm. Investig. 5, 124–133. doi:10.4103/2230-973X.160844
Bianco, A. (2013). Graphene: Safe or toxic? The two faces of the medal. Angew. Chem. Int. Ed. 52, 4986–4997. doi:10.1002/anie.201209099
Burugu, S., Dancsok, A. R., and Nielsen, T. O. (2017). Emerging targets in cancer immunotherapy. Semin. Cancer Biol. 52, 39–52. doi:10.1016/j.semcancer.2017.10.001
Castano, A. P., Mroz, P., and Hamblin, M. R. (2006). Photodynamic therapy and anti-tumour immunity. Nat. Rev. Cancer 6, 535–545. doi:10.1038/nrc1894
Chattopadhyay, S., Dash, S. K., Mandal, D., Das, B., Tripathy, S., Dey, A., et al. (2016). Metal based nanoparticles as cancer antigen delivery vehicles for macrophage based antitumor vaccine. Vaccine 34, 957–967. doi:10.1016/j.vaccine.2015.12.053
Chauhan, A., Khan, T., and Omri, A. (2021). Design and encapsulation of immunomodulators onto gold nanoparticles in cancer immunotherapy. Int. J. Mol. Sci. 22, 8037. doi:10.3390/ijms22158037
Cheetham, A. G., Chakroun, R. W., Ma, W., and Cui, H. (2017). Self-assembling prodrugs. Chem. Soc. Rev. 46 (21), 6638–6663. doi:10.1039/c7cs00521k
Chen, C., Song, M., Du, Y., Yu, Y., Li, C., Han, Y., et al. (2021a). Tumor-associated-macrophage-membrane-coated nanoparticles for improved photodynamic immunotherapy. Nano Lett. 21, 5522–5531. doi:10.1021/acs.nanolett.1c00818
Chen, J., Zeng, Z., Huang, L., Luo, S., Dong, J., Zhou, F. H., et al. (2020). Photothermal therapy technology of metastatic colorectal cancer. Am. J. Transl. Res. 12, 3089–3115.
Chen, Q., Xu, L., Liang, C., Wang, C., Peng, R., and Liu, Z. (2016). Photothermal therapy with immune-adjuvant nanoparticles together with checkpoint blockade for effective cancer immunotherapy. Nat. Commun. 7, 13193. doi:10.1038/ncomms13193
Chen, W. R., Singhal, A. K., Liu, H., and Nordquist, R. E. (2001). Antitumor immunity induced by laser immunotherapy and its adoptive transfer. Cancer Res. 61, 459–461.
Chen, Y., Sun, B., Jiang, X., Yuan, Z., Chen, S., Sun, P., et al. (2021b). Double-acceptor conjugated polymers for NIR-II fluorescence imaging and NIR-II photothermal therapy applications. J. Mat. Chem. B 9, 1002–1008. doi:10.1039/d0tb02499f
Cheng, R., Feng, F., Meng, F., Deng, C., Feijen, J., and Zhong, Z. (2011). Glutathione-responsive nanovehicles as a promising platform for targeted intracellular drug and gene delivery. J. Control. Release 152, 2–12. doi:10.1016/j.jconrel.2011.01.030
Cheng, Z., Li, M., Dey, R., and Chen, Y. (2021). Nanomaterials for cancer therapy: Current progress and perspectives. J. Hematol. Oncol. 14, 85. doi:10.1186/s13045-021-01096-0
Choi, H. S., and Frangioni, J. V. (2010). Nanoparticles for biomedical imaging: Fundamentals of clinical translation. Mol. Imaging 9, 291–310. doi:10.2310/7290.2010.00031
Chong, Y., Ge, C., Yang, Z., Garate, J. A., Gu, Z., Weber, J. K., et al. (2015). Reduced cytotoxicity of graphene nanosheets mediated by blood-protein coating. ACS Nano 9, 5713–5724. doi:10.1021/nn5066606
Chu, H., Cao, T., Dai, G., Liu, B., Duan, H., Kong, C., et al. (2021). Recent advances in functionalized upconversion nanoparticles for light-activated tumor therapy. RSC Adv. 11, 35472–35488. doi:10.1039/d1ra05638g
Chu, H., Zhao, J., Mi, Y., Di, Z., and Li, L. (2019). NIR-light-mediated spatially selective triggering of antitumor immunity via upconversion nanoparticle-based immunodevices. Nat. Commun. 10, 2839. doi:10.1038/s41467-019-10847-0
Clawson, C., Huang, C. T., Futalan, D., Seible, D. M., Saenz, R., Larsson, M., et al. (2010). Delivery of a peptide via poly(D, L-lactic-co-glycolic) acid nanoparticles enhances its dendritic cell-stimulatory capacity. Nanomedicine 6, 651–661. doi:10.1016/j.nano.2010.03.001
Cui, D., Li, J., Zhao, X., Pu, K., and Zhang, R. (2020). Semiconducting polymer nanoreporters for near-infrared chemiluminescence imaging of immunoactivation. Adv. Mat. 32, e1906314. doi:10.1002/adma.201906314
Cui, Y., Dong, H., Cai, X., Wang, D., and Li, Y. (2012). Mesoporous silica nanoparticles capped with disulfide-linked PEG gatekeepers for glutathione-mediated controlled release. ACS Appl. Mat. Interfaces 4, 3177–3183. doi:10.1021/am3005225
Cukier, P., Santini, F. C., Scaranti, M., and Hoff, A. O. (2017). Endocrine side effects of cancer immunotherapy. Endocr. Relat. Cancer 24, T331–T347. doi:10.1530/ERC-17-0358
Danhier, F., Ansorena, E., Silva, J. M., Coco, R., Le Breton, A., and Préat, V. (2012). PLGA-Based nanoparticles: An overview of biomedical applications. J. Control. Release 161, 505–522. doi:10.1016/j.jconrel.2012.01.043
Daniyal, M., Liu, B., and Wang, W. (2020). Comprehensive review on graphene oxide for use in drug delivery system. Curr. Med. Chem. 27, 3665–3685. doi:10.2174/13816128256661902011296290
DeMaria, P. J., and Bilusic, M. (2019). Cancer vaccines. Hematol. Oncol. Clin. North Am. 33, 199–214. doi:10.1016/j.hoc.2018.12.001
Ding, B., Shao, S., Yu, C., Teng, B., Wang, M., Cheng, Z., et al. (2018). Large-pore mesoporous-silica-coated upconversion nanoparticles as multifunctional immunoadjuvants with ultrahigh photosensitizer and antigen loading efficiency for improved cancer photodynamic immunotherapy. Adv. Mat. 30, e1802479. doi:10.1002/adma.201802479
Ding, B., Sheng, J., Zheng, P., Li, C., Li, D., Cheng, Z., et al. (2021). Biodegradable upconversion nanoparticles induce pyroptosis for cancer immunotherapy. Nano Lett. 21, 8281–8289. doi:10.1021/acs.nanolett.1c02790
Ding, Y., Sun, Z., Tong, Z., Zhang, S., Min, J., Xu, Q., et al. (2020). Tumor microenvironment-responsive multifunctional peptide coated ultrasmall gold nanoparticles and their application in cancer radiotherapy. Theranostics 10, 5195–5208. doi:10.7150/thno.45017
Duan, Q. Q., Ma, Y., Che, M. X., Zhang, B. Y., Zhang, Y. X., Li, Y., et al. (2019). Fluorescent carbon dots as carriers for intracellular doxorubicin delivery and track. Drug. Deliv. Sci. Technol. 49, 527–533. doi:10.1016/j.jddst.2018.12.015
Duong, H. T. T., Yin, Y., Thambi, T., Nguyen, T. L., Giang Phan, V. H., Lee, M. S., et al. (2018). Smart vaccine delivery based on microneedle arrays decorated with ultra-pH-responsive copolymers for cancer immunotherapy. Biomaterials 185, 13–24. doi:10.1016/j.biomaterials.2018.09.008
Emens, L. A., Ascierto, P. A., Darcy, P. K., Demaria, S., Eggermont, A. M. M., Redmond, W. L., et al. (2017). Cancer immunotherapy: Opportunities and challenges in the rapidly evolving clinical landscape. Eur. J. Cancer 81, 116–129. doi:10.1016/j.ejca.2017.01.035
Essawy, M. M., El-Sheikh, S. M., Raslan, H. S., Ramadan, H. S., Kang, B., Talaat, I. M., et al. (2021). Function of gold nanoparticles in oral cancer beyond drug delivery: Implications in cell apoptosis. Oral Dis. 27, 251–265. doi:10.1111/odi.13551
Fry, T. J., Shah, N. N., Orentas, R. J., Stetler-Stevenson, M., Yuan, C. M., Ramakrishna, S., et al. (2018). CD22-targeted CAR T cells induce remission in B-ALL that is naive or resistant to CD19-targeted CAR immunotherapy. Nat. Med. 24, 20–28. doi:10.1038/nm.4441
Fu, X., Huang, Y., Zhao, H., Zhang, E., Shen, Q., Di, Y., et al. (2021). Near-infrared-light remote-controlled activation of cancer immunotherapy using photothermal conjugated polymer nanoparticles. Adv. Mat. 33, e2102570. doi:10.1002/adma.202102570
Ge, J., Lan, M., Zhou, B., Liu, W., Guo, L., Wang, H., et al. (2014). A graphene quantum dot photodynamic therapy agent with high singlet oxygen generation. Nat. Commun. 5, 4596. doi:10.1038/ncomms5596
Ge, R., Lin, M., Li, X., Liu, S., Wang, W., Li, S., et al. (2017). Cu2+-loaded polydopamine nanoparticles for magnetic resonance imaging-guided pH- and near-infrared-light-stimulated thermochemotherapy. ACS Appl. Mat. Interfaces 13, 19706–19716. doi:10.1021/acsami.7b05583
Ghaferi, M., Koohi Moftakhari Esfahani, M., Raza, A., Al Harthi, S., Ebrahimi Shahmabadi, H., and Alavi, S. E. (2021). Mesoporous silica nanoparticles: Synthesis methods and their therapeutic use-recent advances. J. Drug Target. 29, 131–154. doi:10.1080/1061186X.2020.1812614
Giordani, S., Camisasca, A., and Maffeis, V. (2019). Carbon nano-onions: A valuable class of carbon nanomaterials in biomedicine. Curr. Med. Chem. 26, 6915–6929. doi:10.2174/0929867326666181126113957
Gonçalves, M., Mignani, S., Rodrigues, J., and Tomás, H. (2020). A glance over doxorubicin based-nanotherapeutics: From proof-of-concept studies to solutions in the market. J. Control. Release 317, 347–374. doi:10.1016/j.jconrel.2019.11.016
Goodman, A., Patel, S. P., and Kurzrock, R. (2017). PD-1-PD-L1 immune-checkpoint blockade in B-cell lymphomas. Nat. Rev. Clin. Oncol. 14, 203–220. doi:10.1038/nrclinonc.2016.168
Guinart, A., Perry, H. L., Wilton-Ely, J. D. E. T., and Tetley, T. D. (2020). Gold nanomaterials in the management of lung cancer. Emerg. Top. Life Sci. 4, 627–643. doi:10.1042/ETLS20200332
Guo, B., Sheng, Z., Hu, D., Li, A., Xu, S., Manghnani, P. N., et al. (2017). Molecular engineering of conjugated polymers for biocompatible organic nanoparticles with highly efficient photoacoustic and photothermal performance in cancer theranostics. ACS Nano 11, 10124–10134. doi:10.1021/acsnano.7b04685
Guo, L., Panderi, I., Yan, D. D., Szulak, K., Li, Y., Chen, Y. T., et al. (2013). A comparative study of hollow copper sulfide nanoparticles and hollow gold nanospheres on degradability and toxicity. ACS Nano 7, 8780–8793. doi:10.1021/nn403202w
Guo, L., Yan, D. D., Yang, D., Li, Y., Wang, X., Zalewski, O., et al. (2014). Combinatorial photothermal and immuno cancer therapy using chitosan-coated hollow copper sulfide nanoparticles. ACS Nano 8, 5670–5681. doi:10.1021/nn5002112
Guo, R., Wang, S., Zhao, L., Zong, Q., Li, T., Ling, G., et al. (2022). Engineered nanomaterials for synergistic photoimmunotherapy. Biomaterials 282, 121425. doi:10.1016/j.biomaterials.2022.121425
Havel, J. J., Chowell, D., and Chan, T. A. (2019). The evolving landscape of biomarkers for checkpoint inhibitor immunotherapy. Nat. Rev. Cancer 19, 133–150. doi:10.1038/s41568-019-0116-x
He, M. F., Li, W. Q., Xie, Z. H., Yang, S. R., He, C. S., Xiong, Z. K., et al. (2022). Peracetic acid activation by mechanochemically sulfidated zero valent iron for micropollutants degradation: Enhancement mechanism and strategy for extending applicability. Water Res. 222, 118887. doi:10.1016/j.watres.2022.118887
He, X., and Xu, C. (2020). Immune checkpoint signaling and cancer immunotherapy. Cell Res. 30, 660–669. doi:10.1038/s41422-020-0343-4
Hojman, P., Gehl, J., Christensen, J. F., and Pedersen, B. K. (2018). Molecular mechanisms linking exercise to cancer prevention and treatment. Cell Metab. 27, 10–21. doi:10.1016/j.cmet.2017.09.015
Hsieh, C. H., Hsieh, H. C., Shih, F. S., Wang, P. W., Yang, L. X., Shieh, D. B., et al. (2021). An innovative NRF2 nanomodulator induces lung cancer ferroptosis and elicits an immunostimulatory tumor microenvironment. Theranostics 11, 7072–7091. doi:10.7150/thno.57803
Hu, C., Liu, Y., Chen, J., He, Q., and Gao, H. (2016). A simple one-step synthesis of melanin-originated redshift emissive carbonaceous dots for bioimaging. J. Colloid. Interface Sci. 480, 85–90. doi:10.1016/j.jcis.2016.07.007
Hu, F., Xu, S., and Liu, B. (2018). Photosensitizers with aggregation-induced emission: Materials and biomedical applications. Adv. Mat. 30, e1801350. doi:10.1002/adma.201801350
Hu, Z., Fang, C., Li, B., Zhang, Z., Cao, C., Cai, M., et al. (2020a). First-in-human liver-tumour surgery guided by multispectral fluorescence imaging in the visible and near-infrared-I/II windows. Nat. Biomed. Eng. 4, 259–271. doi:10.1038/s41551-019-0494-0
Hu, Z., Chen, W. H., Tian, J., and Cheng, Z. (2020b). NIRF nanoprobes for cancer molecular imaging: Approaching clinic. Trends Mol. Med. 26, 469–482. doi:10.1016/j.molmed.2020.02.003
Igarashi, Y., and Sasada, T. (2020). Cancer vaccines: Toward the next breakthrough in cancer immunotherapy. J. Immunol. Res. 2020, 5825401–5825413. doi:10.1155/2020/5825401
Irvine, D. J., and Dane, E. L. (2020). Enhancing cancer immunotherapy with nanomedicine. Nat. Rev. Immunol. 20, 321–334. doi:10.1038/s41577-019-0269-6
Jain, R. K., and Stylianopoulos, T. (2010). Delivering nanomedicine to solid tumors. Nat. Rev. Clin. Oncol. 7, 653–664. doi:10.1038/nrclinonc.2010.139
Jia, C., Yang, T., Liu, Y., Zhu, A., Yin, F., Wang, Y., et al. (2018). A novel human papillomavirus 16 L1 pentamer-loaded hybrid particles vaccine system: Influence of size on immune responses. ACS Appl. Mat. Interfaces 10, 35745–35759. doi:10.1021/acsami.8b11556
Jiang, B. P., Zhou, B., Lin, Z., Liang, H., and Shen, X. C. (2019a). Recent advances in carbon nanomaterials for cancer phototherapy. Chemistry 25, 3993–4004. doi:10.1002/chem.201804383
Jiang, C., Cheng, H., Yuan, A., Tang, X., Wu, J., and Hu, Y. (2015). Hydrophobic IR780 encapsulated in biodegradable human serum albumin nanoparticles for photothermal and photodynamic therapy. Acta Biomater. 14, 61–69. doi:10.1016/j.actbio.2014.11.041
Jiang, Q., Wang, K., Zhang, X., Ouyang, B., Liu, H., Pang, Z., et al. (2020). Platelet membrane-camouflaged magnetic nanoparticles for ferroptosis-enhanced cancer immunotherapy. Small 16, e2001704. doi:10.1002/smll.202001704
Jiang, Y., Cui, D., Fang, Y., Zhen, X., Upputuri, P. K., Pramanik, M., et al. (2017). Amphiphilic semiconducting polymer as multifunctional nanocarrier for fluorescence/photoacoustic imaging guided chemo-photothermal therapy. Biomaterials 145, 168–177. doi:10.1016/j.biomaterials.2017.08.037
Jiang, Y., Huang, J., Zhen, X., Zeng, Z., Li, J., Xie, C., et al. (2019b). A generic approach towards afterglow luminescent nanoparticles for ultrasensitive in vivo imaging. Nat. Commun. 10, 2064. doi:10.1038/s41467-019-10119-x
June, C. H., O'Connor, R. S., Kawalekar, O. U., Ghassemi, S., and Milone, M. C. (2018). CAR T-cell immunotherapy for human cancer. Science 359, 1361–1365. doi:10.1126/science.aar6711
Kang, H., Rho, S., Stiles, W. R., Hu, S., Baek, Y., Hwang, D. W., et al. (2020). Size-dependent EPR effect of polymeric nanoparticles on tumor targeting. Adv. Healthc. Mat. 9, e1901223. doi:10.1002/adhm.201901223
Karousis, N., Suarez-Martinez, I., Ewels, C. P., and Tagmatarchis, N. (2016). Structure, properties, functionalization, and applications of carbon nanohorns. Chem. Rev. 116, 4850–4883. doi:10.1021/acs.chemrev.5b00611
Khatami, F., Matin, M. M., Danesh, N. M., Bahrami, A. R., Abnous, K., Taghdisi, S. M., et al. (2021). Targeted delivery system using silica nanoparticles coated with chitosan and AS1411 for combination therapy of doxorubicin and antimiR-21. Carbohydr. Polym. 266, 118111. doi:10.1016/j.carbpol.2021.118111
Khoobchandani, M., Khan, A., Katti, K. K., Thipe, V. C., Al-Yasiri, A. Y., MohanDoss, D. K. D., et al. (2021). Green nanotechnology of MGF-AuNPs for immunomodulatory intervention in prostate cancer therapy. Sci. Rep. 11, 16797. doi:10.1038/s41598-021-96224-8
Kohout, C., Santi, C., and Polito, L. (2018). Anisotropic gold nanoparticles in biomedical applications. Int. J. Mol. Sci. 19, 3385. doi:10.3390/ijms19113385
Kroschinsky, F., Stölzel, F., von Bonin., S., Beutel, G., Kochanek, M., Kiehl, M., et al. (2017). New drugs, new toxicities: Severe side effects of modern targeted and immunotherapy of cancer and their management. Crit. Care 21, 89. doi:10.1186/s13054-017-1678-1
Kumar, A. R., Devan, A. R., Nair, B., Vinod, B. S., and Nath, L. R. (2021). Harnessing the immune system against cancer: Current immunotherapy approaches and therapeutic targets. Mol. Biol. Rep. 48, 8075–8095. doi:10.1007/s11033-021-06752-9
Lan, G., Ni, K., Xu, Z., Veroneau, S. S., Song, Y., and Lin, W. (2018). Nanoscale metal-organic framework overcomes hypoxia for photodynamic therapy primed cancer immunotherapy. J. Am. Chem. Soc. 140, 5670–5673. doi:10.1021/jacs.8b01072
Lee, H. P., and Gaharwar, A. K. (2020). Light-responsive inorganic biomaterials for biomedical applications. Adv. Sci. (Weinh) 7, 2000863. doi:10.1002/advs.202000863
Lee, J., Chuang, T. H., Redecke, V., She, L., Pitha, P. M., Carson, D. A., et al. (2003). Molecular basis for the immunostimulatory activity of guanine nucleoside analogs: Activation of toll-like receptor 7. Proc. Natl. Acad. Sci. U. S. A. 100, 6646–6651. doi:10.1073/pnas.0631696100
Lee, S. H., Rho, W. Y., Chang, H., Lee, J. H., Kim, J., Lee, S. H., et al. (2021). Carbon nanomaterials for biomedical application. Adv. Exp. Med. Biol. 1309, 257–276. doi:10.1007/978-981-33-6158-4_11
Lesch, S., and Gill, S. (2021). The promise and perils of immunotherapy. Blood Adv. 5, 3709–3725. doi:10.1182/bloodadvances.2021004453c
Li, G., Sun, B., Li, Y., Luo, C., He, Z., and Sun, J. (2021a). Small-molecule prodrug nanoassemblies: An emerging nanoplatform for anticancer drug delivery. Small 17, e2101460. doi:10.1002/smll.202101460
Li, H., Wang, X., Huang, D., and Chen, G. (2020a). Recent advances of lanthanide-doped upconversion nanoparticles for biological applications. Nanotechnology 31, 072001. doi:10.1088/1361-6528/ab4f36
Li, J., Cui, D., Jiang, Y., Huang, J., Cheng, P., and Pu, K. (2019a). Near-infrared photoactivatable semiconducting polymer nanoblockaders for metastasis-inhibited combination cancer therapy. Adv. Mat. 31, e1905091. doi:10.1002/adma.201905091
Li, J., Huang, D., Cheng, R., Figueiredo, P., Fontana, F., Correia, A., et al. (2021b). Multifunctional biomimetic nanovaccines based on photothermal and weak-immunostimulatory nanoparticulate cores for the immunotherapy of solid tumors. Adv. Mat. 34, e2108012. doi:10.1002/adma.202108012
Li, J., and Pu, K. (2020). Semiconducting polymer nanomaterials as near-infrared photoactivatable protherapeutics for cancer. Acc. Chem. Res. 53, 752–762. doi:10.1021/acs.accounts.9b00569
Li, Q., Shi, Z., Zhang, F., Zeng, W., Zhu, D., and Mei, L. (2022). Symphony of nanomaterials and immunotherapy based on the cancer-immunity cycle. Acta Pharm. Sin. B 12, 107–134. doi:10.1016/j.apsb.2021.05.031
Li, T., Li, C., Ruan, Z., Xu, P., Yang, X., Yuan, P., et al. (2019b). Polypeptide-conjugated second near-infrared organic fluorophore for image-guided photothermal therapy. ACS Nano 13, 3691–3702. doi:10.1021/acsnano.9b00452
Li, W., Liu, Z., Fontana, F., Ding, Y., Liu, D., Hirvonen, J. T., et al. (2018). Tailoring porous silicon for biomedical applications: From drug delivery to cancer immunotherapy. Adv. Mat. 30, e1703740. doi:10.1002/adma.201703740
Li, W., Peng, A., Wu, H., Quan, Y., Li, Y., Lu, L., et al. (2020b). Anti-cancer nanomedicines: A revolution of tumor immunotherapy. Front. Immunol. 11, 601497. doi:10.3389/fimmu.2020.601497
Li, X., Liu, L., Li, S., Wan, Y., Chen, J. X., Tian, S., et al. (2019c). Biodegradable π-conjugated oligomer nanoparticles with high photothermal conversion efficiency for cancer theranostics. ACS Nano 13, 12901–12911. doi:10.1021/acsnano.9b05383
Li, Y., Liu, G., Ma, J., Lin, J., Lin, H., Su, G., et al. (2017). Chemotherapeutic drug-photothermal agent co-self-assembling nanoparticles for near-infrared fluorescence and photoacoustic dual-modal imaging-guided chemo-photothermal synergistic therapy. J. Control. Release 258, 95–107. doi:10.1016/j.jconrel.2017.05.011
Li, Y., Xie, Y., Wang, Z., Zang, N., Carniato, F., Huang, Y., et al. (2016). Structure and function of iron-loaded synthetic melanin. ACS Nano 10, 10186–10194. doi:10.1021/acsnano.6b05502
Li, Z., Zhu, L., Sun, H., Shen, Y., Hu, D., Wu, W., et al. (2020c). Fluorine assembly nanocluster breaks the shackles of immunosuppression to turn the cold tumor hot. Proc. Natl. Acad. Sci. U. S. A. 117, 32962–32969. doi:10.1073/pnas.2011297117
Lin, H., Gao, S., Dai, C., Chen, Y., and Shi, J. (2017). A two-dimensional biodegradable niobium carbide (MXene) for photothermal tumor eradication in NIR-I and NIR-II biowindows. J. Am. Chem. Soc. 139, 16235–16247. doi:10.1021/jacs.7b07818
Lin, N. S., Kitamura, M., Saito, M., Hirayama, K., Ide, Y., and Umemura, K. (2022). Distinguishing antioxidant molecules with near-infrared photoluminescence of DNA-wrapped single-walled carbon nanotubes. ACS Omega 7, 28896–28903. doi:10.1021/acsomega.2c02038
Liu, J., He, S., Luo, Y., Zhang, Y., Du, X., Xu, C., et al. (2022). Tumor-microenvironment-activatable polymer nano-immunomodulator for precision cancer photoimmunotherapy. Adv. Mat. 34, e2106654. doi:10.1002/adma.202106654
Liu, J., Li, R., and Yang, B. (2020). Carbon dots: A new type of carbon-based nanomaterial with wide applications. ACS Cent. Sci. 6, 2179–2195. doi:10.1021/acscentsci.0c01306
Liu, J., Liang, H., Li, M., Luo, Z., Zhang, J., Guo, X., et al. (2018). Tumor acidity activating multifunctional nanoplatform for NIR-mediated multiple enhanced photodynamic and photothermal tumor therapy. Biomaterials 157, 107–124. doi:10.1016/j.biomaterials.2017.12.003
Liu, X., Chen, Z. H., Zhang, H., Fan, Y., and Zhang, F. (2021a). Independent luminescent lifetime and intensity tuning of upconversion nanoparticles by gradient doping for multiplexed encoding. Angew. Chem. Int. Ed. 60, 7041–7045. doi:10.1002/anie.202015273
Liu, Y., Jiao, F., Qiu, Y., Li, W., Qu, Y., Tian, C., et al. (2009). Immunostimulatory properties and enhanced TNF- alpha mediated cellular immunity for tumor therapy by C60(OH)20 nanoparticles. Nanotechnology 20, 415102. doi:10.1088/0957-4484/20/41/415102
Liu, Y. Q., Qin, L. Y., Li, H. J., Wang, Y. X., Zhang, R., Shi, J. M., et al. (2021b). Application of lanthanide-doped upconversion nanoparticles for cancer treatment: A review. Nanomedicine 16, 2207–2242. doi:10.2217/nnm-2021-0214
Lu, H., Zhao, Q., Wang, X., Mao, Y., Chen, C., Gao, Y., et al. (2020). Multistimuli responsive mesoporous silica-coated carbon nanoparticles for chemo-photothermal therapy of tumor. Colloids Surf. B Biointerfaces 190, 110941. doi:10.1016/j.colsurfb.2020.110941
Luo, C., Sun, J., Sun, B., Liu, D., Miao, L., Goodwin, T. J., et al. (2016). Facile fabrication of tumor redox-sensitive nanoassemblies of small-molecule oleate prodrug as potent chemotherapeutic nanomedicine. Small 12, 6353–6362. doi:10.1002/smll.201601597
Luo, L., Yang, J., Zhu, C., Jiang, M., Guo, X., Li, W., et al. (2018). Sustained release of anti-PD-1 peptide for perdurable immunotherapy together with photothermal ablation against primary and distant tumors. J. Control. Release 278, 87–99. doi:10.1016/j.jconrel.2018.04.002
Lyu, Y., Zeng, J., Jiang, Y., Zhen, X., Wang, T., Qiu, S., et al. (2018). Enhancing both biodegradability and efficacy of semiconducting polymer nanoparticles for photoacoustic imaging and photothermal therapy. ACS Nano 12, 1801–1810. doi:10.1021/acsnano.7b08616
Ma, W., Cheetham, A. G., and Cui, H. (2016). Building nanostructures with drugs. Nano Today 11, 13–30. doi:10.1016/j.nantod.2015.11.003
Ma, W., Zhu, D., Li, J., Chen, X., Xie, W., Jiang, X., et al. (2020). Coating biomimetic nanoparticles with chimeric antigen receptor T-cell-membrane provides high specificity for hepatocellular carcinoma photothermal therapy treatment. Theranostics 10, 1281–1295. doi:10.7150/thno.40291
Ma, Y., Zhang, Y., Li, X., Zhao, Y., Li, M., Jiang, W., et al. (2019). Near-infrared II phototherapy induces deep tissue immunogenic cell death and potentiates cancer immunotherapy. ACS Nano 13, 11967–11980. doi:10.1021/acsnano.9b06040
Mao, D., Hu, F., Yi, Z., Kenry,, , Xu, S., Yan, S., et al. (2020). AIEgen-coupled upconversion nanoparticles eradicate solid tumors through dual-mode ROS activation. Sci. Adv. 6, eabb2712. doi:10.1126/sciadv.abb2712
Martín-Palma, R. J., Hernández-Montelongo, J., Torres-Costa, V., Manso-Silván, M., and Muñoz-Noval, Á. (2014). Nanostructured porous silicon-mediated drug delivery. Expert Opin. Drug Deliv. 11, 1273–1283. doi:10.1517/17425247.2014.919254
Mohanty, R., Chowdhury, C. R., Arega, S., Sen, P., Ganguly, P., and Ganguly, N. (2019). CAR T cell therapy: A new era for cancer treatment (review). Oncol. Rep. 42, 2183–2195. doi:10.3892/or.2019.7335
Moradipour, M., Chase, E. K., Khan, M. A., Asare, S. O., Lynn, B. C., Rankin, S. E., et al. (2020). Interaction of lignin-derived dimer and eugenol-functionalized silica nanoparticles with supported lipid bilayers. Colloids Surf. B Biointerfaces 191, 111028. doi:10.1016/j.colsurfb.2020.111028
Muluh, T. A., Chen, Z., Li, Y., Xiong, K., Jin, J., Fu, S., et al. (2021). Enhancing cancer immunotherapy treatment goals by using nanoparticle delivery system. Int. J. Nanomedicine 16, 2389–2404. doi:10.2147/IJN.S295300
Osipov, A., Saung, M. T., Zheng, L., and Murphy, A. G. (2019). Small molecule immunomodulation: The tumor microenvironment and overcoming immune escape. J. Immunother. Cancer 7, 224. doi:10.1186/s40425-019-0667-0
Park, W., Heo, Y. J., and Han, D. K. (2018). New opportunities for nanoparticles in cancer immunotherapy. Biomater. Res. 22, 24. doi:10.1186/s40824-018-0133-y
Pitt, J. M., Marabelle, A., Eggermont, A., Soria, J. C., Kroemer, G., and Zitvogel, L. (2016). Targeting the tumor microenvironment: Removing obstruction to anticancer immune responses and immunotherapy. Ann. Oncol. 27, 1482–1492. doi:10.1093/annonc/mdw168
Popescu, R. C., Fufă, M. O., and Grumezescu, A. M. (2015). Metal-based nanosystems for diagnosis. Rom. J. Morphol. Embryol. 56, 635–649.
Porter, A. E., Gass, M., Muller, K., Skepper, J. N., Midgley, P. A., and Welland, M. (2007). Direct imaging of single-walled carbon nanotubes in cells. Nat. Nanotechnol. 2, 713–717. doi:10.1038/nnano.2007.347
Qian, C. G., Chen, Y. L., Feng, P. J., Xiao, X. Z., Dong, M., Yu, J. C., et al. (2017). Conjugated polymer nanomaterials for theranostics. Acta Pharmacol. Sin. 38, 764–781. doi:10.1038/aps.2017.42
Qiu, H., Tan, M., Ohulchanskyy, T. Y., Lovell, J. F., and Chen, G. (2018). Recent progress in upconversion photodynamic therapy. Nanomater. (Basel). 8, 344. doi:10.3390/nano8050344
Ribas, A., and Wolchok, J. D. (2018). Cancer immunotherapy using checkpoint blockade. Science 359, 1350–1355. doi:10.1126/science.aar4060
Riley, R. S., June, C. H., Langer, R., and Mitchell, M. J. (2019). Delivery technologies for cancer immunotherapy. Nat. Rev. Drug Discov. 18, 175–196. doi:10.1038/s41573-018-0006-z
Riley, R. S., O'Sullivan, R. K., Potocny, A. M., Rosenthal, J., and Day, E. S. (2018). Evaluating nanoshells and a potent biladiene photosensitizer for dual photothermal and photodynamic therapy of triple negative breast cancer cells. Nanomater. (Basel). 8, 658. doi:10.3390/nano8090658
Sadat Tabatabaei Mirakabad, F., Nejati-Koshki, K., Akbarzadeh, A., Yamchi, M. R., Milani, M., Zarghami, N., et al. (2014). PLGA-based nanoparticles as cancer drug delivery systems. Asian pac. J. Cancer Prev. 15, 517–535. doi:10.7314/apjcp.2014.15.2.517
Sainz-Urruela, C., Vera-López, S., San Andrés, M. P., and Díez-Pascual, A. M. (2021). Graphene-based sensors for the detection of bioactive compounds: A review. Int. J. Mol. Sci. 22, 3316. doi:10.3390/ijms22073316
Sang, W., Zhang, Z., Dai, Y., and Chen, X. (2019). Recent advances in nanomaterial-based synergistic combination cancer immunotherapy. Chem. Soc. Rev. 48, 3771–3810. doi:10.1039/c8cs00896e
Shao, Y., Liu, B., Di, Z., Zhang, G., Sun, L. D., Li, L., et al. (2020). Engineering of upconverted metal-organic frameworks for near-infrared light-triggered combinational photodynamic/chemo-/immunotherapy against hypoxic tumors. J. Am. Chem. Soc. 142, 3939–3946. doi:10.1021/jacs.9b12788
Shemesh, C. S., Hsu, J. C., Hosseini, I., Shen, B. Q., Rotte, A., Twomey, P., et al. (2021). Personalized cancer vaccines: Clinical landscape, challenges, and opportunities. Mol. Ther. 29, 555–570. doi:10.1016/j.ymthe.2020.09.038
Sheng, G., Tian, N., Duan, H. J., Sun, Z. G., and Chu, H. Q. (2022). Advances in therapeutic nanodrug delivery systems for infectious lung diseases: A review. Acta Mat. Med. 1, 343–364. doi:10.15212/AMM-2022-0019
Shi, C., Li, M., Zhang, Z., Yao, Q., Shao, K., Xu, F., et al. (2020). Catalase-based liposomal for reversing immunosuppressive tumor microenvironment and enhanced cancer chemo-photodynamic therapy. Biomaterials 233, 119755. doi:10.1016/j.biomaterials.2020.119755
Shoshan, M. S., Vonderach, T., Hattendorf, B., and Wennemers, H. (2019). Peptide-coated platinum nanoparticles with selective toxicity against liver cancer cells. Angew. Chem. Int. Ed. 58, 4901–4905. doi:10.1002/anie.201813149
Siegel, R. L., Miller, K. D., Fuchs, H. E., and Jemal, A. (2021). Cancer statistics, 2021. CA Cancer J. Clin. 71, 7–33. doi:10.3322/caac.21654
Singh, P., Pandit, S., Mokkapati, V. R. S. S., Garg, A., Ravikumar, V., and Mijakovic, I. (2018). Gold nanoparticles in diagnostics and therapeutics for human cancer. Int. J. Mol. Sci. 19, 1979. doi:10.3390/ijms19071979
Son, J., Yi, G., Yoo, J., Park, C., Koo, H., and Choi, H. S. (2019). Light-responsive nanomedicine for biophotonic imaging and targeted therapy. Adv. Drug Deliv. Rev. 138, 133–147. doi:10.1016/j.addr.2018.10.002
Song, Y., Wang, L., and Xie, Z. (2021). Metal-organic frameworks for photodynamic therapy: Emerging synergistic cancer therapy. Biotechnol. J. 16, e1900382. doi:10.1002/biot.201900382
Sun, J., Birnbaum, W., Anderski, J., Picker, M. T., Mulac, D., Langer, K., et al. (2018). Use of light-degradable aliphatic polycarbonate nanoparticles as drug carrier for photosensitizer. Biomacromolecules 19, 4677–4690. doi:10.1021/acs.biomac.8b01446
Sung, H., Ferlay, J., Siegel, R. L., Laversanne, M., Soerjomataram, I., Jemal, A., et al. (2021). Global cancer statistics 2020: GLOBOCAN estimates of incidence and mortality worldwide for 36 cancers in 185 countries. CA Cancer J. Clin. 71, 209–249. doi:10.3322/caac.21660
Tajabadi, M. (2019). Application of carbon nanotubes in breast cancer therapy. Drug Res. doi:10.1055/a-0945-1469
Tang, H., Xu, X., Chen, Y., Xin, H., Wan, T., Li, B., et al. (2021). Reprogramming the tumor microenvironment through second-near-infrared-window photothermal genome editing of PD-L1 mediated by supramolecular gold nanorods for enhanced cancer immunotherapy. Adv. Mat. 33, e2006003. doi:10.1002/adma.202006003
Tao, Y., Ju, E., Ren, J., and Qu, X. (2014). Immunostimulatory oligonucleotides-loaded cationic graphene oxide with photothermally enhanced immunogenicity for photothermal/immune cancer therapy. Biomaterials 35, 9963–9971. doi:10.1016/j.biomaterials.2014.08.036
Tian, D., Qin, F., Zhao, H., Zhang, C., Wang, H., Liu, N., et al. (2021). Bio-Responsive nanoparticle for tumor targeting and enhanced photo-immunotherapy. Colloids Surf. B Biointerfaces 202, 111681. doi:10.1016/j.colsurfb.2021.111681
Tieu, T., Wojnilowicz, M., Huda, P., Thurecht, K. J., Thissen, H., Voelcker, N. H., et al. (2021). Nanobody-displaying porous silicon nanoparticles for the co-delivery of siRNA and doxorubicin. Biomater. Sci. 9, 133–147. doi:10.1039/d0bm01335h
Topalian, S. L. (2017). Targeting immune checkpoints in cancer therapy. JAMA 318, 1647–1648. doi:10.1001/jama.2017.14155
Tuncel, D., and Demir, H. V. (2010). Conjugated polymer nanoparticles. Nanoscale 2, 484–494. doi:10.1039/b9nr00374f
Ulbrich, K., Holá, K., Šubr, V., Bakandritsos, A., Tuček, J., and Zbořil, R. (2016). Targeted drug delivery with polymers and magnetic nanoparticles: Covalent and noncovalent approaches, release control, and clinical studies. Chem. Rev. 116, 5338–5431. doi:10.1021/acs.chemrev.5b00589
Velpurisiva, P., Gad, A., Piel, B., Jadia, R., and Rai, P. (2017). Nanoparticle design strategies for effective cancer immunotherapy. J. Biomed. (Syd). 2, 64–77. doi:10.7150/jbm.18877
Vimbela, G. V., Ngo, S. M., Fraze, C., Yang, L., and Stout, D. A. (2017). Antibacterial properties and toxicity from metallic nanomaterials. Int. J. Nanomedicine 12, 3941–3965. doi:10.2147/IJN.S134526
Wang, C., Xu, L., Liang, C., Xiang, J., Peng, R., and Liu, Z. (2014). Immunological responses triggered by photothermal therapy with carbon nanotubes in combination with anti-CTLA-4 therapy to inhibit cancer metastasis. Adv. Mat. 26, 8154–8162. doi:10.1002/adma.201402996
Wang, H., Pan, X., Wang, X., Wang, W., Huang, Z., Gu, K., et al. (2020). Degradable carbon-silica nanocomposite with immunoadjuvant property for dual-modality photothermal/photodynamic therapy. ACS Nano 14, 2847–2859. doi:10.1021/acsnano.9b06168
Wang, M., Song, J., Zhou, F., Hoover, A. R., Murray, C., Zhou, B., et al. (2019a). NIR-triggered phototherapy and immunotherapy via an antigen-capturing nanoplatform for metastatic cancer treatment. Adv. Sci. (Weinh). 6, 1802157. doi:10.1002/advs.201802157
Wang, S., Wu, W., Manghnani, P., Xu, S., Wang, Y., Goh, C. C., et al. (2019b). Polymerization-enhanced two-photon photosensitization for precise photodynamic therapy. ACS Nano 13, 3095–3105. doi:10.1021/acsnano.8b08398
Wang, X., Zhong, X., Li, J., Liu, Z., and Cheng, L. (2021). Inorganic nanomaterials with rapid clearance for biomedical applications. Chem. Soc. Rev. 50, 8669–8742. doi:10.1039/d0cs00461h
Wang, Y., Cheetham, A. G., Angacian, G., Su, H., Xie, L., and Cui, H. (2017a). Peptide-drug conjugates as effective prodrug strategies for targeted delivery. Adv. Drug Deliv. Rev. 110-111, 112–126. doi:10.1016/j.addr.2016.06.015
Wang, Y. F., Liu, G. Y., Sun, L. D., Xiao, J. W., Zhou, J. C., and Yan, C. H. (2013). Nd (3+)-sensitized upconversion nanophosphors: Efficient in vivo bioimaging probes with minimized heating effect. ACS Nano 7, 7200–7206. doi:10.1021/nn402601d
Wang, Y., Zheng, K., Song, S., Fan, D., Zhang, H., and Liu, X. (2018). Remote manipulation of upconversion luminescence. Chem. Soc. Rev. 47, 6473–6485. doi:10.1039/c8cs00124c
Wang, Z., and Cao, Y. J. (2020). Adoptive cell therapy targeting neoantigens: A frontier for cancer research. Front. Immunol. 11, 176. doi:10.3389/fimmu.2020.00176
Wang, Z., Guo, B., Middha, E., Huang, Z., Hu, Q., Fu, Z., et al. (2019c). Microfluidics-prepared uniform conjugated polymer nanoparticles for photo-triggered immune microenvironment modulation and cancer therapy. ACS Appl. Mat. Interfaces 11, 11167–11176. doi:10.1021/acsami.8b22579
Wang, Z. X., Li, Y., Huang, Y., Parent, Y., Ditri, L. R., Zang, T., et al. (2017b). Tunable, metal-loaded polydopamine nanoparticles analyzed by magnetometry. Chem. Mat. 29, 8195–8201. doi:10.1021/acs.chemmater.7b02262
Wei, J., Liu, Y., Yu, J., Chen, L., Luo, M., Yang, L., et al. (2021). Conjugated polymers: Optical toolbox for bioimaging and cancer therapy. Small 17, e2103127. doi:10.1002/smll.202103127
Wei, Z., Wu, M., Lan, S., Li, J., Zhang, X., Zhang, D., et al. (2018). Semiconducting polymer-based nanoparticles for photothermal therapy at the second near-infrared window. Chem. Commun. (Camb) 54, 13599–13602. doi:10.1039/c8cc07583b
Wen, S., Zhou, J., Zheng, K., Bednarkiewicz, A., Liu, X., and Jin, D. (2018). Advances in highly doped upconversion nanoparticles. Nat. Commun. 9, 2415. doi:10.1038/s41467-018-04813-5
Wiehe, A., O'Brien, J. M., and Senge, M. O. (2019). Trends and targets in antiviral phototherapy. Photochem. Photobiol. Sci. 18, 2565–2612. doi:10.1039/c9pp00211a
Xia, H., Li, F., Hu, X., Park, W., Wang, S., Jang, Y., et al. (2016). pH-sensitive Pt nanocluster assembly overcomes cisplatin resistance and heterogeneous stemness of hepatocellular carcinoma. ACS Cent. Sci. 2, 802–811. doi:10.1021/acscentsci.6b00197
Xiang, J., Xu, L., Gong, H., Zhu, W., Wang, C., Xu, J., et al. (2015). Antigen-loaded upconversion nanoparticles for dendritic cell stimulation, tracking, and vaccination in dendritic cell-based immunotherapy. ACS Nano 9, 6401–6411. doi:10.1021/acsnano.5b02014
Xiao, Y., and Yu, D. (2021). Tumor microenvironment as a therapeutic target in cancer. Pharmacol. Ther. 221, 107753. doi:10.1016/j.pharmthera.2020.107753
Xie, D., Jiang, Y., Li, K., Yang, X., and Zhang, Y. (2022). Pickering emulsions stabilized by mesoporous nanoparticles with different morphologies in combination with DTAB. ACS Omega 7, 29153–29160. doi:10.1021/acsomega.2c03215
Xie, Z., Chen, S., Duo, Y., Zhu, Y., Fan, T., Zou, Q., et al. (2019). Biocompatible two-dimensional titanium nanosheets for multimodal imaging-guided cancer theranostics. ACS Appl. Mat. Interfaces 11, 22129–22140. doi:10.1021/acsami.9b04628
Xu, J., Xu, L., Wang, C., Yang, R., Zhuang, Q., Han, X., et al. (2017). Near-infrared-triggered photodynamic therapy with multitasking upconversion nanoparticles in combination with checkpoint blockade for immunotherapy of colorectal cancer. ACS Nano 11, 4463–4474. doi:10.1021/acsnano.7b00715
Xu, N., Hu, A., Pu, X., Li, J., Wang, X., Wang, J., et al. (2022). Fe (III)-chelated polydopamine nanoparticles for synergistic tumor therapies of enhanced photothermal ablation and antitumor immune activation. ACS Appl. Mat. Interfaces 14, 15894–15910. doi:10.1021/acsami.1c24066
Xue, X., Lindstrom, A., and Li, Y. (2019). Porphyrin-based nanomedicines for cancer treatment. Bioconjug. Chem. 30, 1585–1603. doi:10.1021/acs.bioconjchem.9b00231
Xue, X., Lindstrom, A., Qu, H., and Li, Y. (2020). Recent advances on small-molecule nanomedicines for cancer treatment. Wiley Interdiscip. Rev. Nanomed. Nanobiotechnol. 12, e1607. doi:10.1002/wnan.1607
Yan, L., and Li, X. (2016). Biodegradable stimuli-responsive polymeric micelles for treatment of malignancy. Curr. Pharm. Biotechnol. 17, 227–236. doi:10.2174/138920101703160206142821
Yan, Q., Han, D., and Zhao, Y. (2013). Main-chain photoresponsive polymers with controlled location of light-cleavable units: From synthetic strategies to structural engineering. Polym. Chem. 4, 5026–5037. doi:10.1039/c3py00804e
Yang, F., Shi, K., Jia, Y. P., Hao, Y., Peng, J. R., and Qian, Z. Y. (2020a). Advanced biomaterials for cancer immunotherapy. Acta Pharmacol. Sin. 41, 911–927. doi:10.1038/s41401-020-0372-z
Yang, H., He, L. Q., Hu, Y. W., Lu, X., Li, G. R., Liu, B., et al. (2015). Quantitative detection of photothermal and photoelectrocatalytic effects induced by SPR from Au@Pt nanoparticles. Angew. Chem. Int. Ed. 54, 11462–11466. doi:10.1002/anie.201505985
Yang, J., Zhang, X., Liu, C., Wang, Z., Cui, W., Feng, C., et al. (2020b). Biologically modified nanoparticles as theranostic bionanomaterials. Prog. Mat. Sci. 118, 100768. doi:10.1016/J.PMATSCI.2020.100768
Yang, L. X., Wu, Y. N., Wang, P. W., Huang, K. J., Su, W. C., and Shieh, D. B. (2020c). Silver-coated zero-valent iron nanoparticles enhance cancer therapy in mice through lysosome-dependent dual programed cell death pathways: Triggering simultaneous apoptosis and autophagy only in cancerous cells. J. Mat. Chem. B 8, 4122–4131. doi:10.1039/c9tb01477b
Yang, Q., Peng, J., Shi, K., Xiao, Y., Liu, Q., Han, R., et al. (2019). Rationally designed peptide-conjugated gold/platinum nanosystem with active tumor-targeting for enhancing tumor photothermal-immunotherapy. J. Control. Release 308, 29–43. doi:10.1016/j.jconrel.2019.06.031
Yang, Y., Fan, X., Li, L., Yang, Y., Nuernisha, A., Xue, D., et al. (2020d). Semiconducting polymer nanoparticles as theranostic system for near-infrared-II fluorescence imaging and photothermal therapy under safe laser fluence. ACS Nano 14, 2509–2521. doi:10.1021/acsnano.0c00043
Yata, T., Takahashi, Y., Tan, M., Nakatsuji, H., Ohtsuki, S., Murakami, T., et al. (2017). DNA nanotechnology-based composite-type gold nanoparticle-immunostimulatory DNA hydrogel for tumor photothermal immunotherapy. Biomaterials 146, 136–145. doi:10.1016/j.biomaterials.2017.09.014
Yin, C., Zhen, X., Zhao, H., Tang, Y., Ji, Y., Lyu, Y., et al. (2017). Amphiphilic semiconducting oligomer for near-infrared photoacoustic and fluorescence imaging. ACS Appl. Mat. Interfaces 9, 12332–12339. doi:10.1021/acsami.7b02014
Yu, C., Qian, L., Ge, J., Fu, J., Yuan, P., Yao, S. C., et al. (2016). Cell-penetrating poly(disulfide) assisted intracellular delivery of mesoporous silica nanoparticles for inhibition of miR-21 function and detection of subsequent therapeutic effects. Angew. Chem. Int. Ed. 55, 9272–9276. doi:10.1002/anie.201602188
Yu, J., Liu, S., Wang, Y., He, X., Zhang, Q., Qi, Y., et al. (2021). Synergistic enhancement of immunological responses triggered by hyperthermia sensitive Pt NPs via NIR laser to inhibit cancer relapse and metastasis. Bioact. Mat. 7, 389–400. doi:10.1016/j.bioactmat.2021.05.030
Yu, Y., Li, J., Song, B., Ma, Z., Zhang, Y., Sun, H., et al. (2022). Polymeric PD-L1 blockade nanoparticles for cancer photothermal-immunotherapy. Biomaterials 280, 121312. doi:10.1016/j.biomaterials.2021.121312
Yuan, X., Zhu, Y., Li, S., Wu, Y., Wang, Z., Gao, R., et al. (2022). Titanium nanosheet as robust and biosafe drug carrier for combined photochemo cancer therapy. J. Nanobiotechnology 20, 154. doi:10.1186/s12951-022-01374-0
Yue, J., Mei, Q., Wang, P., Miao, P., Dong, W. F., and Li, L. (2022). Light-triggered multifunctional nanoplatform for efficient cancer photoimmunotherapy. J. Nanobiotechnology 20, 181. doi:10.1186/s12951-022-01388-8
Zaimy, M. A., Saffarzadeh, N., Mohammadi, A., Pourghadamyari, H., Izadi, P., Sarli, A., et al. (2017). New methods in the diagnosis of cancer and gene therapy of cancer based on nanoparticles. Cancer Gene Ther. 24, 233–243. doi:10.1038/cgt.2017.16
Zang, X., Zhao, X., Hu, H., Qiao, M., Deng, Y., and Chen, D. (2017). Nanoparticles for tumor immunotherapy. Eur. J. Pharm. Biopharm. 115, 243–256. doi:10.1016/j.ejpb.2017.03.013
Zeng, Y., Xiang, Y., Sheng, R., Tomás, H., Rodrigues, J., Gu, Z., et al. (2021). Polysaccharide-based nanomedicines for cancer immunotherapy: A review. Bioact. Mat. 6, 3358–3382. doi:10.1016/j.bioactmat.2021.03.008
Zhan, Y., Cao, X., Li, Y., Tian, J., Liang, J., Chen, X., et al. (2017). Silica cross-linked micellar core-shell nanoparticles encapsulating IR-780 with strong bright and good biocompatibility for optical imaging in vivo. J. Biomed. Nanotechnol. 13, 144–154. doi:10.1166/jbn.2017.2332
Zhang, C., Zeng, Z., Cui, D., He, S., Jiang, Y., Li, J., et al. (2021). Semiconducting polymer nano-PROTACs for activatable photoimmunometabolic cancer therapy. Nat. Commun. 12, 2934. doi:10.1038/s41467-021-23194-w
Zhang, D. X., Esser, L., Vasani, R. B., Thissen, H., and Voelcker, N. H. (2019a). Porous silicon nanomaterials: Recent advances in surface engineering for controlled drug-delivery applications. Nanomedicine 14, 3213–3230. doi:10.2217/nnm-2019-0167
Zhang, H., Liu, K., Li, S., Xin, X., Yuan, S., Ma, G., et al. (2018a). Self-assembled minimalist multifunctional theranostic nanoplatform for magnetic resonance imaging-guided tumor photodynamic therapy. ACS Nano 12, 8266–8276. doi:10.1021/acsnano.8b03529
Zhang, M., Liu, J., Kuang, Y., Li, Q., Zheng, D. W., Song, Q., et al. (2017). Ingenious pH-sensitive dextran/mesoporous silica nanoparticles based drug delivery systems for controlled intracellular drug release. Int. J. Biol. Macromol. 98, 691–700. doi:10.1016/j.ijbiomac.2017.01.136
Zhang, N., Li, M., Sun, X., Jia, H., and Liu, W. (2018b). NIR-responsive cancer cytomembrane-cloaked carrier-free nanosystems for highly efficient and self-targeted tumor drug delivery. Biomaterials 159, 25–36. doi:10.1016/j.biomaterials.2018.01.007
Zhang, P., Cheng, F., Zhou, R., Cao, J., Li, J., Burda, C., et al. (2014). DNA-hybrid-gated multifunctional mesoporous silica nanocarriers for dual-targeted and microRNA-responsive controlled drug delivery. Angew. Chem. Int. Ed. 53, 2371–2375. doi:10.1002/anie.201308920
Zhang, R., Xu, Y., Zhang, Y., Kim, H. S., Sharma, A., Gao, J., et al. (2019b). Rational design of a multifunctional molecular dye for dual-modal NIR-II/photoacoustic imaging and photothermal therapy. Chem. Sci. 10, 8348–8353. doi:10.1039/c9sc03504d
Zhang, W., Wang, F., Wang, Y., Wang, J., Yu, Y., Guo, S., et al. (2016). pH and near-infrared light dual-stimuli responsive drug delivery using DNA-conjugated gold nanorods for effective treatment of multidrug resistant cancer cells. J. Control. Release 232, 9–19. doi:10.1016/j.jconrel.2016.04.001
Zhang, X., An, L., Tian, Q., Lin, J., and Yang, S. (2020a). Tumor microenvironment-activated NIR-II reagents for tumor imaging and therapy. J. Mat. Chem. B 8, 4738–4747. doi:10.1039/d0tb00030b
Zhang, X., Li, H., Yi, C., Chen, G., Li, Y., Zhou, Y., et al. (2020b). Host immune response triggered by graphene quantum-dot-mediated photodynamic therapy for oral squamous cell carcinoma. Int. J. Nanomedicine 15, 9627–9638. doi:10.2147/IJN.S276153
Zhao, W., Zhao, Y., Wang, Q., Liu, T., Sun, J., and Zhang, R. (2019). Remote light-responsive nanocarriers for controlled drug delivery: Advances and perspectives. Small 15, 1903060. doi:10.1002/smll.201903060
Zhen, X., Pu, K., and Jiang, X. (2021). Photoacoustic imaging and photothermal therapy of semiconducting polymer nanoparticles: Signal amplification and second near-infrared construction. Small 17, e2004723. doi:10.1002/smll.202004723
Zhou, B., Guo, X., Yang, N., Huang, Z., Huang, L., Fang, Z., et al. (2021a). Surface engineering strategies of gold nanomaterials and their applications in biomedicine and detection. J. Mat. Chem. B 9, 5583–5598. doi:10.1039/d1tb00181g
Zhou, B., Shi, B., Jin, D., and Liu, X. (2015). Controlling upconversion nanocrystals for emerging applications. Nat. Nanotechnol. 10, 924–936. doi:10.1038/nnano.2015.251
Zhou, F., Wang, M., Luo, T., Qu, J., and Chen, W. R. (2021b). Photo-activated chemo-immunotherapy for metastatic cancer using a synergistic graphene nanosystem. Biomaterials 265, 120421. doi:10.1016/j.biomaterials.2020.120421
Zhou, F., Wu, S., Song, S., Chen, W. R., Resasco, D. E., and Xing, D. (2012). Antitumor immunologically modified carbon nanotubes for photothermal therapy. Biomaterials 33, 3235–3242. doi:10.1016/j.biomaterials.2011.12.029
Zhou, F., Xing, D., Ou, Z., Wu, B., Resasco, D. E., and Chen, W. R. (2009). Cancer photothermal therapy in the near-infrared region by using single-walled carbon nanotubes. J. Biomed. Opt. 14, 021009. doi:10.1117/1.3078803
Zhou, L., Fan, Y., Wang, R., Li, X., Fan, L., and Zhang, F. (2018). High-capacity upconversion wavelength and lifetime binary encoding for multiplexed biodetection. Angew. Chem. Int. Ed. 57, 12824–12829. doi:10.1002/anie.201808209
Zhou, W., Chen, Y., Zhang, Y., Xin, X., Li, R., Xie, C., et al. (2020). Iodine-rich semiconducting polymer nanoparticles for CT/fluorescence dual-modal imaging-guided enhanced photodynamic therapy. Small 16, e1905641. doi:10.1002/smll.201905641
Zhou, Z., Wang, Y., Yan, Y., Zhang, Q., and Cheng, Y. (2016). Dendrimer-templated ultrasmall and multifunctional photothermal agents for efficient tumor ablation. ACS Nano 10, 4863–4872. doi:10.1021/acsnano.6b02058
Zhu, H., Fang, Y., Miao, Q., Qi, X., Ding, D., Chen, P., et al. (2017). Regulating near-infrared photodynamic properties of semiconducting polymer nanotheranostics for optimized cancer therapy. ACS Nano 11, 8998–9009. doi:10.1021/acsnano.7b03507
Keywords: antitumor immunity, light-activated, nanomaterials, immunotherapy, synergistic therapy
Citation: Wang F, Duan H, Xu W, Sheng G, Sun Z and Chu H (2022) Light-activated nanomaterials for tumor immunotherapy. Front. Chem. 10:1031811. doi: 10.3389/fchem.2022.1031811
Received: 30 August 2022; Accepted: 20 September 2022;
Published: 07 October 2022.
Edited by:
Peisheng Zhang, Hunan University of Science and Technology, ChinaReviewed by:
Zhigang Xu, Southwest University, ChinaBowen Li, National University of Singapore, Singapore
Jingchao Li, Donghua University, China
Copyright © 2022 Wang, Duan, Xu, Sheng, Sun and Chu. This is an open-access article distributed under the terms of the Creative Commons Attribution License (CC BY). The use, distribution or reproduction in other forums is permitted, provided the original author(s) and the copyright owner(s) are credited and that the original publication in this journal is cited, in accordance with accepted academic practice. No use, distribution or reproduction is permitted which does not comply with these terms.
*Correspondence: Hongqian Chu, chuhongqian@bjxkyy.cn
†These authors have contributed equally to this work and share first authorship