- 1State Key Laboratory for Advanced Metals and Materials, University of Science and Technology Beijing, Beijing, China
- 2Beijing Key Laboratory for Chemical Power Source and Green Catalysis, Beijing Institute of Technology, Beijing, China
- 3School of Chemical Engineering and Pharmacy, Wuhan Institute of Technology, Wuhan, China
Carbon dioxide (CO2) gas is the main cause of global warming and has a significant effect on both climate change and human health. In this study, Ni/Ti co-doped Sr1.95Fe1.2Ni0.1Ti0.2Mo0.5O6-δ (SFNTM) double perovskite oxides were prepared and used as solid oxide electrolysis cell (SOEC) cathode materials for effective CO2 reduction. Ti-doping enhances the structural stability of the cathode material and increases the oxygen vacancy concentration. After treatment in 10% H2/Ar at 800°C, Ni nanoparticles were exsolved in situ on the SFNTM surface (Ni@SFNTM), thereby improving its chemisorption and activation capacity for CO2. Modified by the Ti-doping and the in situ exsolved Ni nanoparticles, the single cell with Ni@SFNMT cathode exhibits improved catalytic activity for CO2 reduction, exhibiting a current density of 2.54 A cm−2 at 1.8 V and 800°C. Furthermore, the single cell shows excellent stability after 100 h at 1.4 V, indicating that Ni/Ti co-doping is an effective strategy for designing novel cathode material with high electrochemical performance for SOEC.
Introduction
Carbon dioxide (CO2), a greenhouse gas and the main cause of global warming, has a significant impact on both climate change and human health. (Armaroli and Balzani, 2011; Broecker, 2018; Hoegh-Guldberg et al., 2019). The capture and utilization of CO2 is a promising approach to effectively reduce CO2 concentration and emissions (Fu et al., 2019; Karanikolos et al., 2021). It is known that CO can be further converted to low-carbon fuels and high value added chemicals through subsequent Fischer-Tropsch reactions (Zhang et al., 2002; Quadrelli et al., 2011). Therefore, converting CO2 to CO is a feasible and important method for CO2 utilization. Solid oxide electrolysis cells (SOEC) are high-efficiency electrochemical reactors that can convert CO2 into CO using distributed renewable energy, with an efficiency close to 100 % (Singh et al., 2015; Li et al., 2021). Normally, the CO2 reduction reaction (CO2-RR) occurs at the cathode of the SOEC, where CO2 is electrochemically converted to CO and oxygen ions under an applied potential. The generated oxygen ions are transferred across the solid electrolyte to the anode and converted to O2. The cathode is the core component in the SOEC, and dominates the electrochemical CO2-RR process and the performance of the SOEC.
Presently, Ni-YSZ is the most widely used cathode in SOEC for CO2-RR, owing to its good electrocatalytic activity and cost effectiveness (Song et al., 2018; Xu et al., 2019). However, certain inevitable issues hinder its further application, including Ni oxidation during the CO2/CO redox reaction process in the absence of a safe gas (H2/CO), carbon deposition at high CO concentrations, and Ni particle growth during long-term operation (Dong et al., 2017; Sciazko et al., 2021; Yang et al., 2022). Materials comprising perovskite oxide with mixed ionic-electronic conductivity, such as Sr2Fe1.5Mo0.5O6-δ (SFM), show promise for SOEC, and exhibit excellent redox stability, coke resistance, and long-term stability (Li et al., 2017a; Zhang et al., 2021). Nevertheless, compared with the Ni-YSZ cathode material, SFM oxide exhibits weak chemical CO2 adsorption and inadequate catalytic activity for CO2-RR, which limits the performance of CO2 electrolysis. Recently, the in situ exsolution of transition metal nanoparticles on the surface of perovskite oxide substrates has attracted extensive research attention and is regarded as a promising strategy to enhance the electrocatalytic activity of perovskite oxides. (Wang et al., 2019; Xu et al., 2021)
Briefly, the exsolved metal nanocatalysts, uniformly dispersed on the surface, can effectively strengthen the adsorption capacity of CO2 and improve the electrolysis activity for CO2-RR. Abundant oxygen vacancies were created during the reduction process, thereby supplying more active reaction regions for the chemisorption and catalytic activation of CO2 molecules on the electrode surface (Liu S. B. et al., 2016; Zheng et al., 2017). Furthermore, the metal nanoparticles were firmly anchored on the surface of the electrode material, restricting their aggregation. The strong interaction of the metal-oxide interface also exhibits exceptional electrocatalytic activity for the CO2-RR. Chen et al. concluded that exsolved NiFe alloy nanoparticles in Sr1.9Fe1.5Mo0.4Ni0.1O6-δ enhanced the chemisorption capacity and reaction kinetics of CO2 on the cathode surface (Wang et al., 2016). Bao et al. demonstrated that in situ exsolved FeNi3 nanoparticles on the Sr2Fe1.35Mo0.45Ni0.2O66-δ material strengthened CO2 adsorption and facilitated subsequent CO2-RR in SOEC (Lv et al., 2019). Luo et al. confirmed that in situ exsolved Fe-Ni nanospheres are evenly anchored on the perovskite oxide with oxygen defects, greatly enhancing the catalytic performance of the material as a highly stable and efficient catalyst (Liu S. et al., 2016). However, SFM-based double perovskite oxides tend to transform into Ruddlesden-Popper (RP)-type layer perovskite oxides during the in situ exsolution process in the reduction treatment, causing the initial perovskite structure to deform (Park et al., 2019; Park et al., 2020; Choi et al., 2021). The structural stability can be enhanced by employing stable valence state metallic elements, such as Ti4+, Zr4+, and Nb5+ in severe reducing atmospheres. For example, we introduced a stable valence Ti element to the B-site of SFM oxide, which notably improved its structural stability, produced abundant oxygen vacancies and increased the conductivity of the oxygen ions (Xu et al., 2022).
Herein, a Ti and Ni co-doped double perovskite oxide (Sr1.95Fe1.2Ni0.1Ti0.2Mo0.5O6-δ, SFNTM) was synthesized. After the reduction treatment, the oxide matrix maintained the original perovskite structure, while in situ exsolved Ni nanoparticles were uniformly anchored on its surface to form a metal-oxide heterostructure (Ni@SFNTM) as SOEC cathodes for CO2-RR at high temperatures. Subsequently, the phase structure, CO2 chemisorption and activation, and electrochemical properties of SFNTM and Ni@SFNTM samples were studied. The heterostructure formed by the in situ exsolved Ni nanoparticles and the SFNTM matrix can enhance the CO2 adsorption activity and expand the abundant CO2-RR active sites, thus further enhancing its performance.
Experimental section
Material preparation and cell fabrication
An Sr1.95Fe1.2Ni0.1Ti0.2Mo0.5O6-δ (SFNTM) sample was prepared using a modified sol-gel combustion method. The calculated stoichiometric ratios of Ni(NO3)2·6H2O, Sr(NO3)2, Fe(NO3)3·9H2O, and (NH4)6Mo7O24·4H2O were added to deionized water and stirred at 80°C. C16H36O4Ti was dissolved in ethanol, and the content was added dropwise into the abovementioned solution. Subsequently, citric acid and glycine were added, and a gel was obtained via continuous mixing. A black precursor powder was obtained by heating the gel at 250°C followed by sintering at 1,100°C for 5 h to synthesize SFNMT oxide. The La0.6Sr0.4Co0.2Fe0.8O3 (LSCF) cathode, Ce0.8Sm0.2O2 (SDC), and La0.8Sr0.2Ga0.8Mg0.2O3 (LSGM) electrolyte materials were purchased from Fuel Cell Co. The Ni@SFNTM sample was prepared by reducing the SFNTM in 5 % H2/Ar at 800°C for 5 h.
An electrolyte-supported single cell (SFNTM/Ni@SFNTM|SDC|LSGM|LSCF) was used. Dense disk-shaped LSGM electrolyte flakes (∼300 μm) were formed by dry-pressing and calcined at 1,450°C for 6 h. The SDC slurry was formulated by mixing SDC powder and a binder comprising a mixture of ethyl cellulose and α-terpineol, which was screen-printed onto the two sides of the LSGM electrolyte pellet and subsequently calcined at 1,350°C for 3 h. The LSCF and SFNTM/SFTM ink were formulated by combining the LSCF and SFNTM/SFTM powders with a binder. The LSCF anode and SFNTM/SFTM cathode ink were symmetrically screen-printed onto both sides of the electrolyte pellet and calcined at 1,100°C for 2 h. Silver ink and silver wires were used to cover the electrode surface and the electrode was fired at 750°C for 1 h to act as the current collector.
Characterization
The crystal structures of the as-synthesized and reduced SFNTM samples were investigated using X-ray diffraction (XRD, X'Pert Pro MPD diffractometer). The morphologies and microstructures of the reduced samples were observed using field-emission transmission electron microscopy (FETEM, JEM-2010F) and Scanning electron microscopy (SEM, FEI QUANTA-250). XPS (MULT1LAB 2000) was conducted to characterize the surface valence compositions of the different elements. Temperature-programmed desorption of CO2 (CO2-TPD) measurements were performed using a Micromeritics 2000 instrument with an Ar carrier gas, and the temperature was increased from 50°C to 1,000°C at a rate of 10°C min−1. The prepared materials were first treated under a He atmosphere at 300°C. The oxygen vacancy defects were detected by EPR (Bruker ELEXSYS E500). EPR tests were performed at 600 K with 10 mg sample and the sweep width is 1000G. The synthesized samples were tested by a four-probe direct current method. The Keithley 2,400 source meter was used to test on the dense bar shapes with a size of 2 mm × 4 mm×10 mm, which were prepared by dry pressing method and subsequently sintered at 1,200°C for 3 h, resulting in a density of over 95%. Electrical conductivity relaxation (ECR) measurement were performed under an abrupt switch of the atmosphere from 2:1 CO−CO2 to 1:1 CO−CO2. The variations in conductivity and test time were carried out until new equilibrium conditions are finally reached. Electrochemical impedance spectroscopy (EIS) of a single cell was performed using an AutoLab 302N at an open circuit voltage (OCV) by passing pure CO2 gas into the cathode at a flow rate of 50 ml min−1 while directly exposing the anode side to ambient gas. The corresponding AC impedance was analyzed using ZSimpWin software. The composition of the exhaust gas was evaluated using the online gas chromatography.
Results and discussion
Material structural characterization
XRD was used to characterize the crystal structures of the SFNTM and Ni@SFNTM samples at room temperature. Figure 1A shows the XRD pattern of the SFNTM sample after calcination at 1,100°C for 5 h and the XRD pattern of the Ni@SFNTM sample after reducing SFNTM in 5% H2/Ar at 800°C for 5 h. The SFNTM sample presents a pure cubic perovskite structure, which is consistent with that of the PDF card (PDF #34–0638) with no impurity phase; this result confirms that Ti and Ni were successfully co-doped into the perovskite structure. It should be noted that Ni@SFNTM maintains the cubic perovskite structure after the reducing treatment, which is consistent with the Rietveld refinement data (Supplementary Figure S1 and Supplementary Table S1). At the same time, there is an obvious Ni element peak in the XRD pattern of the Ni@SFNTM sample, which is consistent with that of the PDF card (PDF #34–0638), indicating that Ni can be exsolved in-situ from the SFNTM matrix under reducing conditions. Furthermore, the (110) peak of Ni@SFNTM shifts to a reduced angle, showing lattice expansion that results from the reduction of the B-site transition metal and the generation of oxygen vacancies. A small peak corresponding to Ni metal is observed for the Ni@SFNTM sample, showing that Ni can be exsolved in situ from the SFNTM matrix (Figure 1C). SEM analysis was used to observe the microstructures of the SFNTM and Ni@SFNTM particles. Figure 1B and Supplementary Figure S2 show that the SFNTM particles are interconnected with a smooth surface. After the reduction treatment, Ni@SFNTM presents a glossy surface, while spherical Ni nanoparticles with a mean size of 28 nm are uniformly distributed on the surface of the SFNTM perovskite substrate.
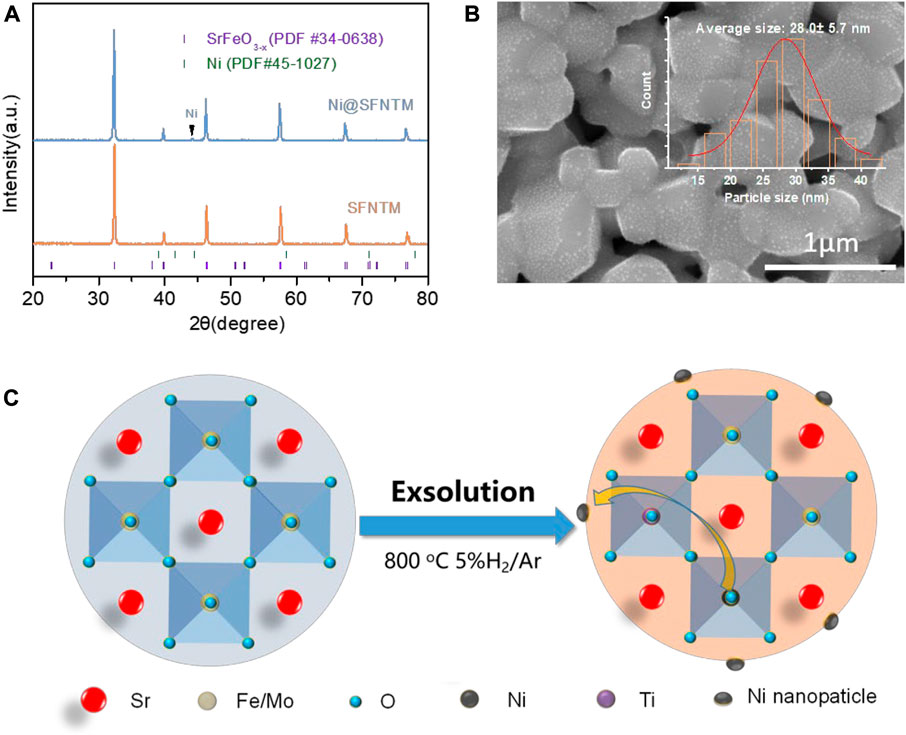
FIGURE 1. (A) XRD patterns of SFTM and Ni@SFNTM powders, (B) SEM image of Ni@SFNTM, inset is particle size distribution of Ni nanoparticles, (C) Schematic diagram of in situ exsolution of SFNTM under reducing conditions.
To further explore the composition and crystal structure of the synthesized samples, HRTEM characterization was performed on the Ni@SFNTM powder. As shown in Figure 2A, the SAED pattern comprises an image of the particles along the (111) crystal axis, which further confirms that the crystal structure of the SFNTM substrate is a cubic perovskite with a spatial group of Fm-3m. The observed lattice fringe distance of 0.283 nm corresponds to the (220) facet of the cubic SFNTM perovskite, which agrees with the XRD data. Moreover, as shown in Figure 2B, the interplanar spacing (0.203 nm) of the nanoparticles is assigned to the (111) plane of the Ni metal. The spherical Ni nanoparticles are partially anchored in the SFNTM substrate, suggesting extended catalytic active sites and strong bonding between the nanoparticles and the substrate, which greatly enhances the chemical and thermal stability. EDS mapping was performed to further determine the elemental composition of the Ni@SFNTM (Figure 2C). All the elements in the SFNTM substrate are evenly distributed. Exsolved Ni nanoparticles are also found on the surface of the SFNTM substrate particle.
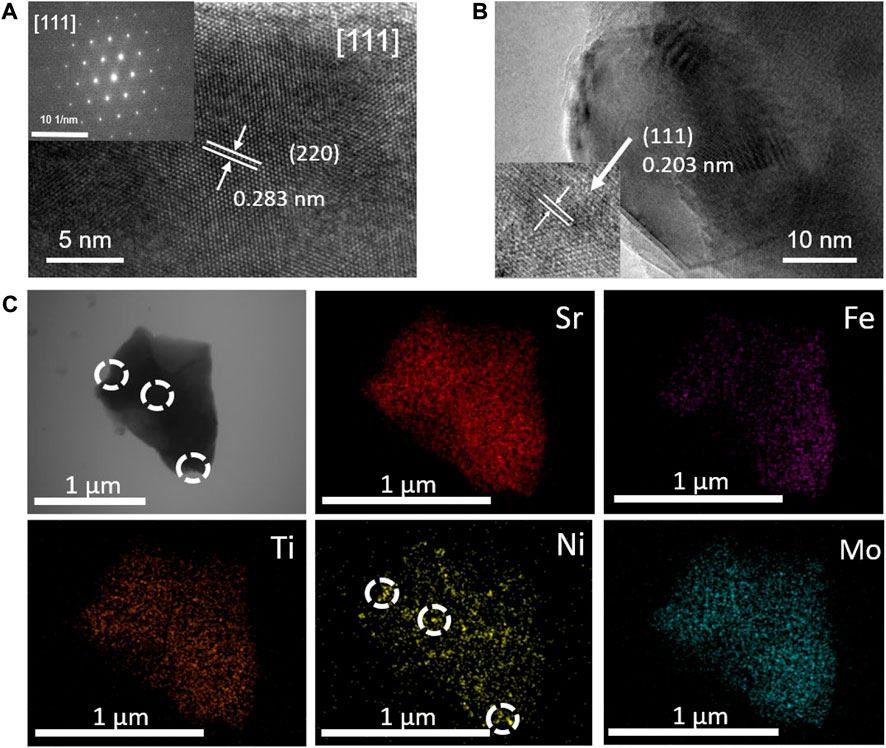
FIGURE 2. (A) HRTEM images and corresponding SEAD image patterns of SFNTM substrate; (B) HRTEM images of Ni nanoparticles; (C) STEM image and corresponding EDS maps of Ni@SFNTM
XPS was performed to determine the valence state changes of the surface elements in the as-prepared and reduced SFNTM samples. Figure 3A and Supplementary Table S2 show the Ni 2p spectra of the samples, before and after the reduction treatment. The figure shows a distinct Ni0 characteristic peak at ∼852.2 eV in the Ni@SFNTM sample, further proving that Ni metal particles can be exsolved from the perovskite substrate under a reducing atmosphere. Ni2+ peaks are also observed in the spectra, showing that only some Ni metal particles are exsolved from the substrate. Figures 3B,C show the XPS spectra of Fe 2p and Mo 3 d for the Ni@SFNTM and SFNTM samples at room temperature. The peaks at 709.7, 711.3 and 713.0 eV belong to Fe2+, Fe3+, and Fe4+, respectively (Zhang B.-W. et al., 2022). As shown in Supplementary Table S3, both the Fe3+ and Fe4+ contents decrease and the Fe2+ content increases after reduction; the average valence of Fe decreases from 3.00 to 2.79. Moreover, the Mo 3 d spectrum presents a spin-orbit doublet structure, belonging to a mixed state of Mo5+ and Mo6+. It can also be seen in Supplementary Table S4 that the Mo6+/Mo5+ ratio decreases after the reduction treatment and the average valence decreases from 5.60 to 5.55, indicating the generation of oxygen vacancies in the SFNTM substrate. The elemental valence states of the Ni, Fe, and Mo transition metals strongly affect the electrocatalytic activity and ionic conductivity of the two samples. The binding energy distributions of the O 1s spectrum are shown in Figure 3D. There are three distinct peaks in the O 1s spectrum corresponding to lattice oxygen (Olat), adsorbed oxygen species (Oads), and hydroxyl or carbonate (OH−/CO32−) on the powder surface (Zhou et al., 2018; Liu et al., 2021). From the fitting results (Supplementary Table S5), it can be seen that the ratio of Oads/Olat increases from 19.5% to 30.5% after reduction, indicating that the oxygen vacancy concentration and the adsorbed oxygen content on the sample surface are enhanced, promoting the electrochemical reaction process at the electrode.
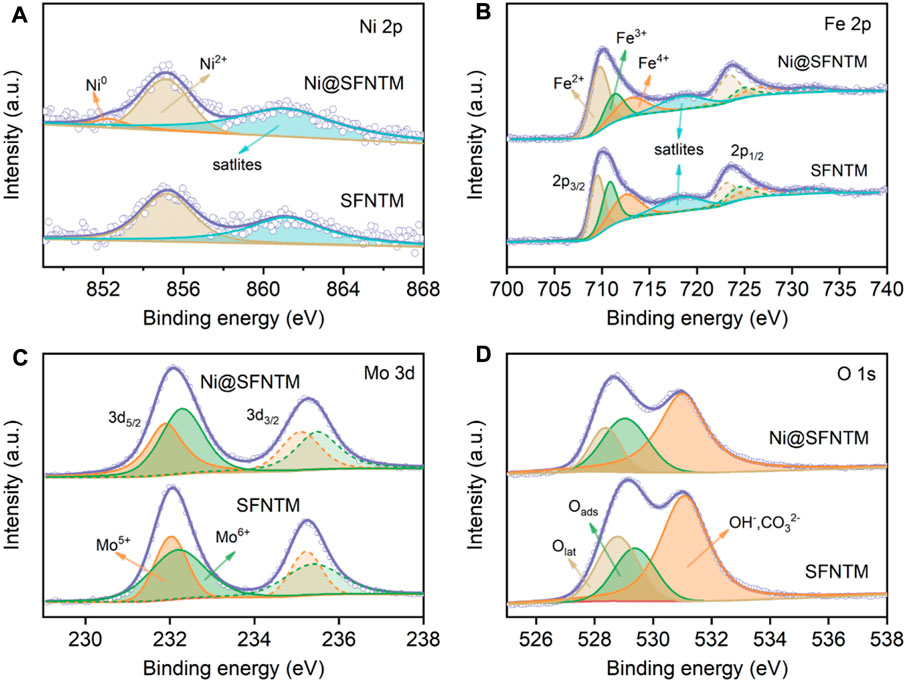
FIGURE 3. XPS characterization of SFNTM and Ni@SFNTM samples: (A) Ni 2p spectra, (B) Fe 2p spectra, (C) Mo 3 d spectra, (D) O 1s spectra.
Electron Paramagnetic Resonance (EPR) analysis was used to determine the relative peak intensities of the SFNTM and Ni@SFNTM samples, which correspond to the spin numbers of the lone electrons. Ni@SFNTM shows a higher relative peak intensity than that of SFNTM (Figure 4A), indicating that Ni@SFNTM has a higher concentration of oxygen vacancies; this result is consistent with the XPS results of the O 1s spectra (Figure 3D) (Xi et al., 2021) Moreover, the surface oxygen exchange and bulk diffusion properties of the two samples were evaluated using the ECR method, which can imply the kinetics of the surface CO2-RR and bulk diffusion in a CO2-rich atmosphere (Zhu et al., 2016; Li et al., 2019). Figure 4B shows that the oxygen exchange rate can reach equilibrium state with an increase in the CO2 concentration. The shorter the relaxation time, the faster the oxygen exchange rate. The Ni@SFNTM sample presents a shorter relaxation time than the SFNTM sample at 800°C, implying that the Ni@SFNTM sample has a faster oxygen ion exchange rate due to the increased oxygen vacancies after reduction and can speed up the oxygen transfer process. Additionally, the calculated surface exchange coefficients (Kchem) of SFNTM and Ni@SFNTM are 2.42 × 10–5 cm s−1 and 8.67 × 10–5 cm s−1, respectively. The corresponding chemical bulk diffusion coefficients (Dchem) are 2.58 × 10–6 cm2 s−1 for SFNTM and 7.85 × 10–6 cm2 s−1 for Ni@SFNTM. In SOECs, the CO2 chemisorption process on the surface of the cathode is a rate-limiting step for CO2-RR, where the adsorption capacity of CO2 is crucial for the cathode materials of SOEC (Lv et al., 2021). The CO2 adsorption properties of Ni@SFNTM and SFNTM were determined using CO2-TPD measurements (Figure 4C). Two desorption peaks at ∼400 and ∼800°C can be clearly observed in the desorption process of CO2 corresponding to the physical desorption and the chemical desorption, respectively. At low temperatures, both samples exhibit similar physical desorption processes (Ye et al., 2017). However, both the chemical desorption peak and the corresponding peak area of Ni@SFNTM are higher than those of SFNTM at high temperatures, indicating an enhanced strong binding force for CO2 adsorption caused by the increased oxygen vacancy and exsolved Ni nano particles. Furthermore, thermogravimetric relaxation was used to investigate the weight changes of the Ni@SFNTM and SFNTM samples by rapidly switching the N2 atmosphere to a CO2 atmosphere at 600°C. Figure 4D shows that the weights of the two samples rapidly increase owing to the adsorption of CO2 and reach equilibrium when the adsorption of CO2 reached saturation. The Ni@SFNTM sample has a higher weight increase (0.68 %) and shorter adsorption time, indicating that Ni@SFNTM has more oxygen vacancies and stronger CO2 chemisorption. This result is in good agreement with the XPS and CO2-TPD results.
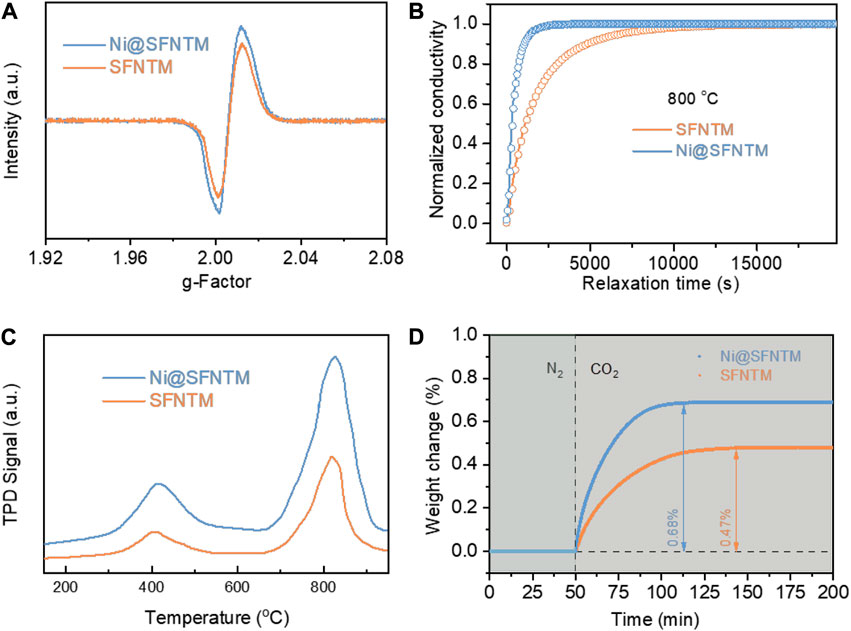
FIGURE 4. (A) EPR measurements and (B) ECR curves of SFNTM and Ni@SFNTM samples, (C) CO2-TPD curves at 800°C, tested after abrupt change of the atmosphere from 2:1 CO-CO2 to 1:1 CO-CO2, (D) Thermogravimetric relaxation curves on changing test gas from N2 to CO2 at 600°C.
In order to study the electrochemical performance and analyze the CO2 electrolysis reaction of the two cathode materials, EIS was performed on the LSGM electrolyte-supported single cells with the configuration of SFNTM/Ni@SFTNM|SDC|LSGM|LSCF under a pure CO2 atmosphere at an OCV. Figure 5A shows the polarization resistance (Rp) of a single cell with Ni@SFNTM and SFNTM as cathodes at 800°C. The Rp value of Ni@SFNTM is 0.29 Ω cm−2, which is much lower than that of SFNTM (0.48 Ω cm−2). The EIS data obtained at applied potential of 1.4 V and 800°C were analyzed, as shown in Supplementary Figure S3. Clearly, the SOEC with the Ni@SFNTM cathode significantly reduces the Rp at 1.4 V, which is consistent with the OCV. Owing to the high catalytic activity of Ni metal and enhanced oxygen vacancies, the reduced Rp improves the CO2-RR activity of Ni@SFNTM. Figure 5B shows the distribution of the relaxation time (DRT) technology used to explore the separation of the pivotal electrode reaction processes and analyze the deconvolution of the EIS data to further understand the CO2-RR electrocatalysis process (Zhang et al., 2015; Zhang et al., 2016). There are three areas in the DRT curves of the Ni@SFNTM and SFNTM samples. The peaks in the high-frequency area (HF) are primarily associated with the oxygen evolution reaction in the anode and the transportation of O2- through the interface of the electrodes and electrolyte (Zhou et al., 2018; Jiang et al., 2019). The low-frequency (LF) area is likely attributed to gas adsorption and the dissociation processes on the cathode surface (Li et al., 2017b). Additionally, the intermediate frequency (IF) is between HF and LF, and represents reactant diffusion and surface processes (Chen et al., 2018; Tian et al., 2020). Both IF and LF exhibit kinetic processes on the electrode surface. It is worth noting that the IF and LF of the Ni@SFNTM cathode significantly decreases, demonstrating that the CO2 adsorption and electrolysis reaction processes improved. The positions of the characteristic peaks related to IF and LF shift to higher frequencies, suggesting that the electrochemical reaction kinetics should be accelerated. In contrast, after the reduction treatment, the exsolved Ni metal and the increased oxygen vacancies supply more active reaction sites for CO2 adsorption and activation, while the formed metal-oxide heterostructure with strong interaction enhances the surface exchange reactions (Lee et al., 2021; Zhang L. H. et al., 2022). This occurrence indicates that the CO2-RR performance of the Ni@SFNTM cathode is enhanced. These results are in agreement with the XPS, TDP, and ECR results. Moreover, the Rp values of the two cathodes at different operating temperatures are investigated as shown in Figure 5C. The polarization resistances of both cathodes decrease with an increase in temperature, and the Rp values of the Ni@SFNTM cathode are lower than those of the SFNTM cathode at a certain temperature. Furthermore, the lower activation energy of the Ni@SFNTM cathode (Figure 5D) also confirms the improved CO2-RR kinetics and enhanced O2- transportation, primarily resulting from the heterostructure and extended reactive area (Lv et al., 2022).
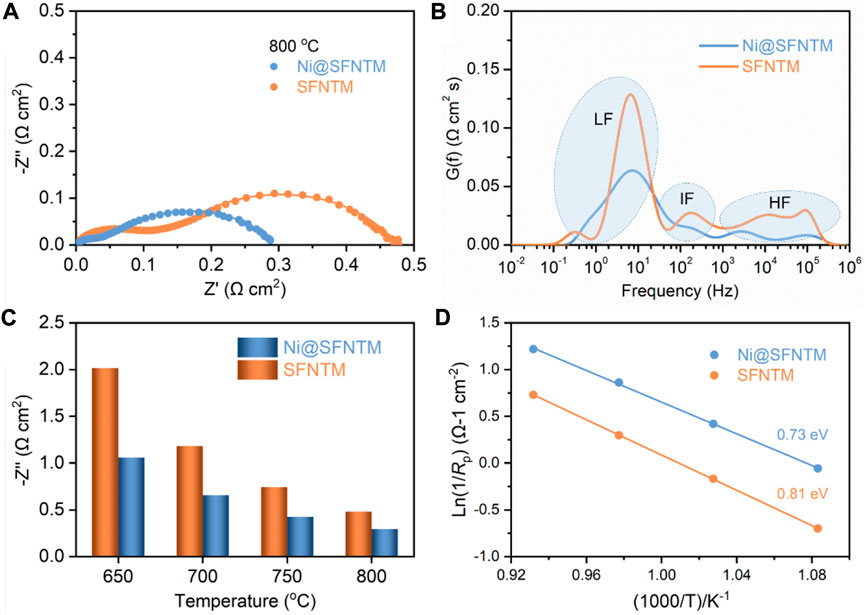
FIGURE 5. (A) EIS patterns of SFNTM and Ni@SFNTM powders at 800°C, and (B) corresponding distribution of the relaxation time (DRT) curves, (C) Impedance values of two samples from 650 to 800°C, (D) CO2-RR activation energy at 650–800°C.
Consequently, the current-voltage (I-V) curves of the single cells with SFNTM and Ni@SFNTM cathodes were measured at 650–800°C respectively, (Figures 6A,B). The CO2 electrolysis current density of the Ni@SFNTM cathode is 2.54 A cm−2 at 1.8 V and 800°C, outperforming the SFNTM cathode (1.86 A cm−2). Because of the anodes and electrolytes of the single cells are similar, the improved CO2-RR performance can be mainly ascribed to the excellent electrocatalytic activity of the Ni@SFNTM cathode. Moreover, the current densities of Ni@SFNTM are also comparable to those of other perovskite oxide cathodes (Supplementary Table S6). The short-term stability measurements of CO2-RR at various voltages are shown in Figure 6C; the Ni@SFNTM-based single cell exhibits an excellent CO2-RR performance at different electrolysis potentials. For instance, the current densities of the single cells with Ni@SFNTM cathode are 1.88 and 2.54 A cm−2 at 1.6 and 1.8 V, respectively, which is approximately 1.43 and 1.36 times than that of the single cell with the SFNTM cathode (1.31 and 1.87 A cm−2). Furthermore, gas chromatographic analysis was used to obtain the CO production rate and corresponding Faradaic efficiency by evaluating the exhaust gas collected at different applied potentials during short-time stability measurements. As shown in Figures 6D,E, both the CO production rate and Faradaic efficiency of the two cells increase with increasing applied potential; the Faradaic efficiency of both cells is close to 100%, demonstrating that CO is the primary product and there is no coke deposition during CO2 electrolysis. The CO generation rate of the Ni@SFNTM-based single cell is 12.5 ml min−1 cm−2 at 1.6 V, approximately 1.56 times that of the SFNTM-based single cell (8 ml min−1 cm−2). This further demonstrates that the formed metal-oxide heterostructure significantly enhances the CO2-RR performance. Additionally, the single cell with the Ni@SFNTM cathode exhibits excellent long-term operational stability. Figure 6F shows that a stable current density is maintained for 100 h under an applied potential of 1.4 V at 800°C in a pure CO2 atmosphere. These results indicate that Ni@SFNTM with a Ni metal and SFNTM oxide heterostructure is a potential SOEC cathode for highly efficient CO2 electrolysis.
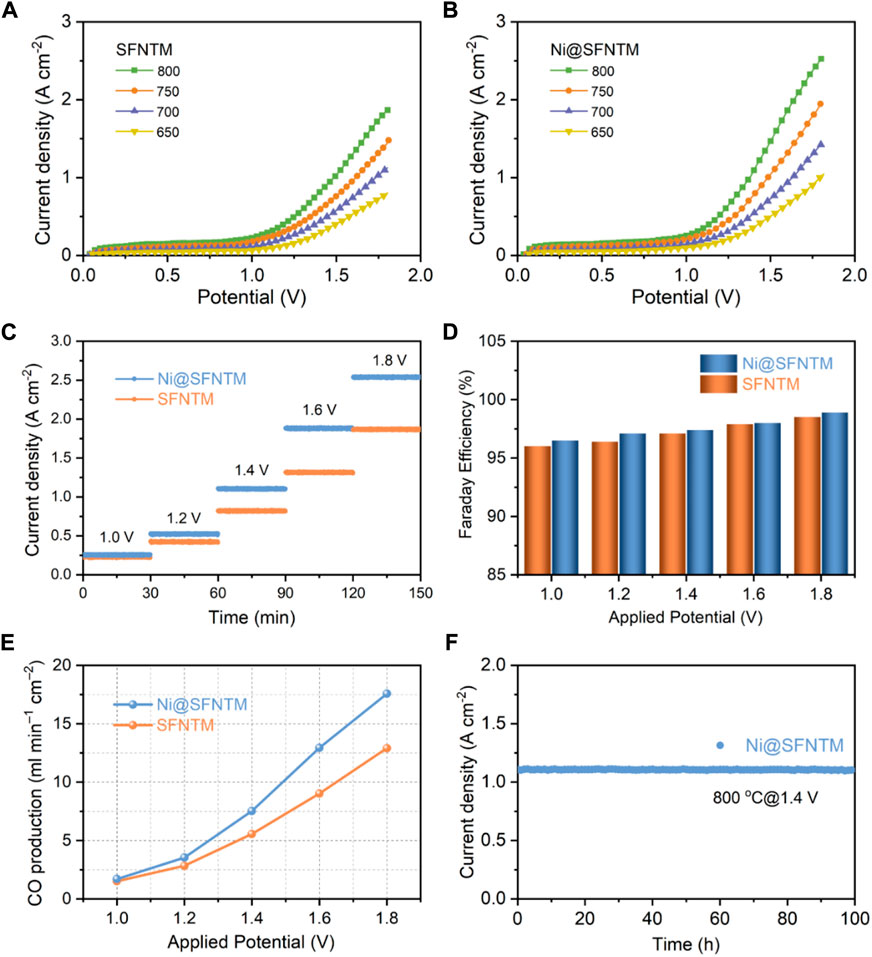
FIGURE 6. Electrochemical performance of (A) SFNTM and (B) Ni@SFNTM samples under pure CO2 atmosphere, (C) Short-term stability under different applied potentials at 800°C, (D) Comparison of Faradaic efficiency under different application potentials, and (E) corresponding CO production rate, (F) Long-term stability of single cell with Ni@SFNTM cathode under pure CO2 atmosphere at 1.4 V and 800°C.
Conclusion
In this study, Ti and Ni co-doped SFM perovskite oxides were prepared and used as cathodes for the direct electrolysis of CO2 in SOECs. A metal-oxide heterostructure was obtained after a reducing treatment in 5% H2/Ar at 800°C, providing more reactive sites for CO2-RR, enhancing the chemisorption and activation capacity of CO2, and significantly improving electrolysis performance. Moreover, the single cell with Ni@SFNTM cathode presented a large CO2 electrolysis current density of 2.54 A cm−2 at 1.8 V and 800°C, exceeding that of the cell comprising the SFNTM cathode (1.87 A cm−2 at 1.8 V); it also exhibits excellent long-term stability. This improved performance is primarily attributed to the metal-oxide heterostructure and abundant oxygen vacancies created by the reduction treatment and the strong interaction between the metal and oxide. These results indicate that Ni@SFNTM with the Ni metal and SFNTM oxide heterostructure is a potential SOEC cathode for the efficient electrolysis of CO2, and this method provides a common strategy for designing high performance electrode materials for SOECs.
Data availability statement
The original contributions presented in the study are included in the article/Supplementary Material, further inquiries can be directed to the corresponding authors.
Author contributions
SZ, main experiment conduct and data curation, manuscript writing. LZ and CX, literature survey, characterization of materials and manuscript curation. DZ and QY, project supervision, financial support, critical review, and manuscript discussion. WS, Data analysis, drawing, manuscript writing and revision. KS, supervision and funding acquisition.
Funding
The work was financially supported by the National Natural Science Foundation of China (Grant Nos 22178023 and 22078022) and Fundamental Research Funds for the Central Universities (FRF-TP-19–077A1).
Acknowledgments
We also thank financial support from the Knowledge Innovation Program of Wuhan -Basic Research (2022020801010354) and Shanxi-Zheda Institute of Advanced Materials and Chemical Engineering (2022SX-TD015).
Conflict of interest
The authors declare that the research was conducted in the absence of any commercial or financial relationships that could be construed as a potential conflict of interest.
Publisher’s note
All claims expressed in this article are solely those of the authors and do not necessarily represent those of their affiliated organizations, or those of the publisher, the editors and the reviewers. Any product that may be evaluated in this article, or claim that may be made by its manufacturer, is not guaranteed or endorsed by the publisher.
Supplementary material
The Supplementary Material for this article can be found online at: https://www.frontiersin.org/articles/10.3389/fchem.2022.1027713/full#supplementary-material
References
Armaroli, N., and Balzani, V. (2011). The legacy of fossil fuels. Chem. Asian J. 6, 768–784. doi:10.1002/asia.201000797
Broecker, W. (2018). CO2: Earth's climate driver. Geochem. Perspect. 7, 117–196. doi:10.7185/geochempersp.7.2
Chen, Y., Yoo, S., Choi, Y., Kim, J. H., Ding, Y., Pei, K., et al. (2018). A highly active, CO2-tolerant electrode for the oxygen reduction reaction. Energy Environ. Sci. 11, 2458–2466. doi:10.1039/c8ee01140k
Choi, J., Park, S., Han, H., Kim, M., Park, M., Han, J., et al. (2021). Highly efficient CO2 electrolysis to CO on Ruddlesden-Popper perovskite oxide with in situ exsolved Fe nanoparticles. J. Mat. Chem. A Mat. 9, 8740–8748. doi:10.1039/d0ta11328j
Dong, D. H., Xu, S. S., Shao, X., Hucker, L., Marin, J., Pham, T., et al. (2017). Hierarchically ordered porous Ni-based cathode-supported solid oxide electrolysis cells for stable CO2 electrolysis without safe gas. J. Mat. Chem. A Mat. 5, 24098–24102. doi:10.1039/c7ta06839e
Fu, H. C., You, F., Li, H. R., and He, L. N. (2019). CO2 capture and in situ catalytic transformation. Front. Chem. 7, 525. doi:10.3389/fchem.2019.00525
Hoegh-Guldberg, O., Jacob, D., Taylor, M., Bolanos, T. G., Bindi, M., Brown, S., et al. (2019). The human imperative of stabilizing global climate change at 1.5°C. Science 365, eaaw6974. doi:10.1126/science.aaw6974
Jiang, Y., Yang, Y., Xia, C., and Bouwmeester, H. J. (2019). Sr2Fe1.4Mn0.1Mo0.5O6−δ perovskite cathode for highly efficient CO2 electrolysis. J. Mat. Chem. A Mat. 7, 22939–22949. doi:10.1039/c9ta07689a
Karanikolos, G. N., Romanos, G. E., and Vega, L. F. (2021). Editorial: Chemical modification of adsorbents for enhanced carbon capture performance. Front. Chem. 9, 657669. doi:10.3389/fchem.2021.657669
Lee, S., Kim, M., Lee, K. T., Irvine, J. T. S., and Shin, T. H. (2021). Enhancing electrochemical CO2 reduction using Ce(Mn, Fe)O2 with La(Sr)Cr(Mn)O3 cathode for high-temperature solid oxide electrolysis cells. Adv. Energy Mat. 11, 2100339. doi:10.1002/aenm.202100339
Li, Y. H., Chen, X. R., Yang, Y., Jiang, Y. N., and Xia, C. R. (2017a). Mixed-conductor Sr2Fe1.5Mo0.5O6-δ as robust fuel electrode for pure CO2 reduction in solid oxide electrolysis cell. ACS Sustain. Chem. Eng. 5, 11403–11412. doi:10.1021/acssuschemeng.7b02511
Li, Y. H., Hu, B. B., Xia, C. R., Xu, W. Q., Lemmon, J. P., and Chen, F. L. (2017b). A novel fuel electrode enabling direct CO2 electrolysis with excellent and stable cell performance. J. Mat. Chem. A 5, 20833–20842. doi:10.1039/c7ta05750d
Li, Y. H., Li, Y., Wan, Y. H., Xie, Y., Zhu, J. F., Pan, H. B., et al. (2019). Perovskite oxyfluoride electrode enabling direct electrolyzing carbon dioxide with excellent electrochemical performances. Adv. Energy Mat. 9, 1803156. doi:10.1002/aenm.201803156
Li, Z. S., Cui, L., Luo, J. L., Li, J. H., and Sun, Y. F. (2021). Perovskite chromite with in-situ assembled Ni-Co nano-alloys: A potential bifunctional electrode catalyst for solid oxide cells. Front. Chem. 8, 595608. doi:10.3389/fchem.2020.595608
Liu, C. Y., Li, S. T., Gao, J. Q., Bian, L. Z., Hou, Y. T., Wang, L. J., et al. (2021). Enhancing CO2 catalytic adsorption on an Fe nanoparticle-decorated LaSrFeO4+δ cathode for CO2 electrolysis. ACS Appl. Mat. Interfaces 13, 8229–8238. doi:10.1021/acsami.0c18997
Liu, S. B., Liu, Q. X., and Luo, J. L. (2016). CO2-to-CO conversion on layered perovskite with in situ exsolved Co-Fe alloy nanoparticles: An active and stable cathode for solid oxide electrolysis cells. J. Mat. Chem. A Mat. 4, 17521–17528. doi:10.1039/c6ta06365a
Liu, S., Liu, Q., and Luo, J. (2016). Highly stable and efficient catalyst with in situ exsolved Fe-Ni alloy nanospheres socketed on an oxygen deficient perovskite for direct CO2 electrolysis. ACS Catal. 6, 6219–6228. doi:10.1021/acscatal.6b01555
Lv, H. F., Lin, L., Zhang, X. M., Gao, D. F., Song, Y. F., Zhou, Y. J., et al. (2019). In situ exsolved FeNi3 nanoparticles on nickel doped Sr2Fe1.5Mo0.5O6 perovskite for efficient electrochemical CO2 reduction reaction. J. Mat. Chem. A Mat. 7, 11967–11975. doi:10.1039/c9ta03065d
Lv, H. F., Lin, L., Zhang, X. M., Li, R. T., Song, Y. F., Matsumoto, H., et al. (2021). Promoting exsolution of RuFe alloy nanoparticles on Sr2Fe1.4Ru0.1Mo0.5O6-δ via repeated redox manipulations for CO2 electrolysis. Nat. Commun. 12, 5665. doi:10.1038/s41467-021-26001-8
Lv, J. W., Sun, W., Xu, C. M., Yang, X. X., Ma, M. J., Zhang, L. H., et al. (2022). Enhancing the catalytic activity and CO2 chemisorption ability of the perovskite cathode for soild oxide electrolysis cell through in situ Fe-Sn alloy nanoparticles. Sep. Purif. Technol. 294, 121127. doi:10.1016/j.seppur.2022.121127
Park, S., Kim, Y., Han, H., Chung, Y. S., Yoon, W., Choi, J., et al. (2019). In situ exsolved Co nanoparticles on Ruddlesden-Popper material as highly active catalyst for CO2 electrolysis to CO. Appl. Catal. B Environ. 248, 147–156. doi:10.1016/j.apcatb.2019.02.013
Park, S., Kim, Y., Noh, Y., Kim, T., Han, H., Yoon, W., et al. (2020). A sulfur-tolerant cathode catalyst fabricated with in situ exsolved CoNi alloy nanoparticles anchored on a Ruddlesden-Popper support for CO2 electrolysis. J. Mat. Chem. A Mat. 8, 138–148. doi:10.1039/c9ta07700f
Quadrelli, E. A., Centi, G., Duplan, J. L., and Perathoner, S. (2011). Carbon dioxide recycling: Emerging large-scale technologies with industrial potential. Chemsuschem 4, 1194–1215. doi:10.1002/cssc.201100473
Sciazko, A., Shimura, T., Komatsu, Y., and Shikazono, N. (2021). Ni-GDC and Ni-YSZ electrodes operated in solid oxide electrolysis and fuel cell modes. J. Therm. Sci. Technol. 16, JTST0013. doi:10.1299/jtst.2021jtst0013
Singh, V., Muroyama, H., Matsui, T., Hashigami, S., Inagaki, T., and Eguchi, K. (2015). Feasibility of alternative electrode materials for high temperature CO2 reduction on solid oxide electrolysis cell. J. Power Sources 293, 642–648. doi:10.1016/j.jpowsour.2015.05.088
Song, Y. F., Zhou, Z. W., Zhang, X. M., Zhou, Y. J., Gong, H. M., Lv, H. F., et al. (2018). Pure CO2 electrolysis over an Ni/ YSZ cathode in a solid oxide electrolysis cell. J. Mat. Chem. A Mat. 6, 13661–13667. doi:10.1039/c8ta02858c
Tian, Y. F., Liu, Y., Naden, A., Jia, L. C., Xu, M., Cui, W., et al. (2020). Boosting CO2 electrolysis performance via calcium-oxide-looping combined with in situ exsolved Ni-Fe nanoparticles in a symmetrical solid oxide electrolysis cell. J. Mat. Chem. A Mat. 8, 14895–14899. doi:10.1039/d0ta05518b
Wang, W. Y., Gan, L. Z., Lemmon, J. P., Chen, F. L., Irvine, J. T. S., and Xie, K. (2019). Enhanced carbon dioxide electrolysis at redox manipulated interfaces. Nat. Commun. 10, 1550. doi:10.1038/s41467-019-09568-1
Wang, Y., Liu, T., Li, M., Xia, C., Zhou, B., and Chen, F. (2016). Exsolved Fe-Ni nano-particles from Sr2Fe1.3Ni0.2Mo0.5O6 perovskite oxide as a cathode for solid oxide steam electrolysis cells. J. Mat. Chem. A Mat. 4, 14163–14169. doi:10.1039/c6ta06078a
Xi, X. A., Liu, J. W., Luo, W. Z., Fan, Y., Zhang, J. J., Luo, J. L., et al. (2021). Unraveling the enhanced kinetics of Sr2Fe1+xMo1-xO6-δ electrocatalysts for high-performance solid oxide cells. Adv. Energy Mater. 11, 2102845. doi:10.1002/aenm.202102845
Xu, C. M., Sun, W., Ren, R. Z., Yang, X. X., Ma, M. J., Qiao, J. S., et al. (2021). A highly active and carbon-tolerant anode decorated with in situ grown cobalt nano-catalyst for intermediate-temperature solid oxide fuel cells. Appl. Catal. B-Environmental 282, 119553. doi:10.1016/j.apcatb.2020.119553
Xu, C. M., Zhang, L. H., Sun, W., Ren, R. Z., Yang, X. X., Ma, M. J., et al. (2022). Co-improving the electrocatalytic performance and H2S tolerance of a Sr2Fe1.5Mo0.5O6-δ based anode for solid oxide fuel cells. J. Mat. Chem. A Mat. 10, 16280–16289. doi:10.1039/d2ta03136a
Xu, C. M., Zhen, S. Y., Ren, R. Z., Chen, H. S., Song, W. L., Wang, Z. H., et al. (2019). Cu-Doped Sr2Fe1.5Mo0.5O6-δ as a highly active cathode for solid oxide electrolytic cells. Chem. Commun. 55, 8009–8012. doi:10.1039/c9cc03455b
Yang, C. C., Tian, Y. F., Pu, J., and Chi, B. (2022). Anion fluorine-doped La0.6Sr0.4Fe0.8Ni0.2O3 perovskite cathodes with enhanced electrocatalytic activity for solid oxide electrolysis cell direct CO2 electrolysis. ACS Sustain. Chem. Eng. 10, 1047–1058. doi:10.1021/acssuschemeng.1c07576
Ye, L. T., Zhang, M. Y., Huang, P., Guo, G. C., Hong, M. C., Li, C. S., et al. (2017). Enhancing CO2 electrolysis through synergistic control of non-stoichiometry and doping to tune cathode surface structures. Nat. Commun. 8, 14785. doi:10.1038/ncomms14785
Zhang, B.-W., Zhu, M.-N., Gao, M.-R., Xi, X., Duan, N., Chen, Z., et al. (2022). Boosting the stability of perovskites with exsolved nanoparticles by B-site supplement mechanism. Nat. Commun. 13, 4618. doi:10.1038/s41467-022-32393-y
Zhang, L. H., Xu, C. M., Sun, W., Ren, R. Z., Yang, X. X., Luo, Y. Z., et al. (2022). Constructing perovskite/alkaline-earth metal composite heterostructure by infiltration to revitalize CO2 electrolysis. Sep. Purif. Technol. 298, 121475. doi:10.1016/j.seppur.2022.121475
Zhang, S. W., Zhu, K., Hu, X.y., Peng, R. R., and Xia, C. R. (2021). Antimony doping to greatly enhance the electrocatalytic performance of Sr2Fe1.5Mo0.5O6-δ perovskite as a ceramic anode for solid oxide fuel cells. J. Mater. Chem. A. 9, 24336–24347. doi:10.1039/d1ta06196h
Zhang, X. M., Liu, L., Zhao, Z., Tu, B. F., Ou, D. R., Cui, D. A., et al. (2015). Enhanced oxygen reduction activity and solid oxide fuel cell performance with a nanoparticles-loaded cathode. Nano Lett. 15, 1703–1709. doi:10.1021/nl5043566
Zhang, X. M., Wu, W. M., Zhao, Z., Tu, B. F., Ou, D. R., Cui, D. A., et al. (2016). Insight into the oxygen reduction reaction on the LSM|GDC interface of solid oxide fuel cells through impedance spectroscopy analysis. Catal. Sci. Technol. 6, 4945–4952. doi:10.1039/c5cy02232k
Zhang, Y. Q., Jacobs, G., Sparks, D. E., Dry, M. E., and Davis, B. H. (2002). CO and CO2 hydrogenation study on supported cobalt Fischer-Tropsch synthesis catalysts. Catal. Today 71, 411–418. doi:10.1016/s0920-5861(01)00468-0
Zheng, Y., Wang, J. C., Yu, B., Zhang, W. Q., Chen, J., Qiao, J. L., et al. (2017). A review of high temperature co-electrolysis of H2O and CO2 to produce sustainable fuels using solid oxide electrolysis cells (SOECs): Advanced materials and technology. Chem. Soc. Rev. 46, 1427–1463. doi:10.1039/c6cs00403b
Zhou, Y. J., Zhou, Z. W., Song, Y. F., Zhang, X. M., Guan, F., Lv, H. F., et al. (2018). Enhancing CO2 electrolysis performance with vanadium-doped perovskite cathode in solid oxide electrolysis cell. Nano Energy 50, 43–51. doi:10.1016/j.nanoen.2018.04.054
Keywords: solid oxide electrolysis cells (SOECs), double perovskite oxide, cathode, in situ exsolution, CO2 reduction reaction
Citation: Zhen S, Zhang L, Xu C, Zhang D, Yi Q, Sun W and Sun K (2022) Ti/Ni co-doped perovskite cathode with excellent catalytic activity and CO2 chemisorption ability via nanocatalysts exsolution for solid oxide electrolysis cell. Front. Chem. 10:1027713. doi: 10.3389/fchem.2022.1027713
Received: 25 August 2022; Accepted: 21 September 2022;
Published: 10 October 2022.
Edited by:
Tao Wei, Jiangsu University of Science and Technology, ChinaReviewed by:
Tian Xia, Heilongjiang University, ChinaShuiyun Shen, Shanghai Jiao Tong University, China
Xiaoliang Zhou, Southwest Petroleum University, China
Copyright © 2022 Zhen, Zhang, Xu, Zhang, Yi, Sun and Sun. This is an open-access article distributed under the terms of the Creative Commons Attribution License (CC BY). The use, distribution or reproduction in other forums is permitted, provided the original author(s) and the copyright owner(s) are credited and that the original publication in this journal is cited, in accordance with accepted academic practice. No use, distribution or reproduction is permitted which does not comply with these terms.
*Correspondence: Qun Yi, eXEyMDIxMDlAd2l0LmVkdS5jbg==; Wang Sun, c3Vud2FuZ0BiaXQuZWR1LmNu