- 1School of Chemistry and Materials Science, Jiangsu Normal University, Xuzhou, China
- 2College of Chemistry and Materials Engineering, Wenzhou University, Wenzhou, China
Four homodinuclear lanthanide complexes, Dy2 (LOEt)2(OAc)4 (1), Tb2 (LOEt)2(OAc)4 (2), Ho2(LOEt)2(OAc)4 (3), and Gd2 (LOEt)2(OAc)4 (4), have been synthesized and characterized based on a tripodal oxygen ligand Na [(η5-C5H5)Co(P(O)(OC2H5)2)3] (NaLOEt). Structural analyses show that the acetate anions bridge two symmetry-related Ln3+ ions in the μ2:η1:η1 and μ2:η1:η2 coordination patterns, and each lanthanide (III) ion owns a twisted square antiprism (SAPR) conformation. Static magnetic measurements reveal the weak intramolecular ferromagnetic interaction between dysprosium (III) ions in 1 and antiferromagnetic Ln3+···Ln3+ couplings in the other three complexes. Through the analysis of the ligand-field effect and magnetic anisotropy axis orientation, the reasons for the lack of dynamic magnetic behavior in 1 were identified.
Introduction
As novel nano-molecular magnetic materials, single-molecule magnets (SMMs), showing slow relaxation of magnetization, have attracted widespread interest in both theoretical and applied research areas because of their intriguing structures and specific physical/chemical properties (Leuenberger and Loss, 2001; Bogani and Wernsdorfer, 2008; Troiani and Affronte, 2011; Li J et al., 2021). It has been found that the introduction of paramagnetic lanthanide ions with larger magnetic anisotropy and stronger spin-orbit coupling is an effective approach to construct promising SMMs.
So far, many high-performance mononuclear lanthanide-based SMMs, also named single-ion magnets (SIMs), have been reported (Liu et al., 2018; Zhu et al., 2019; Parmar et al., 2021; Sutter et al., 2022). The studies revealed that a suitable crystal-field environment with specific symmetry around lanthanide spin centers, such as D4d (Ishikawa et al., 2003; Bala et al., 2019; Zhuo et al., 2021), D4h (Ding et al., 2021; Thomas-Hargreaves et al., 2021), D5h (Chen et al., 2016; Ding et al., 2022; Sutter et al., 2022), D6h (Canaj et al., 2019; Li et al., 2019; Zhu et al., 2021), and C∞ (Goodwin et al., 2017; Guo et al., 2017; Guo et al., 2018), usually leads to a remarkable single-ion magnetic anisotropy and slow magnetic relaxation behavior.
For Ln-SIMs, it is convenient to investigate the relationship between the ligand-field effect, uniaxial magnetic anisotropy, and magnetic relaxation processes. However, some factors, e.g., the higher coordination number and relatively low axisymmetric tendency of lanthanide ions and the common quantum tunneling of magnetization (QTM) effect in SIMs, still maintain a certain level of challenge for molecular design.
As an alternative, the research on polynuclear lanthanide-based SMMs, especially simple dinuclear lanthanide systems, provides a broader space for suppressing QTM and fine-tuning the dynamic magnetic behaviors by introducing intramolecular magnetic couplings (Morita et al., 2018; Gao et al., 2019; Goodwin, 2020; Gould et al., 2020; Meng et al., 2020; Li X et al., 2021; Xu et al., 2021).
Many reports on dinuclear Ln-SMMs have shown that the effective regulation of local symmetry around spin carriers, magnetic anisotropy axis orientation, and the strength and nature of paramagnetic Ln3+···Ln3+ magnetic interactions through choosing appropriate ligands is still the focus of current research. Therefore, four new acetate-bridged dinuclear lanthanide complexes Dy2 (LOEt)2(OAc)4 (1), Tb2 (LOEt)2(OAc)4 (2), Ho2(LOEt)2(OAc)4 (3), and Gd2 (LOEt)2(OAc)4 (4) were designed and prepared in this study by virtue of the chelation coordination feature of a tripodal oxygen ligand Na [(η5-C5H5)Co(P(O)(OC2H5)2)3] (NaLOEt) and the variable bridge modes of acetate anion (Scheme 1). Their crystal structures and magnetic properties were also significantly investigated.
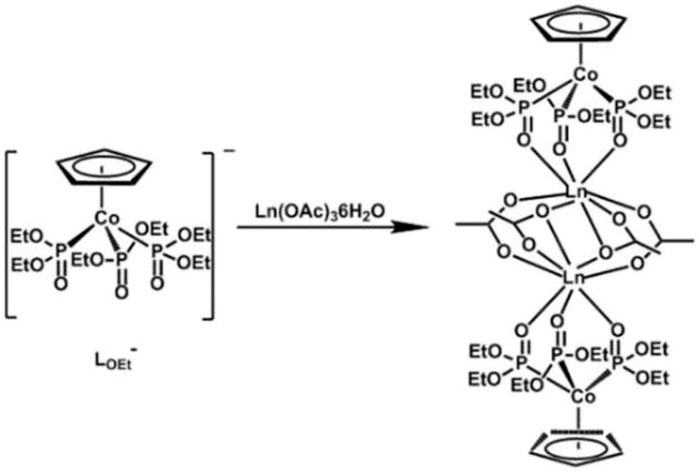
SCHEME 1. Synthetic process of target complexes Ln2 (LOEt)2(OAc)4 (Ln = Dy (1), Tb (2), Ho (3), and Gd (4)).
Experimental sections
Preparation of Dy2 (LOEt)2(OAc)4 (1)
For the preparation, 27.8 mg (0.062 mmol) Dy(OAc)3·6H2O and 35.6 mg (0.062 mmol) tripodal ligand NaLOEt were dissolved in 8 ml of methanol and 5 ml of acetone. The resultant solution was treated for 10 h at 90°C. After about 6 days, suitable yellow crystals can be produced by evaporating the clear mother solution at room temperature (yield = 52%, based on NaLOEt). Main IR data (cm−1): 2977(m), 1625(s), 1447(m), 1416(m), 1142(s), 1038(s), 932(m), 832(m), 771(m), 722(m), and 583(m). Anal. Calcd for C42H82Co2Dy2O26P6 (%): C, 30.91; H, 5.07. Found: C, 31.10; H, 5.23. UV-Vis (λmax/nm with log (ε/dm3 mol−1 cm−1)): 245(4.77) and 335(3.92).
Preparation of Tb2 (LOEt)2(OAc)4 (2)
The preparation of Tb2 (LOEt)2(OAc)4 (2) followed the same procedure as (1), using 27.5 mg (0.062 mmol) Tb(OAc)3·6H2O. Similar yellow crystals can be produced (yield = 45%, based on NaLOEt). Main IR data (cm−1): 2977(m), 1621(s), 1442(m), 1414(m), 1141(s), 1038(s), 932(m), 832(m), 771(m), 721(m), and 582(m). Anal. Calcd for C42H82Co2Tb2O26P6 (%): C, 31.05; H, 5.09. Found: C, 31.23; H, 5.26. UV-Vis (λmax/nm with log (ε/dm3 mol−1 cm−1)): 244(4.78) and 335(3.91).
Preparation of Ho2(LOEt)2(OAc)4 (3)
The preparation of Ho2(LOEt)2(OAc)4 (3) followed the same procedure as (1), using 27.9 mg (0.062 mmol) Ho(OAc)3·6H2O. Similar yellow crystals can be produced (yield = 46%, based on NaLOEt). Main IR data (cm−1): 2978(m), 1623(s), 1445(m), 1414(m), 1141(s), 1038(s), 933(m), 832(m), 770(m), 722(m), and 582(m). Anal. Calcd for C42H82Co2Ho2O26P6 (%): C, 30.82; H, 5.05. Found: C, 31.01; H, 5.25. UV-Vis (λmax/nm with log (ε/dm3 mol−1 cm−1)): 244(4.77) and 335(3.90).
Preparation of Gd2 (LOEt)2(OAc)4 (4)
The preparation of Gd2 (LOEt)2(OAc)4 (4) followed the same procedure as (1), using 27.4 mg (0.062 mmol) Gd(OAc)3·6H2O. Similar yellow crystals can be produced (yield = 48%, based on NaLOEt). Main IR data (cm−1): 2977(m), 1624(s), 1446(m), 1415(m), 1142(s), 1039(s), 932(m), 831(m), 771(m), 722(m), and 582(m). Anal. Calcd for C42H82Co2Gd2O26P6 (%): C, 31.11; H, 5.10. Found: C, 31.30; H, 5.28. UV-Vis (λmax/nm with log (ε/dm3 mol−1 cm−1)): 243(4.77) and 334(3.89).
Result and discussion
As mentioned earlier, the organometallic tripodal oxygen ligand NaLOEt is an ideal building block to encapsulate metal cores with its oxygen-based tridentate coordination sites (Gao et al., 2014; Lim et al., 2016; Van Raden et al., 2022). As a result, target lanthanide complexes were synthesized by a one-step reaction of various lanthanide acetate hydrates with NaLOEt. Satisfactory crystals for X-ray crystallography can be obtained by evaporating the mixed solution. These air-stable complexes are readily soluble in acetonitrile, acetone, dichloromethane, and methanol. Detailed characterization has been performed by IR spectra, elemental analysis, UV-Vis absorption spectra (Supplementary Figure S1), and magnetic measurements.
Purity analysis
Powder X-ray diffraction (PXRD) experiment data were measured at room temperature to verify the phase purity of crystal samples. The main experimental peaks match well with the simulated PXRD patterns according to the X-ray crystal data on the respective complexes (Figure 1), confirming the good purity of the prepared products.
Crystal structure description
These electrically neutral complexes crystallize in the triclinic crystal system (Pī space group), and each asymmetric unit contains one Ln3+ ion, one tripodal anionic ligand [LOEt]−, and two acetate anions (Figure 2 and Supplementary Figures S2–S4). Related crystallographic parameters are listed in Supplementary Table S1 with CCDC numbers 2182586 (1), 2182587 (2), 2182588 (3), and 2182589 (4). Some main bond length and bond angle data are presented in Supplementary Table S2. Because they are crystallographically isostructural, only the molecular structure of 1 is fully described as a representative. In Figure 2A, the paramagnetic Dy13+ ion is coordinated through eight oxygen atoms (O1, O2, and O3 from tripodal anionic [LOEt]−, O1_7, O1_8, O1_7A, O2_7A, and O2_8A from acetate anions, respectively). These Dy-O bond lengths range between 2.311 (2) and 2.537 (2) Å. The tripodal anionic [LOEt]− situates above the dysprosium (III) ion, and the diamagnetic cobalt (III) ion is surrounded by three phosphorus atoms and a cyclopentadienyl ring. The acetate anions use two different coordination patterns, μ2:η1:η1 (Figure 3A) and μ2:η1:η2 (Figure 3B), to bridge two symmetry-related dysprosium (III) ions with an intramolecular Dy13+···Dy1A3+ distance of 3.915 (8) Å. The continuous shape measurement (CShM) method by SHAPE analysis (Alvarez et al., 2005) was performed to determine the precise geometry of lanthanide centers (Supplementary Table S3). The eight-coordinated paramagnetic dysprosium (III) ion in 1 has a twisted square antiprism (SAPR, D4d) conformation (Figure 2B) with calculated CShM value S = 1.619. As shown in the crystal packing diagram of 1 (Figure 4), no special intermolecular interactions can be found with the shortest intermolecular distance between dysprosium (III) ions of 9.844 (3) Å.
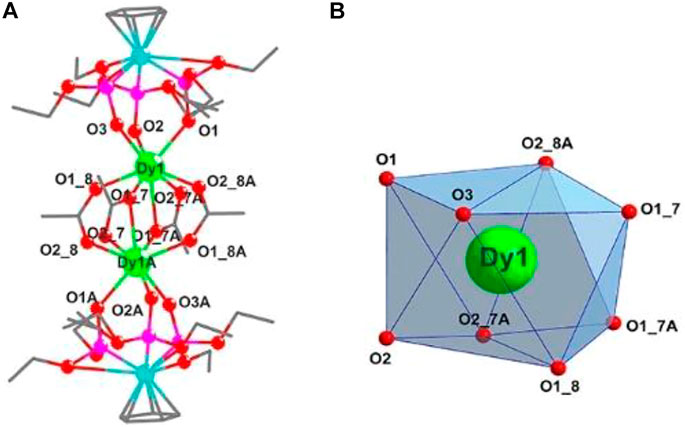
FIGURE 2. (A) Molecular structure diagram of 1 (Dy, green; Co, aqua; O, red; P, pink; and C, gray). The H atoms are omitted for clarity. (B) Coordination polyhedron of the Dy13+ ion.
Magnetism investigation
Temperature-dependent direct current (dc) magnetic susceptibility plots (χMT vs. T) are presented in Figure 5, which were collected under Hdc = 1 kOe between 2.0 and 300 K. Since the diamagnetic cobalt (III) cations in the system have no effect on magnetic properties, the measured χMT values at 300 K are 27.87 (1), 23.60 (2), 26.82 (3), and 15.51 (4) cm3 K mol−1, comparable to the theoretically calculated results for two isolated paramagnetic lanthanide (III) ions. In high-temperature regions, the χMT products of 1, 2, and 3 decrease slowly and reach the respective minimum values of 23.08 (1, 9.0 K), 11.65 (2, 2.0 K), and 6.81 (3, 2.0 K) cm3 K mol−1, usually caused by antiferromagnetic couplings between adjacent lanthanide (III) ions, and/or depopulation of Ln3+ ions excited Stark (MJ) sublevels (Lim et al., 2016; Liu et al., 2016; Li J et al., 2021). Upon lowering the temperature to 2.0 K, the χMT value of 1 rises again to a maximum of 24.40 cm3 K mol−1, indicating the existence of weak intramolecular ferromagnetic interactions between the paramagnetic dysprosium (III) centers (Gao et al., 2019; Shen et al., 2020; Li X et al., 2021; Liu et al., 2021). In 4, the χMT values are almost unchanged from 300 to 30.0 K and drop eventually to around 2.0 K to 12.64 cm3 K mol−1, suggesting the occurrence of antiferromagnetic Gd3+∙∙∙Gd3+ coupling. Subsequently, the isotropic spin Hamiltonian equation Ĥ = −2JŜGd1·ŜGd1A was applied to fit χMT vs. T data on 2 in order to reveal the nature and strength of the magnetic interaction between gadolinium (III) ions. The calculated values using the PHI program (Chilton et al., 2013a) are g = 1.98 and J = −0.025 cm−1 (the negative J value reveals weak antiferromagnetic interactions between gadolinium (III) ions).
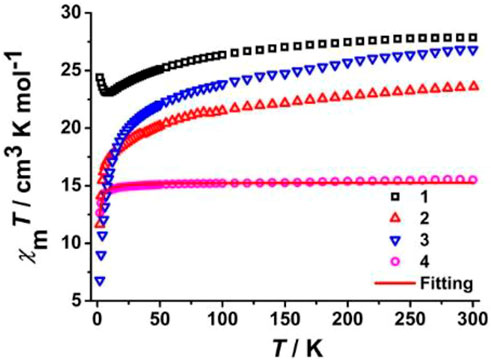
FIGURE 5. Temperature-dependent χMT plots under Hdc = 1 kOe of all prepared complexes. The red curve is the fitting result of the experimental χMT products of 4.
Magnetic field-dependent magnetizations (M vs. H) of all prepared complexes were then measured at 2.0 K under magnetic fields between 0 and 70 kOe, showing that M rises rapidly below about 15 kOe and then increases slowly in the high-magnetic field region (Figure 6). The M values at Hdc = 70 kOe are 11.80 Nβ for 1, 9.22 Nβ for 2, and 11.94 Nβ for 3. Such deviation from their respective theoretical saturation M values is ascribed to crystal field-induced low-excited states and significant magnetic anisotropy (Hutchings et al., 2014; Yang et al., 2014; Gao et al., 2019), while the maximum M value of 4 (13.84 Nβ) is consistent with the saturation value of 14.0 Nβ for two noninteracting gadolinium (III) ions.
Alternative current (ac) magnetic experiments were measured to study its dynamic magnetic behavior. Unfortunately, no obvious temperature-dependent out-of-phase (χ'') susceptibility signal peaks at the high frequency of 999 Hz were shown under Hdc = 0 Oe for 1 containing anisotropic Kramer dysprosium (III) ions (Supplementary Figure S5), possibly originating from the effect of stronger QTM. As a further investigation, the dc magnetic field of 2,500 Oe was employed. The expected χ'' signal peaks were still unable to be observed (Figure 7). We think there are two main reasons for the lack of SMM behavior in 1. On the one hand, the relatively larger distortion from the ideal D4d geometry around the dysprosium (III) center (higher calculated CShM value SSAPR = 1.619) may lead to the weaker ligand-field effect and uniaxial magnetic anisotropy. On the other hand, electrostatic calculation by means of the MAGELLAN program (Chilton et al., 2013b) was used to judge the direction of dysprosium (III) ion’s ground state (MJ = ±15/2) magnetic anisotropy axis (Figure 8). The result shows that the two magnetic axes are parallel to each other in a centrosymmetric molecule, and the angle between the magnetic axis of the Dy13+ ion and the unit vector linking two dysprosium (III) ions (Dy13+ and Dy1A3+) is 86.6°. Furthermore, as an important structural parameter affecting the ligand field strength, the Dy1-O2 bond length is 2.311 (2) Å, which is the shortest among those other Dy-O bond lengths in the twisted SAPR polyhedron. The magnetic axis of the Dy13+ ion is aligned along the shortest Dy1-O2 bond with an included angle of 58.4°. The above-mentioned two large angular deviations confirm that such a weak ligand field in this system is not conductive to activating the magnetic relaxation process.
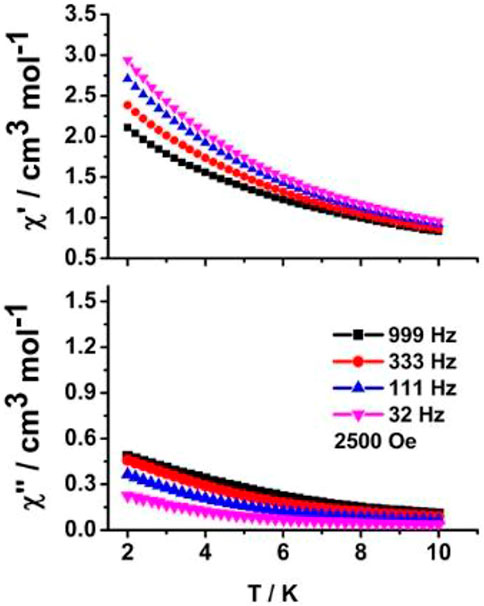
FIGURE 7. Temperature-dependent in-phase (χ′) (up) and out-of-phase (χ'') (down) ac susceptibility curves under Hdc = 2,500 Oe of 1.
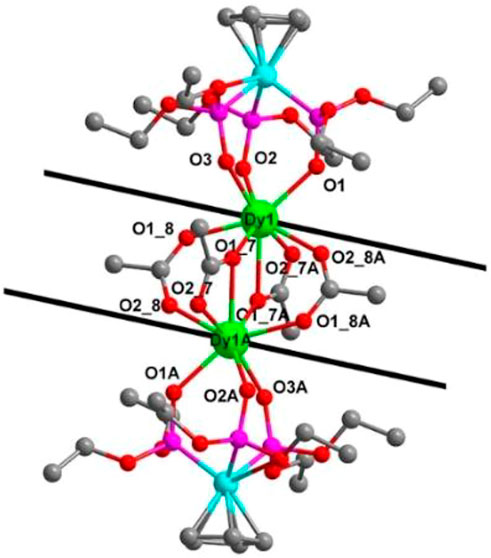
FIGURE 8. Ground state (MJ = ±15/2) magnetic anisotropy axes’ direction of dysprosium (III) ion in 1.
For complexes 2 and 3 containing anisotropic non-Kramer lanthanide (III) ions, the χ'' signals were also very weak at the frequency of 999 Hz under Hdc = 0 Oe and Hdc = 2,500 Oe, respectively (Supplementary Figures S6, S7), which is raised by their fast magnetization relaxation behavior in such a weak ligand field environment.
Conclusion
In this work, we reported four acetate-bridged homodinuclear lanthanide complexes based on a tripodal oxygen ligand NaLOEt. Structural analyses show acetate anions bridge two symmetry-related Ln3+ ions in the μ2:η1:η1 and μ2:η1:η2 coordination patterns, and each lanthanide ion owns a twisted SAPR conformation. Magnetic analyses reveal the weak intramolecular ferromagnetic interaction between dysprosium (III) ions in 1 and antiferromagnetic Ln3+···Ln3+ coupling in the other complexes. The weaker ligand-field effect caused by the larger distorted geometry and the deviation of the magnetic anisotropy axis orientation with a specific lanthanide–ligand coordination bond leads to the lack of SMM behavior.
Although the expected SMM behaviors could not be found in this system, these well-known factors, including the effective suppression of QTM, regulation of Ln3+···Ln3+ magnetic interactions, and construction of reasonable crystal field symmetry, still remain important for affecting the slow magnetic relaxation behaviors of SMMs. Further efforts to design, synthesize, and study novel molecular magnetic materials are in progress in our group.
Data availability statement
The datasets presented in this study can be found in online repositories. The names of the repository/repositories and accession number(s) can be found in the article/Supplementary Material.
Author contributions
Idea and methodology were conceived by YS, Y-JJ, and FG. Synthesis and crystals grown were performed by YS, Y-JJ, Z-HC, and R-CL. General characterization and crystal structural analysis were performed by YS, Y-JJ, Z-HC, J-YG, and FG. Magnetic testing and data analysis were performed by YS, J-YG, and FG. The manuscript was drafted and edited by YS, Y-JJ, J-YG, and FG. All authors have given approval to the manuscript.
Funding
This work was supported by the National Natural Science Foundation of China (No. 21401085) and the Priority Academic Program Development (PAPD) of Jiangsu Higher Education Institutions.
Conflict of interest
The authors declare that the research was conducted in the absence of any commercial or financial relationships that could be construed as a potential conflict of interest.
Publisher’s note
All claims expressed in this article are solely those of the authors and do not necessarily represent those of their affiliated organizations, or those of the publisher, the editors, and the reviewers. Any product that may be evaluated in this article, or claim that may be made by its manufacturer, is not guaranteed or endorsed by the publisher.
Supplementary material
The Supplementary Material for this article can be found online at: https://www.frontiersin.org/articles/10.3389/fchem.2022.1021358/full#supplementary-material
References
Alvarez, S., Alemany, P., Casanova, D., Cirera, J., Llunell, M., and Avnir, D. (2005). Shape maps and polyhedral interconversion paths in transition metal chemistry. Coord. Chem. Rev. 249 (17-18), 1693–1708. doi:10.1016/j.ccr.2005.03.031
Bala, S., Huang, G. Z., Ruan, Z. Y., Wu, S. G., Liu, Y., Wang, L. F., et al. (2019). A square antiprism dysprosium single-ion magnet with an energy barrier over 900 K. Chem. Commun. 55 (67), 9939–9942. doi:10.1039/c9cc05135j
Bogani, L., and Wernsdorfer, W. (2008). Molecular spintronics using single-molecule magnets. Nat. Mat. 7 (3), 179–186. doi:10.1038/nmat2133
Canaj, A. B., Dey, S., Martí, E. R., Wilson, C., Rajaraman, G., and Murrie, M. (2019). Insight into D6h symmetry: Targeting strong axiality in stable dysprosium(III) hexagonal bipyramidal single-ion magnets. Angew. Chem. Int. Ed. 58 (40), 14146–14151. doi:10.1002/anie.201907686
Chen, Y. C., Liu, J. L., Ungur, L., Liu, J., Li, Q. W., Wang, L. F., et al. (2016). Symmetry-supported magnetic blocking at 20 K in pentagonal bipyramidal Dy(III) single-ion magnets. J. Am. Chem. Soc. 138 (8), 2829–2837. doi:10.1021/jacs.5b13584
Chilton, N. F., Anderson, R. P., Turner, L. D., Soncini, A., and Murray, K. S. (2013a). Phi: A powerful new program for the analysis of anisotropic monomeric and exchange-coupled polynuclear d- and f-block complexes. J. Comput. Chem. 34 (13), 1164–1175. doi:10.1002/jcc.23234
Chilton, N. F., Collison, D., McInnes, E. J. L., Winpenny, R. E. P., and Soncini, A. (2013b). An electrostatic model for the determination of magnetic anisotropy in dysprosium complexes. Nat. Commun. 4, 2551. doi:10.1038/ncomms3551
Ding, X., Luo, Q., Zhai, Y., Zhang, X., Lv, Y., Zhang, X., et al. (2022). Rigid dysprosium(III) single-molecule magnets exhibit preserved superparamagnetism in solution. Chin. J. Chem. 40 (5), 563–570. doi:10.1002/cjoc.202100722
Ding, X. L., Zhai, Y. Q., Han, T., Chen, W. P., Ding, Y. S., and Zheng, Y. Z. (2021). A local D4h symmetric dysprosium(III) single-molecule magnet with an energy barrier exceeding 2000 K. Chem. Eur. J. 27 (8), 2623–2627. doi:10.1002/chem.202003931
Gao, F., Cui, L., Song, Y., Li, Y. Z., and Zuo, J. L. (2014). Calix[4]arene-supported mononuclear lanthanide single-molecule magnet. Inorg. Chem. 53 (1), 562–567. doi:10.1021/ic4026624
Gao, F., Wang, L., Zhu, G. Z., Liu, Y. H., Yang, H., Li, X., et al. (2019). Controllable syntheses and magnetic properties of novel homoleptic triple-decker lanthanide complexes. Dalton Trans. 48 (35), 13360–13368. doi:10.1039/c9dt02565k
Goodwin, C. A. P. (2020). Blocking like it's hot: A synthetic chemists' path to high-temperature lanthanide single molecule magnets. Dalton Trans. 49 (41), 14320–14337. doi:10.1039/d0dt01904f
Goodwin, C. A. P., Ortu, F., Reta, D., Chilton, N. F., and Mills, D. P. (2017). Molecular magnetic hysteresis at 60 kelvin in dysprosocenium. Nature 548 (7668), 439–442. doi:10.1038/nature23447
Gould, C. A., Mu, E., Vieru, V., Darago, L. E., Chakarawet, K., Gonzalez, M. I., et al. (2020). Substituent effects on exchange coupling and magnetic relaxation in 2, 2'-bipyrimidine radical-bridged dilanthanide complexes. J. Am. Chem. Soc. 142 (50), 21197–21209. doi:10.1021/jacs.0c10612
Guo, F. S., Day, B. M., Chen, Y. C., Tong, M. L., Mansikkamäki, A., and Layfield, R. A. (2017). A dysprosium metallocene single-molecule magnet functioning at the axial limit. Angew. Chem. Int. Ed. 56 (38), 11445–11449. doi:10.1002/anie.201705426
Guo, F. S., Day, B. M., Chen, Y. C., Tong, M. L., Mansikkamäki, A., and Layfield, R. A. (2018). Magnetic hysteresis up to 80 kelvin in a dysprosium metallocene single-molecule magnet. Science 362 (6421), 1400–1403. doi:10.1126/science.aav0652
Hutchings, A. J., Habib, F., Holmberg, R. J., Korobkov, I., and Murugesu, M. (2014). Structural rearrangement through lanthanide contraction in dinuclear complexes. Inorg. Chem. 53 (4), 2102–2112. doi:10.1021/ic402682r
Ishikawa, N., Sugita, M., Ishikawa, T., Koshihara, S., and Kaizu, Y. (2003). Lanthanide double-decker complexes functioning as magnets at the single-molecular level. J. Am. Chem. Soc. 125 (29), 8694–8695. doi:10.1021/ja029629n
Leuenberger, M. N., and Loss, D. (2001). Quantum computing in molecular magnets. Nature 410 (6830), 789–793. doi:10.1038/35071024
Li, J., Xiong, S.-J., Li, C., Jin, B., Zhang, Y.-Q., Jiang, S.-D., et al. (2021). Manipulation of molecular qubits by isotope effect on spin dynamics. CCS Chem. 3 (9), 2548–2556. doi:10.31635/ccschem.020.202000384
Li, X., Liu, Y. H., Zhu, G. Z., Yang, F. L., and Gao, F. (2021). Successive syntheses and magnetic properties of homodinuclear lanthanide macrocyclic complexes. Dalton Trans. 50 (35), 12215–12225. doi:10.1039/d1dt01514a
Li, Z. H., Zhai, Y. Q., Chen, W. P., Ding, Y. S., and Zheng, Y. Z. (2019). Air-stable hexagonal bipyramidal dysprosium(III) single-ion magnets with nearly perfect D6h local symmetry. Chem. Eur. J. 25 (71), 16219–16224. doi:10.1002/chem.201904325
Lim, K. S., Baldoví, J. J., Lee, W. R., Song, J. H., Yoon, S. W., Suh, B. J., et al. (2016). Switching of slow magnetic relaxation dynamics in mononuclear dysprosium(III) compounds with charge density. Inorg. Chem. 55 (11), 5398–5404. doi:10.1021/acs.inorgchem.6b00410
Liu, C. M., Sun, R., Wang, B. W., Wu, F., Hao, X., and Shen, Z. (2021). Homochiral ferromagnetic coupling Dy2 single-molecule magnets with strong magneto-optical faraday effects at room temperature. Inorg. Chem. 60 (16), 12039–12048. doi:10.1021/acs.inorgchem.1c01218
Liu, J., Chen, Y. C., Liu, J. L., Vieru, V., Ungur, L., Jia, J. H., et al. (2016). A stable pentagonal bipyramidal Dy(III) single-ion magnet with a record magnetization reversal barrier over 1000 K. J. Am. Chem. Soc. 138 (16), 5441–5450. doi:10.1021/jacs.6b02638
Liu, J. L., Chen, Y. C., and Tong, M. L. (2018). Symmetry strategies for high performance lanthanide-based single-molecule magnets. Chem. Soc. Rev. 47 (7), 2431–2453. doi:10.1039/c7cs00266a
Meng, Y. S., Qiao, Y. S., Yang, M. W., Xiong, J., Liu, T., Zhang, Y. Q., et al. (2020). Weak exchange coupling effects leading to fast magnetic relaxations in a trinuclear dysprosium single-molecule magnet. Inorg. Chem. Front. 7 (2), 447–454. doi:10.1039/c9qi01252d
Morita, T., Damjanovic, M., Katoh, K., Kitagawa, Y., Yasuda, N., Lan, Y., et al. (2018). Comparison of the magnetic anisotropy and spin relaxation phenomenon of dinuclear terbium(III) phthalocyaninato single-molecule magnets using the geometric spin arrangement. J. Am. Chem. Soc. 140 (8), 2995–3007. doi:10.1021/jacs.7b12667
Parmar, V. S., Mills, D. P., and Winpenny, R. E. P. (2021). Mononuclear dysprosium alkoxide and aryloxide single-molecule magnets. Chem. Eur. J. 27 (28), 7625–7645. doi:10.1002/chem.202100085
Shen, F. X., Pramanik, K., Brandao, P., Zhang, Y. Q., Jana, N. C., Wang, X. Y., et al. (2020). Macrocycle supported dimetallic lanthanide complexes with slow magnetic relaxation in Dy2 analogues. Dalton Trans. 49 (40), 14169–14179. doi:10.1039/d0dt02778b
Sutter, J. P., Béreau, V., Jubault, V., Bretosh, K., Pichon, C., and Duhayon, C. (2022). Magnetic anisotropy of transition metal and lanthanide ions in pentagonal bipyramidal geometry. Chem. Soc. Rev. 51 (8), 3280–3313. doi:10.1039/d2cs00028h
Thomas-Hargreaves, L. R., Hunger, D., Kern, M., Wooles, A. J., van Slageren, J., Chilton, N. F., et al. (2021). Insights into D4h@metal-symmetry single-molecule magnetism: The case of a dysprosium-bis(boryloxide) complex. Chem. Commun. 57 (6), 733–736. doi:10.1039/d0cc07446b
Troiani, F., and Affronte, M. (2011). Molecular spins for quantum information technologies. Chem. Soc. Rev. 40 (6), 3119–3129. doi:10.1039/c0cs00158a
Van Raden, J. M., Alexandropoulos, D. I., Slota, M., Sopp, S., Matsuno, T., Thompson, A. L., et al. (2022). Singly and triply linked magnetic porphyrin lanthanide arrays. J. Am. Chem. Soc. 144 (19), 8693–8706. doi:10.1021/jacs.2c02084
Xu, S. M., An, Z. W., Zhang, W., Zhang, Y. Q., and Yao, M. X. (2021). Ligand field and anion-driven structures and magnetic properties of dysprosium complexes. CrystEngComm 23 (15), 2825–2834. doi:10.1039/d1ce00115a
Yang, F., Zhou, Q., Zeng, G., Li, G., Gao, L., Shi, Z., et al. (2014). Anion effects on the structures and magnetic properties of binuclear lanthanide single-molecule magnets. Dalton Trans. 43 (3), 1238–1245. doi:10.1039/c3dt52634h
Zhu, Z., Guo, M., Li, X. L., and Tang, J. (2019). Molecular magnetism of lanthanide: Advances and perspectives. Coord. Chem. Rev. 378, 350–364. doi:10.1016/j.ccr.2017.10.030
Zhu, Z., Zhao, C., Feng, T., Liu, X., Ying, X., Li, X. L., et al. (2021). Air-stable chiral single-molecule magnets with record anisotropy barrier exceeding 1800 K. J. Am. Chem. Soc. 143 (27), 10077–10082. doi:10.1021/jacs.1c05279
Zhuo, W., Tian, Y. M., Chen, P., Sun, W. B., Wang, B. W., and Gao, S. (2021). A series of counter cation-dependent tetra β-diketonate mononuclear lanthanide(III) single-molecule magnets and immobilization on pre-functionalised gan substrates by anion exchange reaction. J. Mat. Chem. C 9 (21), 6911–6922. doi:10.1039/d1tc01263k
Keywords: lanthanide complexes, ligand-field effect, single-molecule magnets, magnetic properties, magnetic interactions
Citation: Sheng Y, Jiang Y-J, Cheng Z-H, Liu R-C, Ge J-Y and Gao F (2022) Syntheses, structures, and magnetic properties of acetate-bridged lanthanide complexes based on a tripodal oxygen ligand. Front. Chem. 10:1021358. doi: 10.3389/fchem.2022.1021358
Received: 17 August 2022; Accepted: 05 September 2022;
Published: 19 September 2022.
Edited by:
Zhao-Bo Hu, Jiangxi University of Science and Technology, ChinaReviewed by:
Xing-Cai Huang, Yancheng Teachers University, ChinaJing Ru, Liaocheng University, China
Copyright © 2022 Sheng, Jiang, Cheng, Liu, Ge and Gao. This is an open-access article distributed under the terms of the Creative Commons Attribution License (CC BY). The use, distribution or reproduction in other forums is permitted, provided the original author(s) and the copyright owner(s) are credited and that the original publication in this journal is cited, in accordance with accepted academic practice. No use, distribution or reproduction is permitted which does not comply with these terms.
*Correspondence: Feng Gao, Z2YyMDE2QGpzbnUuZWR1LmNu; Jing-Yuan Ge, Z2VqaW5neXVhbjkwQDEyNi5jb20=