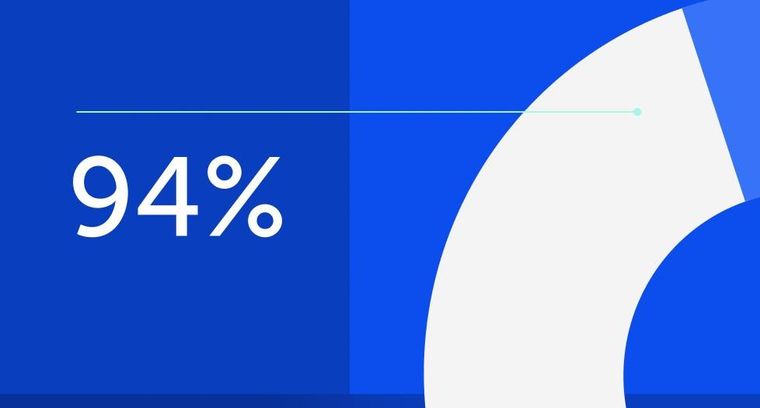
94% of researchers rate our articles as excellent or good
Learn more about the work of our research integrity team to safeguard the quality of each article we publish.
Find out more
ORIGINAL RESEARCH article
Front. Chem., 30 September 2022
Sec. Nanoscience
Volume 10 - 2022 | https://doi.org/10.3389/fchem.2022.1020538
This article is part of the Research Topic2D Materials for Electrochemical /BiosensingView all 5 articles
Abstract: A mixed-dimensional V2CTx/Ti3C2Tx composite interlayer was successfully prepared to tackle severe polysulfide (LiPS) shuttling and sluggish sulfur redox kinetics for high-performance lithium–sulfur batteries. In the unique nanoarchitecture, two-dimensional Ti3C2Tx nanosheets served as a stable skeleton with superb electronic conductivity, good mechanical strength, and high polysulfide adsorption, whereas one-dimensional V2CTx nanorods played a crucial role in chemisorbing LiPSs and catalyzing the conversion of LiPSs due to their high polarity and electrocatalysis. With the synergistic effect of V2CTx and Ti3C2Tx composite nanostructures, the cells with the mixed-dimensional V2CTx/Ti3C2Tx composite interlayer showed an impressive long-term cycling stability and small capacity decay rate of 0.062% per cycle over 600 cycles at 1 C and exhibited an outstanding rate capability of 935.3 mAh·g−1 at 2 C.
Lithium–sulfur batteries (LSBs) have been regarded as one of the most promising next-generation high-energy storage devices. This is because of their advantages of possessing high energy density (2,600 Wh·kg−1) and being low cost and environmentally friendly (Chung et al., 2018; Ye et al., 2020; Xia et al., 2021). However, the notorious shuttle effect of lithium polysulfides (LiPSs) leads to the loss of active materials, the sluggish LiPS redox kinetics, and the inferior cycling performance. These could limit the specific capacity and cycling lifetime of LSBs (Chen et al., 2019; Tian et al., 2020).
It is important that the severe shuttle effect and sluggish LiPS redox kinetics are overcome to obtain high electrochemical performance of LSBs. Among the effective strategies, the high-performance interlayers between the cathode and separator are proposed. Among the various materials, the carbon-based materials are extensively applied to prepare the interlayer because they have excellent electrical conductivity (Ma et al., 2020; Wei et al., 2020; Yu et al., 2020). However, the weak interaction between nonpolar carbon materials and LiPSs is difficult to block the diffusion of LiPSs to Li metal anode. Therefore, some polar materials, such as metal oxides (Guo et al., 2019; Li et al., 2020), sulfides (Paolella et al., 2018; Yao et al., 2018), nitrides (Yao et al., 2020), and metal–organic frameworks (Wu et al., 2019), are used to prepare interlayers to further chemically anchor LiPSs. Although they can well immobilize the LiPSs and hinder the shuttle effect, their poor electrical conductivity slows down the multistep redox reactions of sulfur (S) species. Recent research studies showed that a catalyst could make the conversion of LiPSs become fast and shorten the residence time of LiPSs in an electrolyte (Song et al., 2019; Zhang et al., 2020). Therefore, if the incorporated interlayers have an excellent electrical conductivity and could anchor the LiPSs as well as catalyze the LiPS conversion, the slow LiPS redox kinetics and the notorious shuttle effect of the LiPSs could be effectively resolved. Thus, the high-performance interlayer was constructed with a rational design. As a representative, post-graphene two-dimensional (2D) black phosphorus (BP) (Hu et al., 2020) and MXenes (Naguib et al., 2011; Gao et al., 2020; Gao et al., 2021a; Gao et al., 2021b; Zhang et al., 2021; Gao et al., 2022a; Gao et al., 2022b; Cao et al., 2022) have attracted tremendous attention since 2011, due to the layered structure and excellent physicochemical properties. Ti3C2Tx, a typical MXene material, has been widely used as interlayer materials in LSBs (Ghidiu et al., 2014; Liang et al., 2015; Song et al., 2020). Compared with the carbon-/metal-based materials, Ti3C2Tx possesses good conductivity for electron transport and high mechanical properties for structure stability. Nevertheless, 2D Ti3C2Tx nanosheets tend to aggregate, which will lead to a decrease in active sites for trapping and catalyzing LiPSs, and slow down the ion transport as well (Qiu et al., 2020). One-dimensional (1D) V2CTx nanostructures has been recently explored, which possesses the advantages of strong adsorption to LiPSs and catalytic properties (Wu et al., 2020; Zhang et al., 2022). Therefore, mixed-dimensional V2CTx/Ti3C2Tx nanostructures together as interlayers will achieve high electrochemical performance for LSBs.
Herein, we construct a mixed-dimensional V2CTx/Ti3C2Tx composite interlayer on separators for LSBs. It can anchor LiPSs by strong chemisorption and accelerate their redox kinetics under the existence of V2CTx catalyst. Based on the unique structure, the LSBs achieve an excellent rate capacity of 935.3 mAh·g−1 at 2 C. Also, a low-capacity rate decay of 0.062% is obtained after 600 cycles at 1 C. These results illustrate that the incorporation of the multifunctional interlayer is a direct and effective method to achieve high electrochemical performance for LSBs.
One-dimensional V2CTx nanorods and two-dimensional Ti3C2Tx nanosheets were synthesized based on the previous reports (Qiu et al., 2020; Zhang et al., 2022). The suspensions of 1D V2CTx nanorods and 2D Ti3C2Tx nanosheets were mixed at a mass ratio of 2:8. Then, saturated LiCl solution was added to the resultant mixed solution and stirred, which led to the mixed V2CTx/Ti3C2Tx nanostructures settled at the bottom of the container by negatively charge-induced self-assembly (Ghidiu et al., 2014; Naguib et al., 2015). Then, the sediment was washed to remove needless salt. Finally, the V2CTx/Ti3C2Tx powder was obtained by vacuum-drying. Subsequently, V2CTx/Ti3C2Tx and PVDF were dissolved in NMP solution at a mass ratio of 9:1, and then the mixed solution was filtered on a Celgard separator (PP) under vacuum. The obtained thin film is called the mixed-dimensional V2CTx/Ti3C2Tx composite interlayer (the corresponding separator is labelled as V2CTx/Ti3C2Tx-PP). The V2CTx and Ti3C2Tx interlayers were prepared using the same procedure. The corresponding separators are labelled as V2CTx-PP and Ti3C2Tx-PP, respectively.
Li2S6 solution of 0.01 M was prepared by the chemical reaction of Li2S and S at a molar ratio of 1:5 in a 1, 3-dioxolane (DOL) and 1, 2-dimethoxyethane (DME) mixture (1:1 v/v) at 60°C. A volume of 12 mg of Ti3C2Tx and V2CTx/Ti3C2Tx was added into 1 ml of Li2S6 solution, and then, the solutions were rested for adsorption.
Ti3C2Tx and V2CTx/Ti3C2Tx (w/w 2:8) were dissolved in alcohol solution. The resulting solutions were dripped onto the carbon paper (CP) with a diameter of 15 mm (labelled as Ti3C2Tx-CP and V2CTx/Ti3C2Tx-CP). Both mass loadings were ∼0.50 mg·cm−2. The 2025-type coin cells with two Ti3C2Tx-CP or V2CTx/Ti3C2Tx-CP electrodes as the anode and cathode were assembled, using PP and 0.1 M of Li2S6 as the electrolyte.
First, 0.25 M of Li2S8 solution was obtained by dissolving S and Li2S at a molar ratio of 7:1 in a tetraglyme solvent at 60°C overnight. Following this, Ti3C2Tx and V2CTx/Ti3C2Tx were dissolved in alcohol solution to obtain uniform suspensions by ultrasound, which were dripped on CPs (10 mm diameter) and dried at 60°C to obtain electrodes. The coin cell was composed of Ti3C2Tx-CP or V2CTx/Ti3C2Tx-CP cathode, lithium anode, and PP. The Li2S8 electrolyte of 0.25 M was used on the cathode side, and the standard electrolyte was used on the anode side. Then, the cells were galvanostatically discharged to 2.06 V at 0.112 mA and then kept at 2.05 V. Also, Li2S nucleated and grew until the current of 10−2 A was reached.
The morphology of mixed-dimensional V2CTx/Ti3C2Tx composite interlayers were observed using scanning electron microscopy (SEM) (SU70, Japan). The X-ray diffraction (XRD) measurement was recorded using a Rigaku D/max2600 X-ray diffractometer. The cathode is a mixture of Ketjen black/sulfur, super-P, and PVDF at a mass ratio of 8:1:1. The diameter of the cathode was about 13 mm, and the loading of S was ∼1 mg·cm−2. The coin cells were assembled with KB/S cathode, Li anode, and PP with Ti3C2Tx-PP, V2CTx/Ti3C2Tx-PP, or V2CTx-PP, respectively. The electrolyte consisted of 1.0 M lithium bis-trifluoromethane sulfonimide (LiTFSI), 2% LiNO3 additives, and a mixture of DOL/DME (volume ratio = 1:1). The galvanostatic charge–discharge (GCD) profile of the assembled cells was tested at 0.5 C in the voltage range of 1.7–2.6 V using the LAND cell testing system. The electrochemical impedance spectra (EIS) and cyclic voltammograms (CV) were carried out using an electrochemical workstation (VMP3). X-ray photoelectron spectroscopy (XPS) was conducted by applying ESCALAB 250XI.
SEM images of 1D V2CTx nanorods and 2D Ti3C2Tx nanosheets are shown in Figures 1A,B. The length of V2CTx nanorods is about 230 nm, while the diameter is tens of nanometers. Also, the size of irregular 2D Ti3C2Tx nanosheets is a few micrometers. Furthermore, the top-view SEM image of the mixed-dimensional V2CTx/Ti3C2Tx composite interlayer is presented in Figure 1C. The trace amount of V2CTx nanorods can be seen on the top surface of the composite interlayer. On the other hand, the cross-sectional SEM image of the V2CTx/Ti3C2Tx composite interlayer shows that V2CTx and Ti3C2Tx are superimposed on each other, forming a well-arranged layered stacking structure (Figure 1D). XRD of the V2CTx/Ti3C2Tx composite interlayer was carried out to characterize the crystalline structure, as shown in Figure 1E. The two diffraction peaks at 6.1 and 7.45o correspond to the (002) crystal plane of 2D Ti3C2Tx nanosheets and 1D V2CTx nanorods, respectively (Li et al., 2017; Zhang et al., 2022). In contrast, the (002) diffraction peak intensity of V2CTx is weaker, due to the low content of V2CTx. The XRD pattern proves that V2CTx and Ti3C2Tx were successfully synthesized. The composition of the V2CTx/Ti3C2Tx composite interlayer was further confirmed by XPS analysis (Li et al., 2017; Zhang et al., 2022). A high-resolution XPS spectrum of C 1s in the V2CTx/Ti3C2Tx composite interlayer is shown in Figure 1F. C-V and C-Ti bonds were observed. Meanwhile, the corresponding V-C and Ti-C bonds can also be found in the high-resolution XPS spectra of V 2p and Ti 2p in V2CTx and Ti3C2Tx, respectively, further proving that the composite interlayer is composed of V2CTx and Ti3C2Tx (Figures 1G,H). The LiPS adsorption behavior of V2CTx/Ti3C2Tx composite materials was surveyed by visualized adsorption tests, as shown in Figures 1I,J. Ti3C2Tx was used as the control sample. Figure 1I shows the initial states of different samples placed in Li2S6 solutions. In Figure 1J, the solvent colors with both Ti3C2Tx and V2CTx/Ti3C2Tx composite materials undergo a significant change after resting for 5 h. The solution including Ti3C2Tx materials changes only lighter in color. However, the solution including V2CTx/Ti3C2Tx composite materials become almost colorless. These findings demonstrate the strong chemical adsorption of V2CTx/Ti3C2Tx composite materials to LiPSs compared with Ti3C2Tx.
FIGURE 1. SEM images of (A) V2CTx nanorods, (B) Ti3C2Tx nanosheets, and (C) V2CTx/Ti3C2Tx composite interlayer. (D) Cross-sectional SEM image and (E) XRD pattern of the V2CTx/Ti3C2Tx composite interlayer. High-resolution XPS spectra of (F) C1s, (G) V 2p, and (H) Ti 2p of the V2CTx/Ti3C2Tx composite interlayer. Visualized experiment of Li2S6: (I) initial states and (J) final states after 5 h.
The mixed-dimensional interlayer with more active sites and stronger catalytic capacity can facilitate the solid–liquid–solid transformations of the S species. At the first stage of LiPS conversions in LSBs, S undergoes solid–liquid phase transformation to high-order LiPSs and then liquid–liquid conversion to low-order LiPSs. Rapid liquid–liquid phase conversions will reduce LiPS accumulation in the electrolyte, which is conductive to ion transport. To investigate the effect of interlayers on the conversions of LiPSs in a liquid–liquid phase, the CV curves of V2CTx/Ti3C2Tx-CP and Ti3C2Tx-CP symmetric cells were measured, as shown in Figure 2A. The current response of the CP symmetric cell without Li2S6 is almost in line. It can be seen from Figure 2A that the current response of V2CTx/Ti3C2Tx-CP symmetric cells with Li2S6 is the largest among the Ti3C2Tx-CP and CP cells, indicating that V2CTx/Ti3C2Tx composite materials accelerate the liquid–liquid conversion of LiPSs, make the liquid phase LiPS conversion become more thorough, and boost the LiPS redox kinetics. At the same time, the accumulation of LiPSs in the electrolyte will also be greatly reduced. This is due to the rapid conversion of liquid LiPSs. It is beneficial to suppress the shuttle effect. Figure 2B shows the EIS curves of symmetric cells. The smallest semicircle diameter of the V2CTx/Ti3C2Tx-CP symmetric cell implies the smallest charge transfer resistance (Rct), thus confirming the rapid electron and ion transportation at the interface between V2CTx/Ti3C2Tx composite materials and S species. Kinetics of Li2S precipitation in LSBs is another significant factor for high-performance LSBs. This is due to the fact that 75% of the capacity originates from the Li2S deposition during discharge. Therefore, Li2S nucleation tests were carried out. The constant-voltage discharge curves at 2.05 V are shown in Figures 2C,D. Obviously, the V2CTx/Ti3C2Tx-CP electrode demonstrates the earlier Li2S deposition compared with Ti3C2Tx-CP electrodes, indicating that the V2CTx/Ti3C2Tx-CP electrode has the greater catalytic ability, and accelerates the conversion of LiPSs to Li2S. The Li2S precipitation capacity on Ti3C2Tx-CP and V2CTx/Ti3C2Tx-CP electrodes were calculated by the integral of current vs. time, corresponding to 540 and 595 mAh·g−1, respectively. The precipitation capacity of the V2CTx/Ti3C2Tx-CP electrode becomes higher, implying that the faster LiPS conversion is achieved. The PP, Ti3C2Tx-PP, and V2CTx/Ti3C2Tx-PP cells were assembled to assess the effect of PP, Ti3C2Tx-PP, and V2CTx/Ti3C2Tx-PP on the electrochemical performance of LSBs. Figure 2E shows the CV profiles of PP, Ti3C2Tx-PP, and V2CTx/Ti3C2Tx-PP cells at a scan rate of 0.1 mV·s−1. Two reduction peaks for three cells can be seen and be attributed to the two reduction processes of S8 to LiPSs (Li2Sx, 4 ≤ x ≤ 8) and Li2S4 to Li2S2/Li2S, respectively. Two oxidation peaks correspond to the oxidation process of Li2S to LiPSs, which are then oxidized to S8. The CV curve of the V2CTx/Ti3C2Tx-PP cell shows the most intense peak, the lowest electrochemical polarization, and the highest current density, illustrating that the V2CTx/Ti3C2Tx composite interlayer makes LiPS redox reactions become the fastest among PP and Ti3C2Tx-PP cells. In addition, Figure 2F also reveals the excellent electrochemical kinetics of the V2CTx/Ti3C2Tx-PP cell from the EIS curve. The results show that the V2CTx/Ti3C2Tx-PP cell has the lowest Rct and excellent charge transfer ability at the electrolyte/electrode interface. This is due to its high ionic and electronic conductivity.
FIGURE 2. (A) CV curves of different symmetric cells under 50 mV·s−1 and (B) EIS spectra of V2CTx/Ti3C2Tx-CP, Ti3C2Tx-CP, and CP symmetric cells using Li2S6. Current vs. time curves for (C) Ti3C2Tx-CP and (D) V2CTx/Ti3C2Tx-CP electrodes. (E) CV curves of PP, Ti3C2Tx-PP, and V2CTx/Ti3C2Tx-PP cells at 0.1 mV·s−1. (F) EIS spectra of PP, Ti3C2Tx-PP, and V2CTx/Ti3C2Tx-PP cells.
The GCD curves of different cells were measured at 0.5 C, as shown in Figure 3A. The discharge capacities of V2CTx/Ti3C2Tx-PP, Ti3C2Tx-PP, V2CTx-PP, and PP cells are 1,090, 2, 977.3, 892.4, and 842.8 mAh·g−1, respectively. In contrast, the V2CTx/Ti3C2Tx-PP cell has the highest specific discharge capacity due to the existence of trace amounts of V2CTx. In addition, the polarization overpotential of the V2CTx/Ti3C2Tx-PP cell is only 211 mV, which is significantly smaller than that of other cells and consistent with the results of CV tests. Then, the cycling performance tests of the PP, Ti3C2Tx-PP, and V2CTx/Ti3C2Tx-PP cells were also performed, as shown in Figure 3B. The V2CTx/Ti3C2Tx-PP cell can still remain at a high discharge capacity of 775.2 mAh·g−1 and high capacity retention rate of 71% after 300 cycles. However, the capacity retention rate of Ti3C2Tx-PP and PP cells are only 69 and 52% after 300 cycles, respectively. Under the rate performance test shown in Figure 3C, the V2CTx/Ti3C2Tx cell shows excellent rate performance as the current density changes due to the good ionic and electronic conductivity of the V2CTx/Ti3C2Tx composite interlayer. In Figure 3C, the discharge capacity of the V2CTx/Ti3C2Tx-PP cell is 1,299.2, 1,185.9, 1,061.8, 998.3, and 935.3 mAh·g−1 at 0.1, 0.2, 0.5, 1, and 2 C, respectively. Subsequently, the discharge capacity can be restored to the high reversible capacity of 1,151.8 mAh·g−1 by reducing to 0.1 C. However, the discharge capacity of Ti3C2Tx-PP and PP cells is 753.9 and 469.2 mAh·g−1 at 2 C, respectively, indicating that the capacity decays faster with the increase in current density. Their reversible capacities correspond to 993 and 647.1 mAh·g−1 at 0.1 C, respectively. Figure 3D shows the GCD profiles of the V2CTx/Ti3C2Tx-PP cell. The polarization of the V2CTx/Ti3C2Tx-PP cell is only 123.4 mV at 0.1 C. The excellent rate performance and low polarization of the V2CTx/Ti3C2Tx-PP cell can be attributed to the important role of the mixed-dimensional V2CTx/Ti3C2Tx composite interlayer in electrical conductivity and chemical anchoring. The cycle stability at a high current density is an important factor to evaluate the performance of LSBs. Therefore, the long cycling stability of different batteries were tested at 1 C. In Figure 3E, the V2CTx/Ti3C2Tx-PP cell has an excellent long-term cycling stability with a high initial capacity of 969.9 mAh·g−1 and low capacity decay rate of 0.062% after 600 cycles. In contrast, the Ti3C2Tx-PP cell has a capacity decay rate of 0.074%. The PP cell is out of service after less than 400 cycles. The V2CTx/Ti3C2Tx cell demonstrates excellent electrochemical performance and cycle stability. It is also worth noting that the electrochemical performance of the mixed-dimensional V2CTx/Ti3C2Tx composite interlayer is highly competitive compared with that of the other materials reported (Table 1). In a word, the adsorbed LiPSs on the composite interlayer can quickly obtain electrons and ions at the adsorption sites to continue the redox reactions based on the high conductivity and abundant active sites of V2CTx/Ti3C2Tx. Meanwhile, the catalytic effect of V2CTx accelerates the LiPS redox kinetics.
FIGURE 3. (A) GCD curves of V2CTx/Ti3C2Tx-PP, Ti3C2Tx-PP, V2CTx-PP, and PP cells at 0.5 C. (B) Cycling performance of V2CTx/Ti3C2Tx-PP, Ti3C2Tx-PP, and PP cells at 0.5 C. (C) Rate performance of V2CTx/Ti3C2Tx-PP, Ti3C2Tx-PP, and PP cells. (D) GCD curves of the V2CTx/Ti3C2Tx-PP cell at current densities of 0.1, 0.2, 0.5, 1, and 2 C. (E) Cycling performance of V2CTx/Ti3C2Tx-PP, Ti3C2Tx-PP, and PP cells at 1 C.
TABLE 1. Comparison of the electrochemical performance between this work and other previously reported works.
In conclusion, we construct a mixed-dimensional V2CTx/Ti3C2Tx composite interlayer to suppress LiPS shuttling and accelerate LiPS redox kinetics. Profiting from the advantages of strong chemisorption of the composite interlayer to LiPSs and catalysis of V2CTx, the V2CTx/Ti3C2Tx cell achieves an excellent rate capacity of 935.3 mAh·g−1 at 2 C and low capacity rate decay of 0.062% after 600 cycles at 1 C. Meanwhile, designing a mixed-dimensional composite interlayer can provide a route to develop high-performance LSBs.
The original contributions presented in the study are included in the article/Supplementary Material. Further inquiries can be directed to the corresponding author/s.
WiZ, WcZ, and JY contributed to the material preparation and characterization. HL contributed to the TEM measurement. XZ and LW conceived the idea and designed the experiments.
This work was supported by the National Natural Science Foundation of China (52072099) and the Team Program of the Natural Science Foundation of Heilongjiang Province, China (No. TD 2021E005).
The authors declare that the research was conducted in the absence of any commercial or financial relationships that could be construed as a potential conflict of interest.
All claims expressed in this article are solely those of the authors and do not necessarily represent those of their affiliated organizations, or those of the publisher, the editors, and the reviewers. Any product that may be evaluated in this article, or claim that may be made by its manufacturer, is not guaranteed or endorsed by the publisher.
Cao, F. C., Zhang, Y., Wang, H. Q., Khan, K., Tareen, A. K., Qian, W. J., et al. (2022). Recent advances in oxidation stable chemistry of 2D MXenes. Adv. Mater. 34, 2107554. doi:10.1002/adma.202107554
Chen, L., Yang, W., Liu, J., and Zhou, Y. (2019). Decorating CoSe2 hollow nanospheres on reduced graphene oxide as advanced sulfur host material for performance enhanced lithium-sulfur batteries. Nano Res. 12, 2743–2748. doi:10.1007/s12274-019-2508-3
Chung, S. H., Chang, C. H., and Manthiram, A. (2018). Progress on the critical parameters for lithium-sulfur batteries to be practically viable. Adv. Funct. Mat. 28, 1801188. doi:10.1002/adfm.201801188
Gao, B., Li, Y. Y., Ma, C. Y., Shu, Y. Q., Wu, G., Chen, B. C., et al. (2022). Ta4C3 MXene as a saturable absorber for femtosecond mode-locked fiber lasers. J. Alloys Compd. 900, 163529. doi:10.1016/j.jallcom.2021.163529
Gao, L. F., Bao, W. L., Kuklin, A. V., Mei, S., Zhang, H., and Ågren, H. (2021). Hetero‐MXenes: Theory, synthesis, and emerging applications. Adv. Mat. 33, 2004129. doi:10.1002/adma.202004129
Gao, L. F., Chen, H. L., Kuklin, A. V., Wageh, S., Al-Ghamdi, A. A., Ågren, H., et al. (2022). Optical properties of few-layer Ti3CN MXene: From experimental observations to theoretical calculations. ACS Nano 16, 3059–3069. doi:10.1021/acsnano.1c10577
Gao, L. F., Ma, C. Y., Wei, S. R., Kuklin, A. V., Zhang, H., and Ågren, H. (2021). Applications of few-layer Nb2C MXene: Narrow-band photodetectors and femtosecond mode-locked fiber lasers. ACS Nano 15, 954–965. doi:10.1021/acsnano.0c07608
Gao, L. G., Li, C., Huang, W. C., Mei, S., Lin, H., Ou, Q., et al. (2020). MXene/polymer membranes: Synthesis, properties, and emerging applications. Chem. Mat. 32, 1703–1747. doi:10.1021/acs.chemmater.9b04408
Ghidiu, M., Lukatskaya, M. R., Zhao, M. Q., Gogotsi, Y., and Barsoum, M. W. (2014). Conductive two-dimensional titanium carbide 'clay' with high volumetric capacitance. Nature 516, 78–81. doi:10.1038/nature13970
Guo, P., Sun, K., Shang, X., Liu, D., Wang, Y., Liu, Q., et al. (2019). Nb 2 O5/RGO nanocomposite modified separators with robust polysulfide traps and catalytic centers for boosting performance of lithium-sulfur batteries. Small 15, 1902363. doi:10.1002/smll.201902363
Han, F. F., Jin, Q., Xiao, J. P., Wu, L. L., and Zhang, X. T. (2022). V2CTx catalyzes polysulfide conversion to enhance the redox kinetics of Li-S batteries. Dalton Trans. 51, 2560–2566. doi:10.1039/d1dt04158d
Hu, H. G., Shi, Z., Khan, K., Cao, R., Liang, W. Y., Tareen, A. K., et al. (2020). Recent advances in doping engineering of black phosphorus. J. Mat. Chem. A 8, 5421–5441. doi:10.1039/d0ta00416b
Huang, W. C., Zhu, J., Wang, M. K., Hu, L. P., Tang, Y. F., Shu, Y. Q., et al. (2020). Emerging mono‐elemental bismuth nanostructures: Controlled synthesis and their versatile applications. Adv. Funct. Mat. 31, 2007584. doi:10.1002/adfm.202007584
Li, L., Zhang, M. Y., Zhang, X. T., and Zhang, Z. G. (2017). New Ti3 C2 aerogel as promising negative electrode materials for asymmetric supercapacitors. J. Power Sources 364, 234–241. doi:10.1016/j.jpowsour.2017.08.029
Li, N., Chen, F., Chen, X. T., Chen, Z. X., Qi, Y., Li, X. D., et al. (2020). A bipolar modified separator using TiO2 nanosheets anchored on N-doped carbon scaffold for high-performance Li-S batteries. J. Mater. Sci. Technol. 55, 152–158. doi:10.1016/j.jmst.2019.09.012
Liang, X., Garsuch, A., and Nazar, L. F. (2015). Sulfur cathodes based on conductive MXene nanosheets for high-performance lithium-sulfur batteries. Angew. Chem. Int. Ed. 54, 3907–3911. doi:10.1002/anie.201410174
Ma, Q., Hu, M., Yuan, Y., Pan, Y., Chen, M., Zhang, Y., et al. (2020). Colloidal dispersion of Nb2O5/reduced graphene oxide nanocomposites as functional coating layer for polysulfide shuttle suppression and lithium anode protection of Li-S battery. J. Colloid Interface Sci. 566, 11–20. doi:10.1016/j.jcis.2020.01.066
Naguib, M., Kurtoglu, M., Presser, V., Lu, J., Niu, J., Heon, M., et al. (2011). Two-dimensional nanocrystals produced by exfoliation of Ti3AlC2. Adv. Mat. 23, 4248–4253. doi:10.1002/adma.201102306
Naguib, M., Unocic, R. R., Armstrong, B. L., and Nanda, J. (2015). Large-scale delamination of multi-layers transition metal carbides and carbonitrides "MXenes". Dalton Trans. 44, 9353–9358. doi:10.1039/c5dt01247c
Paolella, A., Laul, D., Timoshevskii, V., Zhu, W., Marras, S., Bertoni, G., et al. (2018). The role of metal disulfide interlayer in Li-S batteries. J. Phys. Chem. C 122, 1014–1023. doi:10.1021/acs.jpcc.7b08719
Qi, K. L., and Zhang, F. (2022). Rational surface engineering of Ti3C2Tx MXene for high-performance lithium-sulfur batteries. Mater. Lett. 318, 132134. doi:10.1016/j.matlet.2022.132134
Qiu, S. Y., Wang, C., Jiang, Z. X., Zhang, L. S., Gu, L. L., Wang, K. X., et al. (2020). Rational design of MXene@TiO2 nanoarray enabling dual lithium polysulfide chemisorption towards high-performance lithium-sulfur batteries. Nanoscale 12, 16678–16684. doi:10.1039/d0nr03528a
Song, Y., Cai, W., Kong, L., Cai, J., Zhang, Q., and Sun, J. (2019). Rationalizing electrocatalysis of Li-S chemistry by mediator design: Progress and prospects. Adv. Energy Mat. 10, 1901075. doi:10.1002/aenm.201901075
Song, Y. Z., Sun, Z. T., Fan, Z. D., Cai, W. L., Shao, Y. L., Sheng, G., et al. (2020). Rational design of porous nitrogen-doped Ti3C2 MXene as a multifunctional electrocatalyst for Li-S chemistry. Nano Energy 70, 104555. doi:10.1016/j.nanoen.2020.104555
Tian, Y., Li, G., Zhang, Y., Luo, D., Wang, X., Zhao, Y., et al. (2020). Low‐bandgap Se‐deficient antimony selenide as a multifunctional polysulfide barrier toward high‐performance lithium-sulfur batteries. Adv. Mat. 32, 1904876. doi:10.1002/adma.201904876
Wei, L., Li, W. L., Zhao, T., Zhang, N. X., Li, L., Wu, F., et al. (2020). Cobalt nanoparticles shielded in N-doped carbon nanotubes for high areal capacity Li-S batteries. Chem. Commun. 56, 3007–3010. doi:10.1039/c9cc08218b
Wu, M., He, Y., Wang, L. B., Xia, Q. X., and Zhou, A. G. (2020). Synthesis and electrochemical properties of V2C MXene by etching in opened/closed environments. J. Adv. Ceram. 9, 749–758. doi:10.1007/s40145-020-0411-8
Wu, X., Liu, N., Guan, B., Qiu, Y., Wang, M., Cheng, J., et al. (2019). Redox mediator: A new strategy in designing cathode for prompting redox process of Li-S batteries. Adv. Sci. 6, 1900958. doi:10.1002/advs.201900958
Xia, G., Zheng, Z. Q., Ye, J. J., Li, X. T., Biggs, M. J., and Hu, C. (2021). Carbon microspheres with embedded FeP nanoparticles as a cathode electrocatalyst in Li-S batteries. Chem. Eng. J. 406, 126823. doi:10.1016/j.cej.2020.126823
Yao, S., Cui, J., Huang, J. Q., Lu, Z., Deng, Y., Chong, W. G., et al. (2018). Novel 2D Sb2S3 nanosheet/CNT coupling layer for exceptional polysulfifide recycling performance. Adv. Energy Mat. 8, 1700279. doi:10.1002/aenm.201800710
Yao, Y., Wang, H., Yang, H., Zeng, S., Xu, R., Liu, F., et al. (2020). A dual‐functional conductive framework embedded with TiN‐VN heterostructures for highly efficient polysulfide and lithium regulation toward stable Li-S full batteries. Adv. Mat. 32, 1905658. doi:10.1002/adma.201905658
Ye, Z. Q., Jiang, Y., Li, L., Wu, F., and Chen, R. J. (2020). A high‐efficiency CoSe electrocatalyst with hierarchical porous polyhedron nanoarchitecture for accelerating polysulfides conversion in Li-S batteries. Adv. Mat. 32, 2002168. doi:10.1002/adma.202002168
Yu, B., Chen, D. J., Wang, Z. G., Qi, F., Zhang, X. J., Wang, X. Q., et al. (2020). Mo2C quantum dots@graphene functionalized separator toward high-current-density lithium metal anodes for ultrastable Li-S batteries. Chem. Eng. J. 399, 125837. doi:10.1016/j.cej.2020.125837
Zhang, B., Luo, C., Deng, Y. Q., Huang, Z. J., Zhou, G. M., Lv, W., et al. (2020). Optimized catalytic WS2 -WO3 heterostructure design for accelerated polysulfide conversion in lithium-sulfur batteries. Adv. Energy Mat. 10, 2000091. doi:10.1002/aenm.202000091
Zhang, W. Q., Jin, Q., Xiao, J. P., Yao, J., and Zhang, X. T. (2022). Rational design of adsorption-catalysis functional separator for highly efficient Li-S batteries. J. Alloys Compd. 900, 163414. doi:10.1016/j.jallcom.2021.163414
Keywords: polysulfides, sulfur redox kinetics, V2CTx/Ti3C2Tx, separator, shuttling, lithium–sulfur batteries
Citation: Zhang W, Zhang W, Yao J, Lu H, Zhang X and Wu L (2022) Mixed-dimensional V2CTx/Ti3C2Tx composite interlayer to boost electrochemical performance of Li-S batteries. Front. Chem. 10:1020538. doi: 10.3389/fchem.2022.1020538
Received: 16 August 2022; Accepted: 12 September 2022;
Published: 30 September 2022.
Edited by:
Weichun Huang, Nantong University, ChinaReviewed by:
Ye Zhang, University of South China, ChinaCopyright © 2022 Zhang, Zhang, Yao, Lu, Zhang and Wu. This is an open-access article distributed under the terms of the Creative Commons Attribution License (CC BY). The use, distribution or reproduction in other forums is permitted, provided the original author(s) and the copyright owner(s) are credited and that the original publication in this journal is cited, in accordance with accepted academic practice. No use, distribution or reproduction is permitted which does not comply with these terms.
*Correspondence: Xitian Zhang, eHR6aGFuZ3poYW5nQGhvdG1haWwuY29t; LiLi Wu, d2xsNzkwMTA3QGhvdG1haWwuY29t
†These authors have contributed equally to this work
Disclaimer: All claims expressed in this article are solely those of the authors and do not necessarily represent those of their affiliated organizations, or those of the publisher, the editors and the reviewers. Any product that may be evaluated in this article or claim that may be made by its manufacturer is not guaranteed or endorsed by the publisher.
Research integrity at Frontiers
Learn more about the work of our research integrity team to safeguard the quality of each article we publish.