- 1College of Resources and Environment, University of Chinese Academy of Sciences, Beijing, China
- 2Binzhou Institute of Technology, Weiqiao-UCAS Science and Technology Park, Binzhou, Shandong Province, China
- 3RCEES-IMCAS-UCAS Joint-Lab of Microbial Technology for Environmental Science, Beijing, China
Antibiotics widely exist in medical wastewater, which seriously endanger human health. With the spread of the COVID-19 and monkeypox around the world, a large number of antibiotics have been abused and discharged. How to realize the green and efficient treatment of medical wastewater has become a hot research topic. As a common electrochemical water treatment technology, electrochemical oxidation technology (EOT) could effectively achieve advanced treatment of medical wastewater. Since entering the 21st century, electrochemical oxidation water treatment technology has received more and more attention due to its green, efficient, and easy-to-operate advantages. In this study, the research progress of EOT for the treatment of medical wastewater was reviewed, including the exploration of reaction mechanism, the preparation of functional electrode materials, combining multiple technologies, and the design of high-efficiency reactors. The conclusion and outlook of EOT for medical wastewater treatment were proposed. It is expected that the review could provide prospects and guidance for EOT to treat medical wastewater.
Introduction
Antibiotics are one of the ubiquitous refractory organic pollutants (ROPs) in the water environment, and have attracted widespread attention due to their long-term residual, semi-volatile, and high toxicity (Scott et al., 2016). In particular, a large number of antibiotics exist in medical wastewater, and the concentration could reach the level of mg/L (Khan et al., 2020). The high toxicity and resistance to degradation of antibiotics could seriously endanger human health after entering the environment (Patangia et al., 2022). Trace amounts of antibiotics in the environment could increase the resistance of microorganisms, which leads to the development of drug-resistant superbugs (Sandegren, 2019). In addition, the number of papers related to the advanced treatment of medical wastewater continues to increase with the spread of the COVID-19 and monkeypox virus around the world (Figure 1). Therefore, it is urgent to find a green and efficient method to remove antibiotics in aqueous solution.
Currently, EOT is gradually becoming a favorable technology for degrading antibiotics from wastewater due to the mineralization of antibiotics into carbon dioxide and water (Lima et al., 2020). In particular, EOT has the advantages of green, high efficiency, easy operation, and mild reaction conditions (Xue et al., 2021). In addition, EOT could carry out biochemical and disinfection treatment of medical wastewater at the same time, which effectively cuts off the transmission path of pathogens (Lei et al., 2020). It has been widely concerned by researchers because of its unique application value. At present, the reaction rate of EOT was mainly improved through the development of functional electrode materials (Shestakova and Sillanpää, 2017). In addition, electrochemical oxidation water treatment technology could achieve deep purification of low-concentration medical wastewater by combining with photocatalytic technology and membrane treatment technology (Cho et al., 2019).
In this paper, the current research progress of EOT in medical wastewater treatment was reviewed. The advantages of EOT in water treatment are highlighted, and new research directions for EOT to treat medical wastewater were proposed, including functional electrode materials, multi-technology integration, and electrochemical reactors. Overall, this review provided new ideas for the development and engineering application of electrochemical oxidation water treatment technology.
Electrochemical indirect oxidation
Electrochemical indirect oxidation exhibited unique advantages compared with electrochemical direct oxidation. For example, the rate of migration of contaminants from the solution to the electrode surface would not be inhibited; the oxidation reaction on the anode surface would increase the electrochemical oxidation rate. In particular, a large amount of reactive intermediates (•OH, •O2−) was generated in the anode during the reaction process, and the medical wastewater was treated advanced through a single electron transfer process (Garcia-Segura et al., 2018).
Reactive intermediates
Hydroxyl radicals (•OH), active chlorine, chlorine-containing oxidants, etc. are the main reactive intermediates in the electrochemical indirect oxidation process. In particular, •OH exhibits stronger oxidative properties (E = 2.8V) compared to others (Miao et al., 2020). The generation of chemisorbed •OH was attributed to lattice oxygen in the anode material (Xu et al., 2022). However, physisorbed •OH was generated through the reaction between electrons and water molecules (or OH−) (Martínez-Huitle and Brillas, 2009). In addition, active chlorine species, including •Cl, Cl2, and HClO, were efficiently generated when electrochemical oxidation was used to treat chlorine-containing wastewater (Huang et al., 2020). Also, high health risk by-products chlorite and perchlorate were generated (Hao et al., 2022).
Anode material
The electrode material not only affected the oxygen evolution overpotential of the electrode, but also determined the amount and type of active intermediates generated. Chemisorbed •OH was generated on the surface of active electrodes (such as ruthenium, titanium) and interacted with the electrodes to generate superoxide. Moreover, the refractory organic pollutants were selectively oxidized into small molecular substances (Nakayama and Honda, 2021). However, physisorbed •OH was generated on the surface of inactive electrodes (such as Pb, Sn, Sb), which could mineralize organic matter into carbon dioxide and water (Mameda et al., 2020). At present, Dória et al. (2021) and Zhu et al. (2021) presented active electrodes such as Ti/RuO2−Sb2O4 and Ti/RuO2-ItO2 have been developed, indicating that the stability of active anodes could be effectively improved by loading metal oxides. The stability and activity of inactive anode materials were enhanced by means of doping or intercalation (Rai and Sinha, 2022). The oxidation rate of chlorinated hydrocarbons was increased by 60% when using the inactive anode compared to the active anode, which might be attributed to the free radicals generated by the inactive anode (Zhang et al., 2016). Interestingly, the removal efficiency of organics by the active electrode was significantly higher than that of the inactive electrode in the treatment of chlorinated wastewater. This might be attributed to the higher instantaneous current efficiency of metal active electrodes compared with graphite electrodes and diamond electrodes (Kraft et al., 2002). As reported, the generation efficiency of active chlorine of Ru, Ir, and Pt electrodes is significantly higher than that of inactive electrodes (such as PbO2 and SnO2) (Sirés et al., 2014). Zinc oxide-coated electrodes could effectively degrade perfluorinated compounds in wastewater, removing 66% of pollutants within 40 min (Zhang et al., 2016). Therefore, active electrodes were preferentially used in the treatment of chlorine-containing wastewater.
Mediated electrochemical oxidation
Mediated electrochemical oxidation degrades organic pollutants by reversible redox couples. The redox substances could not only be recycled, but also could avoid the surface of the anode material from being polluted by organic substances (Figure 2A). Ag2+ is a strong oxidant and could react with halide ions to form precipitates during water treatment, thereby reducing the rate of electrochemical oxidation (Kakiuchi and Samec, 2020). As reported, Co3+ as an oxidant could not only improve the kinetics of the oxidation reaction, but also effectively avoid the formation of precipitates (Sequeira et al., 2006). Ce4+ acted as a medium to participate in the electrochemical oxidation process, and the solution pH, electrolyte, and current density affected the oxidation rate of cerium and then changed the degradation rate of phenolic pollutants (Matheswaran et al., 2008). In particular, Ce4+ exhibited excellent stability during the reaction. Moreover, the reaction temperature, reaction time, and reactor flow rate were all factors that affected the rate of the mediated electrochemical oxidation reaction (Balaji et al., 2008). Overall, the mediated oxidation process could be enhanced by adding redox substances, thereby improving the removal efficiency of pollutants in the treatment of actual wastewater.
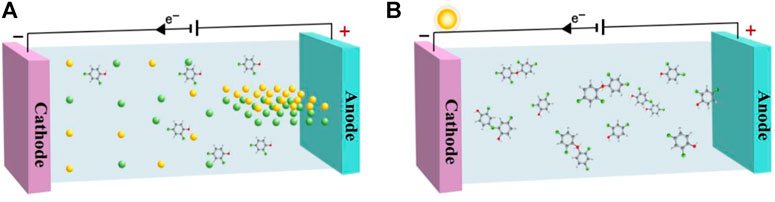
FIGURE 2. (A) Mediated electrochemical oxidation system, (B) Photo/electrochemical oxidation system.
Photo/electrochemical oxidation
Photo/electrochemical oxidation is a photoresponse that generates holes and electrons under illumination conditions, which are enhanced by the action of an electric field (Figure 2B). TiO2 is currently a commonly used photocatalyst, and electron-holes are generated by electron transitions from the valence band (VB) to the conduction band (CB) under UV (<380 nm) light irradiation (Liu et al., 2019a). Reactive oxygen species (ROS) could be efficiently generated by single-electron transfer of photogenerated electrons (holes), thereby decomposing organic pollutants (Tao et al., 2020). In addition, the applied electric field could effectively inhibit the recombination of electron-holes and accelerate the generation of ROS. The photo-assisted electrochemical system was constructed using TiO2 as the electrode material, which improved the degradation efficiency of bisphenol A (Liu et al., 2019b). The TiO2/Ti electrode exhibited good stability under low current density conditions and was suitable for application in a three-electrode system. It has been reported that the TiO2/Ti electrode could effectively degrade pollutants under UV irradiation, which was attributed to the generation of ROS (Carneiro et al., 2004). Due to the stability at high current density, Ru0.3Ti0.7O2/Ti electrodes were widely used in two-electrode systems. The degradation efficiency of total organic carbon (TOC) increased by 50% in the Ru0.3Ti0.7O2/Ti/UV system, which might be due to the photoelectric synergistic generation of ·OH (Hussain et al., 2017). In particular, BiOCl/NaNbO3 nanomaterials exhibited high current density and electron-hole separation efficiency due to their special bandgap position and layered structure (Li et al., 2022). Overall, the photo/electrochemical oxidation synergistic system could effectively improve the degradation efficiency of wastewater.
Electrochemical reactor
The electron transfer rate and the degradation efficiency of organic pollutants during the reaction are not only related to the electrode material, but also to the configuration of the reactor. At present, the commonly used electrochemical reactors are submerged and penetrating. Due to the free diffusion method, the submerged electrochemical reactor had the disadvantages of low current efficiency and low mass transfer rate, and could not treat low-concentration wastewater (Figure 3A). In particular, penetrating electrochemical reactors exhibited numerous advantages due to their unique sandwich structure, including small plate spacing, high electron transfer rate, and low energy consumption (Figure 3B) (Wangwang et al., 2019). In comparison to the submerged electrochemical reactor, Santos et al. (2014) showed the degradation efficiency of pollutants increased by 200% using the penetrating electrochemical reactor, which was attributed to its sandwich reactor structure. Using carbon-based catalysts as electrocatalytic anodes, the degradation efficiency of pollutants was increased by 600% in the penetrating electrochemical reactor, which was due to the efficient mass transfer efficiency (Han et al., 2012). A novel type of flow reactor was invented, and the degradation efficiency of synergistic electrochemical oxidation of levofloxacin increased by 95% (Pieczyńska et al., 2019). A tubular electrode reactor was designed to enhance electrochemical wastewater treatment, which was attributed to the titanium oxide (M-TiSO) anode and superior reactor configuration (Liang et al., 2021). The construction of an electric Fenton/double anode reactor system significantly improved the degradation efficiency of bisphenol A, which was attributed to the slow release of Fe2+ (Zhao et al., 2022). Also, this indicated a good synergistic effect between the electric Fenton and the double anode reactor. A penetrating electrochemical reactor was constructed using graphene as the electrode material to treat chlorine-containing wastewater and exhibited good removal efficiency, which was attributed to the HClO generated during the reaction (Liu et al., 2014). Moreover, the rate of electrochemical oxidation reaction was affected by the mode of power supply. Compared with DC power supply, pulse power supply not only improved the utilization rate of electric energy, but also effectively reduced the deposition of organic matter on the anode surface. As reported, the degradation efficiency was increased by 80% using the pulse power supply method, and the effects of the initial pollutant concentration and current density were investigated (Jiang et al., 2021). Overall, the penetrating electrochemical reactor in the pulse power supply mode and the electric fenton could effectively improve the treatment efficiency of wastewater.
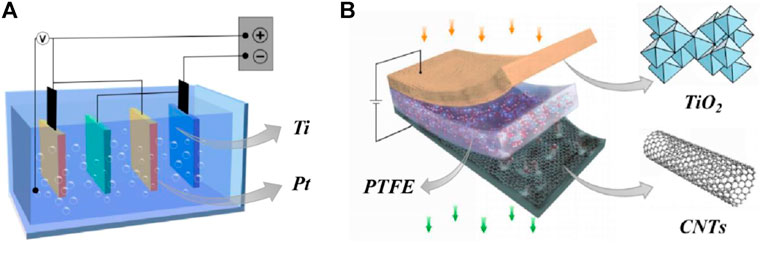
FIGURE 3. (A) Submerged electrochemical reactor system, (B)Penetrating electrochemical reactor system.
Conclusion and outlook
With the development of material technology and electrochemical technology, the research work on electrochemical oxidation water treatment technology showed a continuous and in-depth trend. Novel electrode materials and electrochemical reactors were constantly being developed, forming a green, efficient, and energy-saving electrochemical oxidation water treatment technology. In addition, the research on the joint application of various technologies had made great progress. A large number of articles discussed the application of EOT in water treatment, but most of them remained in the laboratory stage. Various problems still exist in the application process and need to be solved urgently. The main research directions in the future were proposed.
1) Preparation of high-performance electrode materials. High-performance electrode materials could effectively improve the rate of interfacial electron transfer and mass transfer, which was the key to electrochemical water oxidation treatment technology. Furthermore, the economics of electrode materials should be considered during practical engineering applications.
2) Optimization of electrochemical reactors. Excellent electrochemical reactors could effectively improve the efficiency of water treatment and the utilization of electricity by interface regulation. Also, the design of the electrochemical reactor should consider the ease of operation in practical applications.
3) Combined application of multiple technologies. By the combination of electric Fenton, light, media and EOT, the synergy between technologies could be exerted to improve the efficiency of water treatment. Moreover, potential environmental risks should be considered in the combined application of multiple technologies.
Data availability statement
The original contributions presented in the study are included in the article/Supplementary Material, further inquiries can be directed to the corresponding author.
Author contributions
CZ: conceptualization, methodology, writing—original draft, writing—review and editing. ZY: supervision, funding acquisition, writing—original draft. CZ and XW discussed the results and revised the manuscript.
Funding
This work was funded by Binzhou Institute of Technology (GYY-DTFZ-2022-003), and the Fundamental Research Funds for the Central Universities.
Conflict of interest
The authors declare that the research was conducted in the absence of any commercial or financial relationships that could be construed as a potential conflict of interest.
Publisher’s note
All claims expressed in this article are solely those of the authors and do not necessarily represent those of their affiliated organizations, or those of the publisher, the editors and the reviewers. Any product that may be evaluated in this article, or claim that may be made by its manufacturer, is not guaranteed or endorsed by the publisher.
References
Balaji, S., Chung, S. J., Matheswaran, M., Vasilivich, K. V., and Moon, I. S. (2008). Destruction of organic pollutants by cerium(IV) meo process: A study on the influence of process conditions for edta mineralization. J. Hazard. Mat. 150 (3), 596–603. doi:10.1016/j.jhazmat.2007.05.009
Carneiro, P. A., Osugi, M. E., Sene, J. J., Anderson, M. A., and Zanoni, M. (2004). Evaluation of color removal and degradation of a reactive textile azo dye on nanoporous TiO2 thin-film electrodes. Electrochimica Acta 49 (22-23), 3807–3820. doi:10.1016/j.electacta.2003.12.057
Cho, K., Lee, S., Kim, H., Kim, H.-E., Son, A., Kim, E.-j., et al. (2019). Effects of reactive oxidants generation and capacitance on photoelectrochemical water disinfection with self-doped titanium dioxide nanotube arrays. Appl. Catal. B Environ. 257, 117910. doi:10.1016/j.apcatb.2019.117910
Dória, A. R., Santos, G. O. S., Pelegrinelli, M. M. S., Silva, D. C., de Matos, D. B., Cavalcanti, E. B., et al. (2021). Improved 4-nitrophenol removal at Ti/RuO2–Sb2O4–TiO2 laser-made anodes. Environ. Sci. Pollut. Res. 28 (19), 23634–23646. doi:10.1007/s11356-020-10451-6
Garcia-Segura, S., Ocon, J. D., and Chong, M. N. (2018). Electrochemical oxidation remediation of real wastewater effluents—a review. Process Saf. Environ. Prot. 113, 48–67. doi:10.1016/j.psep.2017.09.014
Han, L., Chad, D., and Veciti, N. (2012). Reactive transport mechanism for organic oxidation during electrochemical filtration: Mass-transfer, physical adsorption, and electron-transfer. J. Phys. Chem. C 116 (1), 374–383. doi:10.1021/jp209390b
Hao, Y., Ma, H., Proietto, F., Galia, A., and Scialdone, O. (2022). Electrochemical treatment of wastewater contaminated by organics and containing chlorides: Effect of operative parameters on the abatement of organics and the generation of chlorinated by-products. Electrochimica Acta 402, 139480. doi:10.1016/j.electacta.2021.139480
Huang, X., Zhang, Y., Bai, J., Li, J., Li, L., Zhou, T., et al. (2020). Efficient degradation of N-containing organic wastewater via chlorine oxide radical generated by a photoelectrochemical system. Chem. Eng. J. 392, 123695. doi:10.1016/j.cej.2019.123695
Hussain, S., Steter, J. R., Gul, S., and Motheo, A. J. (2017). Photo-assisted electrochemical degradation of sulfamethoxazole using a Ti/Ru0.3Ti0.7O2 anode: Mechanistic and kinetic features of the process. J. Environ. Manage. 201, 153–162. doi:10.1016/j.jenvman.2017.06.043
Jiang, Z., Cheng, Z., Yan, C., Zhang, X., Tian, Y., Zhang, X., et al. (2021). Simultaneous removal of nitrogen and refractory organics from a biologically treated leachate by pulse electrochemical oxidation in a multi-channel flow reactor. ACS Omega 6 (39), 25539–25550. doi:10.1021/acsomega.1c03567
Kakiuchi, T., and Samec, Z. (2020). An electrochemical viewpoint on the solubility of silver halides in water. J. Solid State Electrochem. 24 (11), 3185–3189. doi:10.1007/s10008-020-04792-y
Khan, N. A., Ahmed, S., Farooqi, I. H., Ali, I., Vambol, V., Changani, F., et al. (2020). Occurrence, sources and conventional treatment techniques for various antibiotics present in hospital wastewaters: A critical review. TrAC Trends Anal. Chem. 129, 115921. doi:10.1016/j.trac.2020.115921
Kraft, A., Blaschke, M., and Kreysig, D. (2002). Electrochemical water disinfection Part III: Hypochlorite production from potable water with ultrasound assisted cathode cleaning. J. Appl. Electrochem. 32 (6), 597–601. doi:10.1023/a:1020199313115
Lei, Q., Zheng, J., Ma, J., Wang, X., Wu, Z., and Wang, Z. (2020). Simultaneous solid-liquid separation and wastewater disinfection using an electrochemical dynamic membrane filtration system. Environ. Res. 180, 108861. doi:10.1016/j.envres.2019.108861
Li, L., Cao, W., Yao, J., Liu, W., Li, F., and Wang, C. (2022). Synergistic piezo-photocatalysis of BiOCl/NaNbO3 heterojunction piezoelectric composite for high-efficient organic pollutant degradation. Nanomaterials 12 (3), 353. doi:10.3390/nano12030353
Liang, J., You, S., Yuan, Y., and Yuan, Y. (2021). A tubular electrode assembly reactor for enhanced electrochemical wastewater treatment with a Magnéli-phase titanium suboxide (M-TiSO) anode and in situ utilization. RSC Adv. 11 (40), 24976–24984. doi:10.1039/d1ra02236a
Lima, V. B., Goulart, L. A., Rocha, R. S., Steter, J. R., and Lanza, M. R. V. (2020). Degradation of antibiotic ciprofloxacin by different AOP systems using electrochemically generated hydrogen peroxide. Chemosphere 247, 125807. doi:10.1016/j.chemosphere.2019.125807
Liu, B., Yang, J., Wang, J., Zhao, X., and Nakata, K. (2019a). High sub-band gap response of TiO2 nanorod arrays for visible photoelectrochemical water oxidation. Appl. Surf. Sci. 465, 192–200. doi:10.1016/j.apsusc.2018.09.098
Liu, C., Zhang, A.-Y., Si, Y., Pei, D.-N., and Yu, H.-Q. (2019b). Photochemical protection of reactive sites on defective TiO2–x surface for electrochemical water treatment. Environ. Sci. Technol. 53 (13), 7641–7652. doi:10.1021/acs.est.9b01307
Liu, Y., Dustin Lee, J. H., Xia, Q., Ma, Y., Yu, Y., Lanry Yung, L. Y., et al. (2014). A graphene-based electrochemical filter for water purification. J. Mat. Chem. A 2 (39), 16554–16562. doi:10.1039/c4ta04006f
Mameda, N., Park, H., and Choo, K.-H. (2020). Hybrid electrochemical microfiltration treatment of reverse osmosis concentrate: A mechanistic study on the effects of electrode materials. Desalination 493, 114617. doi:10.1016/j.desal.2020.114617
Martínez-Huitle, C., and Brillas, E. (2009). Decontamination of wastewaters containing synthetic organic dyes by electrochemical methods: A general review. Appl. Catal. B Environ. 87 (3-4), 105–145. doi:10.1016/j.apcatb.2008.09.017
Matheswaran, M., Balaji, S., Sang, J. C., and Moon, I. S. (2008). Mediated electrochemical oxidation of phenol in continuous feeding mode using Ag (II) and Ce (IV) mediator ions in nitric acid: A comparative study. Chem. Eng. J. 144 (1), 28–34. doi:10.1016/j.cej.2008.01.005
Miao, F., Gao, M., Yu, X., Xiao, P., Wang, M., Wang, Y., et al. (2020). TiO2 electrocatalysis via three-electron oxygen reduction for highly efficient generation of hydroxyl radicals. Electrochem. Commun. 113, 106687. doi:10.1016/j.elecom.2020.106687
Nakayama, T., and Honda, R. (2021). Electrochemical and mechanistic study of oxidative degradation of favipiravir by electrogenerated superoxide through proton-coupled electron transfer. ACS Omega 6 (33), 21730–21740. doi:10.1021/acsomega.1c03230
Patangia, D. V., Anthony Ryan, C., Dempsey, E., Paul Ross, R., and Stanton, C. (2022). Impact of antibiotics on the human microbiome and consequences for host health. MicrobiologyOpen 11 (1), e1260. doi:10.1002/mbo3.1260
Pieczyńska, A., Ossowski, T., Bogdanowicz, R., and Siedlecka, E. (2019). Electrochemical degradation of textile dyes in a flow reactor: Effect of operating conditions and dyes chemical structure. Int. J. Environ. Sci. Technol. (Tehran). 16 (2), 929–942. doi:10.1007/s13762-018-1704-0
Rai, D., and Sinha, S. (2022). Research trends in the development of anodes for electrochemical oxidation of wastewater. Rev. Chem. Eng. 0, 67. doi:10.1515/revce-2021-0067
Sandegren, L. (2019). Low sub-minimal inhibitory concentrations of antibiotics generate new types of resistance. Sustain. Chem. Pharm. 11, 46–48. doi:10.1016/j.scp.2018.12.006
Santos, E. D., Sena, S., Silva, D., Ferro, S., Battisti, A. D., and Martínez-Huitle, C. (2014). Scale-up of electrochemical oxidation system for treatment of produced water generated by Brazilian petrochemical industry. Environ. Sci. Pollut. Res. 21 (14), 8466–8475. doi:10.1007/s11356-014-2779-x
Scott, G. I., Porter, D. E., Norman, R. S., Scott, C. H., Uyaguari-Diaz, M. I., Maruya, K. A., et al. (2016). Antibiotics as CECs: An overview of the hazards posed by antibiotics and antibiotic resistance. Front. Mar. Sci. 3, 24. doi:10.3389/fmars.2016.00024
Sequeira, C., Santos, D., and Brito, P. (2006). Mediated and non-mediated electrochemical oxidation of isopropanol. Appl. Surf. Sci. 252 (17), 6093–6096. doi:10.1016/j.apsusc.2005.11.028
Shestakova, M., and Sillanpää, M. (2017). Electrode materials used for electrochemical oxidation of organic compounds in wastewater. Rev. Environ. Sci. Biotechnol. 16 (2), 223–238. doi:10.1007/s11157-017-9426-1
Sirés, I., Brillas, E., Oturan, M. A., Rodrigo, M. A., and Panizza, M. (2014). Electrochemical advanced oxidation processes: Today and tomorrow. A review. Environmental Science&Pollution Research 21 (14), 8336–8367. doi:10.1007/s11356-014-2783-1
Tao, C., Jia, Q., Han, B., and Ma, Z. (2020). Tunable selectivity of radical generation over TiO2 for photocatalysis. Chem. Eng. Sci. 214, 115438. doi:10.1016/j.ces.2019.115438
Wangwang, He, Tang, Jie, Liang, D., Jilai, G., Lin, , Liu, Z., et al. (2019). Various cell architectures of capacitive deionization: Recent advances and future trends. Water Res. 150, 225–251. doi:10.1016/j.watres.2018.11.064
Xu, J., Liu, Y., Li, D., Li, L., Zhang, Y., Chen, S., et al. (2022). Insights into the electrooxidation of florfenicol by a highly active La-doped Ti4O7 anode. Sep. Purif. Technol. 291, 120904. doi:10.1016/j.seppur.2022.120904
Xue, Y., Hu, X., Sun, Q., Wang, H.-y., Wang, H.-l., and Hou, X.-m. (2021). Review of electrochemical degradation of phenolic compounds. Int. J. Min. Metall. Mat. 28 (9), 1413–1428. doi:10.1007/s12613-020-2241-7
Zhang, C., Tang, J., Peng, C., and Jin, M. (2016). Degradation of perfluorinated compounds in wastewater treatment plant effluents by electrochemical oxidation with Nano-ZnO coated electrodes. J. Mol. Liq. 221, 1145–1150. doi:10.1016/j.molliq.2016.06.093
Zhao, M., Ma, X., Li, R., Mei, J., Rao, T., Ren, G., et al. (2022). In-situ slow production of Fe2+ to motivate electro-Fenton oxidation of bisphenol A in a flow through dual-anode reactor using current distribution strategy: Advantages, CFD and toxicity assessment. Electrochimica Acta 411, 140059. doi:10.1016/j.electacta.2022.140059
Keywords: electrochemical oxidation, electrode materials, electrochemical reactor, medical wastewater, advanced treatment
Citation: Zhang C, Yu Z and Wang X (2022) A review of electrochemical oxidation technology for advanced treatment of medical wastewater. Front. Chem. 10:1002038. doi: 10.3389/fchem.2022.1002038
Received: 24 July 2022; Accepted: 23 August 2022;
Published: 15 September 2022.
Edited by:
Dan Shao, Shaanxi University of Science and Technology, ChinaReviewed by:
Zuzanna Bielan, Institute of Fluid Flow Machinery (PAN), PolandCopyright © 2022 Zhang, Yu and Wang. This is an open-access article distributed under the terms of the Creative Commons Attribution License (CC BY). The use, distribution or reproduction in other forums is permitted, provided the original author(s) and the copyright owner(s) are credited and that the original publication in this journal is cited, in accordance with accepted academic practice. No use, distribution or reproduction is permitted which does not comply with these terms.
*Correspondence: Zhisheng Yu, eXV6c0B1Y2FzLmFjLmNu