- School of Materials Science and Engineering, Anhui University of Technology, Maanshan, China
Two-dimensional (2D) carbon materials are considered as efficient catalysts for improving hydrogen storage in MgH2, but their catalytic mechanisms of different materials remain unclear. Herein we compare the hydrogen storage properties of MgH2 with doping different 2D carbon materials for revealing their catalytic effecting mechanisms. It can be seen that the effect of 2D metal carbides including Nb2C and Ti2C are superior to 2D graphene for improving hydrogen storage properties of MgH2, where the Ti2C exhibits the best catalytic effect with a remarkable decrease of activation energy (Ea) from ∼124 kJ/mol for doping graphene to ∼86 kJ/mol. This is related to the changes of individual metal and graphite chemical valence states of catalysts. The high catalytic activity of the hydrogen storage reaction originates from its unique layered structure and in situ formation of MHX, i.e., the tiny metal crystals can serve as a channel to facilitate hydrogen transport in MgH2 matrix. Moreover, the Ti catalytic effect is better than Nb, which originates from the surface of the multivalent Ti atoms is an intermediate of the electron moving between H− and Mg2+, thus leading to the Ti2C catalyzed MgH2 with superior hydrogen kinetic and cyclic performance.
Introduction
Magnesium ranks sixth in the Earth’s crust, is widely distributed in nature, is inexpensive, and its 7.6 wt% hydrogen storage capacity and good reversibility make magnesium a hot topic for hydrogen storage materials (Nabgan et al., 2017; Shao et al., 2018). Meanwhile, the position of magnesium in the periodic table is the second main family in the third cycle, belonging to lively metal. Thus, magnesium oxide films will form on the surface, which is not conducive to the hydrogenation reaction. Besides, the hydrogenation enthalpy of MgH2 was 76.0 kJ/mol. It is much higher than that of most metal hydrides (generally 20–40 kJ/mol), resulting in poor thermodynamic stability, which is due to the strong ionic bond between hydrogen and magnesium (Ding et al., 2017; Si et al., 2021); finally, MgH2 starts to absorb hydrogen at about 285°C and hydrogenates at 385°C, because the molecular hydrogen needs to overcome 432 kJ/mol energy barrier to carry out the hydrogenation reaction with magnesium (Zhang et al., 2011). The high temperature of hydrogen absorption and desorption and the slow rate of hydrogen absorption and release lead to its poor kinetic performance (Chen and Zhu, 2008; Jain et al., 2010; Ma et al., 2017). Although MgH2 has certain advantages in hydrogen storage materials, it still needs to be further improved in its hydrogen storage performance to achieve practical application.
Lowering the operating temperature and increasing the rate of dehydrogenation of MgH2 are key challenges for its application, which can be overcome by catalytic, alloying, nano-grading and nano-constraining methods (Xia et al., 2015; Yu et al., 2017; Zhu et al., 2020a). Adjusting the binding energy of the Mg-H bonds by the alloy element (Edalati et al., 2018) or locally by additives is an effective way to alter the MgH2 thermodynamics. Catalyst doping (Wang et al., 2018) has proven to be the most feasible and efficient method to accelerate MgH2 dehydrogenation dynamics. However, nanoscale Mg-based hydrogen storage materials will agglomerate after repeated hydrogen absorption and discharge, leading to the degradation of hydrogen storage performance, and similarly repeated Mg-based alloy-based hydrogen storage material powders will lead to the degradation of hydrogen storage performance. It was shown that the hydrogen adsorption performance of mixtures of MgH2 and carbon materials including graphite, activated carbon, multi-walled carbon nanotubes (MWCNTs), carbon nanofibers (CNF) and activated carbon fibers were studied by M. A. et al. (Lillo-Ródenas et al., 2008). By comparison, the introduction of graphite can effectively reduce the decomposition temperature of MgH2. This is due to the superior physicochemical properties and high specific surface area of graphene, and most importantly, graphene has a two-dimensional monolayer crystal structure, which increases the interfacial area and thus provides more active catalytic sites (Novoselov et al., 2004).
Moreover, transition metal (TM) [Ni (Zou et al., 2012), Ti (Ren et al., 2014; Lotoskyy et al., 2018), Nb (Liu et al., 2015), Fe (Chen et al., 2016), Co (Lu et al., 2017; Khan et al., 2019)], transition metal oxides [TiO2 (Zhang et al., 2019; Ma et al., 2020), Nb2O5 (Hanada et al., 2006)] and transition metal-based alloys [TiCu (Zhou et al., 2019), FeNb (Santos et al., 2014)] are the most feasible and reliable alloys for the catalysts of magnesium-based hydrogen storage materials. Meanwhile, two-dimensional carbon material catalysts are also widely used in MgH2 systems such as Ti3C2/TiO2(A)-C (Gao et al., 2020), Ni@FL-Ti3C2 (Zhu et al., 2020b; Gao et al., 2020; ), Ni@C-Mxene (Huang et al., 2021), etc. Large surface area or nanobunching can explain the excellent catalytic efficiency of catalysts with unique structural features. Ma et al. (2013) reported the distribution of small metal nanocrystals in the composites as a way to facilitate hydrogen transport and improve dehydrogenation performance. A contradictive mechanism was later proposed by Li et al. (2006) in density flooding theory calculations, and their analysis showed that Ti2C is only a catalyst rather than a reactant, where the polyvalent Ti atoms on the surface act as an intermediate for electron transport between H− and Mg2+, making dehydrogenation easier. And the good hydrogen adsorption capacity and thermal conductivity of Ti2C 2D materials also contribute to the improvement of the dehydrogenation thermodynamics of MgH2. These contradictive mechanisms enable us to further clarify the intrinsic catalytic mechanism by comparison of different 2D materials including graphene, Nb2C, and Ti2C.
In this paper, the distinct hydrogen storage properties of Mg-based materials are examined by adding different 2D carbides catalysts. As compared to single graphene catalysts, the synergy of introduced transition metal TM (Nb, Ti) and graphite was systematically studied to explore the effect of each catalyst on the hydrogen storage performance of MgH2, and the catalytic effect of two composites including MgH2@Nb2C and MgH2@Ti2C was carefully compared with MgH2@Graphene, thus revealing the difference in the effect of 2D carbides on the hydrogen storage of MgH2.
Experimental section
Preparation of MgH2 2D carbon composites
The starting chemicals of magnesium hydride (MgH2, purity 95%, powder) and two-dimensional graphene (Graphene, purity 99%, powder) from Alfa Aesar and Nb2C (purity 99%, powder) and Ti2C (purity 98%, powder) from Xianfeng Nanomaterials Technology Co, China. First, a total of 500 mg of MgH2 powder and 2D graphene powder with a mass ratio of 19:1 were weighed and placed in a ball mill jar with an argon atmosphere, a ball-to-material ratio of 40:1, a rotation speed of 400 r/min, and ball milled on a ball mill model XQM-2A for 10 h. The treatment of MgH2 as a comparison sample and the process of preparing MgH2@Graphene was kept consistent. Then, the preparation process of MgH2@Nb2C and MgH2@Ti2C was kept the same as that of preparing MgH2@Graphene.
Characterization and hydrogen sorption measurements
To understand the composition and structure of the samples, the properties of the samples such as cell parameters, crystallographic surface indices, dot matrix parameters and atomic occupancies were further analyzed using software such as Jade 6 and Powder X, respectively. The XRD instrument used to test the samples was a D2 PHASER XE-T Edition with an energy resolution of up to 380 eV, and a Cu target was selected as the ray tube. The hydrogen desorption capacity, temperature and reaction rate of the hydrogen storage material were measured directly using DSC. For the experiments, a 20 mg sample is placed in a quartz vessel and then placed in a reaction vessel at a temperature range from room temperature to 400°C, with the heating rate set by the properties of the sample. During the test, the mechanical automatic recording of the corresponding mass and temperature at each temperature can further calculate the amount of hydrogen release, heat absorption peak and heat release peak. To further observe the tissue morphology of the composites, a scanning electron microscope (SEM) of NONA NanoSEM 430 from DYC was used to observe the composites and combined with Mapping to analyze and determine the elemental composition and elemental distribution. Before sample testing, the samples were treated with conductive adhesive and observed using backscattered electron imaging mode. Talos F 200 X S/TEM type equipment was used to further visualize the tissue morphology of the samples through microscopic (TEM) and high resolution (STEM) images, and then combined with Digital Micrograph software to perform IFFT (Fast Fourier Transform) and FFT (Fast Fourier Transform) on the transmission electron diffraction images of the samples to observe the lattice images, atomic arrangement and crystallographic indices. To prepare the samples, they were placed in tetrahydrofuran (THF), followed by ultrasonic shaking, well dispersed and coated onto porous carbon films, and finally dried. All preparations are done in a glove box containing argon gas (>99.99% purity). The samples required for the experiment are loaded into the reaction vessel and then taken out of the glove box and connected to the P-C-T experimental setup to enter the testing phase. In the constant temperature hydrogen absorption and discharge test phase, the amount of hydrogen released can be found according to the pressure change and the curve of hydrogen pressure change with time can be made.
Results and discussion
Structural and morphological features
Figure 1A shows the XRD pattern of the ball-milled product of MgH2@Graphene composite, from which the diffraction peaks of MgH2 and Graphene can be seen, indicating that the graphene is loaded with MgH2. To further prove that the ball-milled product contains Graphene, the Raman test was performed on it, and the Raman pattern is shown in Figure 1B, with wave numbers 1,380 and 1,570 cm−1 show distinct peaks, which represent the D-band and G-band of graphene, respectively; the D-band corresponds to disordered carbon or defective graphite structures in the sample, and the G-band is characteristic of graphite layers (Grochala and Edwards, 2004), confirming the presence of graphene. Figure 1C shows the XRD image of the ball-milled product of the MgH2@Nb2C composite, from which only MgH2 is present in the ball-milled product, probably because the carbon and niobium nanosized particles are too fine to be detected, to further prove the presence of carbon and niobium in the ball-milled product, Raman tests were performed on it. The Raman spectrum is shown in Figure 1D, which shows distinct peaks at wave numbers 1,374 and 1,568 cm−1, which represent the D-band and G-band of graphene, respectively, confirming the carbon as graphene.
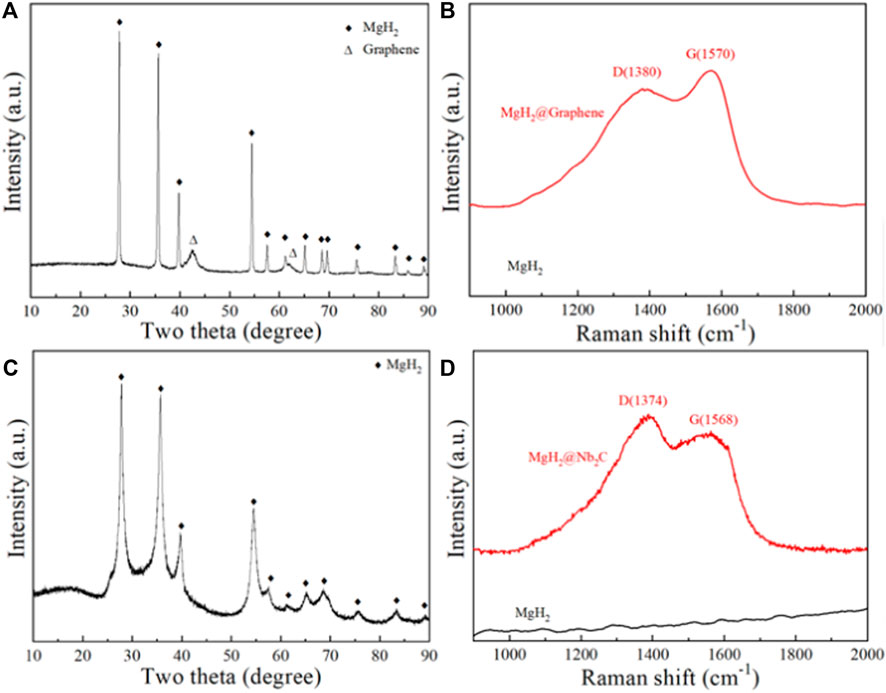
FIGURE 1. MgH2@Graphene composites for (A) XRD pattern and (B) Raman pattern, MgH2@Nb2C composites for (C) XRD pattern and (D) Raman pattern.
To further study the microscopic morphology of the MgH2@Graphene and MgH2@Nb2C composites, scanning electron microscopy analysis was performed. The scanning electron microscopy analysis of MgH2@Graphene composites is shown in Figure 2. It is obvious that the MgH2@Graphene particles are smaller than those of pure MgH2, and it can also be found that the MgH2@Graphene particles are more uniformly distributed in size and do not appear to be agglomerated; the corresponding elemental distribution diagram observes that the carbon is more like a mesh structure, making the MgH2 particles uniformly loaded on the graphite layer. The finer MgH2@Graphene particles are attributed to the reaction process between MgH2 and two-dimensional graphene. On the one hand, graphene provides a reaction attachment point for the nucleation growth of magnesium grains, and on the other hand, graphene restricts the MgH2@Graphene particles. Meanwhile, graphene restricts the mobility of MgH2 grains, making them difficult to agglomerate, thus causing the refinement of MgH2 grains while inhibiting the agglomeration of MgH2.
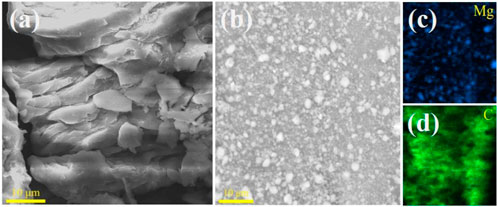
FIGURE 2. SEM images for (A) MgH2, (B) MgH2@Graphene, and (C,D) corresponding elemental mapping images of Mg and (C).
The scanning electron microscopy analysis of the MgH2@Ti2C composite is shown in Figures 3A–D, and the scanning electron microscopy analysis of the MgH2@Nb2C composite is shown in Figures 3E–H. The presence of Ti and Nb elements is visible at a magnification of ×5,000, confirming the presence of the transition metal Ti as well as the metal Niobium. The SEM images also reveal a more uniform distribution of the composite particle sizes without agglomeration, and the corresponding elemental distribution maps indicate a homogeneous distribution of both Ti and C as well as Nb and C on the Mg substrate. In summary, the introduction of graphene-based transition metals Ti and Nb is feasible and the MgH2@Ti2C and MgH2@Nb2C composites were successfully prepared.
Improved hydrogen storage properties
Figure 4A shows the temperature-programmed desorption curves of MgH2@Nb2C composite, MgH2@Graphene composite and pure MgH2, from which the starting hydrogen release temperature of pure MgH2 is 305°C and the ending hydrogen release temperature is 425°C, while the starting hydrogen release temperature of MgH2@Graphene composite prepared by mechanical ball milling is 260°C and the ending hydrogen release temperature is 405°C. To derive the minimum temperature at which the maximum hydrogen release rate is achieved, we differentiate Figure 4A to obtain Figure 4B, from which we know that the minimum temperature at which the maximum hydrogen release rate of pure MgH2 is 360°C, the minimum temperature of the maximum hydrogen release rate of MgH2@Graphene composite is at 330°C, while the MgH2@Nb2C composite is 310°C, which is 50°C lower than that of pure MgH2, indicating that the addition of transition metal niobium on top of graphene can effectively reduce the temperature of the maximum hydrogen release rate. In summary, the addition of the two-dimensional layered metal carbide Nb2C can further reduce the hydrogen release temperature of pure MgH2 compared to that of single graphene. The two-dimensional layered metal carbide Nb2C has a remarkable catalytic effect on MgH2 hydrogen storage properties. To further validate the catalytic effect of two-dimensional carbon materials with different metal sites on improving the hydrogen storage of MgH2, we chose the two-dimensional layered metal carbide Ti2C as the catalyst for MgH2. Figure 4C shows the temperature-programmed desorption curves of MgH2@Ti2C and MgH2@Nb2C composites. It can be seen from the figure that the initial desorption temperature of the MgH2@Nb2C composite is 250°C and the termination temperature is 350°C. The initial desorption temperature of MgH2@Ti2C is 235°C and the termination temperature is 325°C, indicating that under the same experimental conditions, the lower desorption temperature of MgH2@Ti2C and the better catalytic performance of Ti2C. To obtain the minimum temperature of the maximum discharge rate, we differentiated Figure 4C and obtained Figure 4D. From Figure 4D, the minimum temperature of MgH2@Nb2C composite is 310°C, and 290°C for MgH2@Ti2C composite, the minimum temperature of the maximum discharge rate is reduced by 20°C, indicating that MgH2@Ti2C has faster hydrogenation rate, and the catalytic performance is better. In conclusion, the 2-dimensional layered metal carbide Ti2C can further reduce the hydrogenation temperature of MgH2 and make its discharge rate faster than the single graphene.
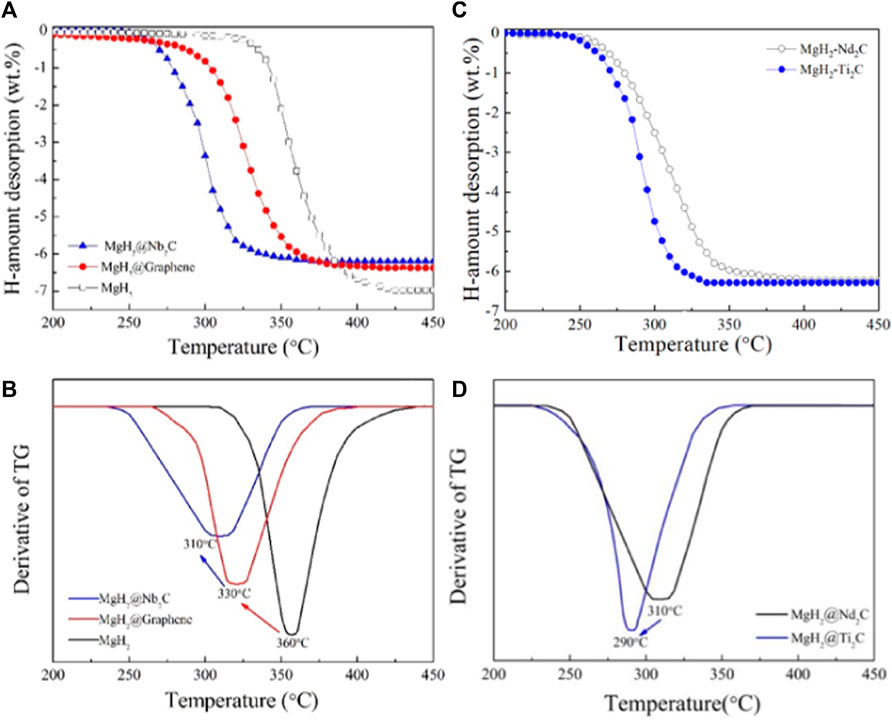
FIGURE 4. (A) The temperature—programmed desorption curves for the MgH2@Nb2C (B) corresponding first order derivative graph, (C) The temperature-programmed desorption curves for the MgH2@Ti2C (D) corresponding first order derivative graph.
Figure 5A shows the isothermal hydrogen absorption curves of MgH2@Nb2C composites and MgH2@Graphene composites at different temperatures. It can be seen from the figure that MgH2 with Graphene addition has almost no hydrogen absorption behavior at 100°C and only 1 wt% at 150°C for 60 min. In contrast, MgH2@Nb2C composites, at 100°C for 20 min, absorb up to 3 wt% of hydrogen, which is much higher than MgH2@Graphene. Not only that, the hydrogen absorption of MgH2@Nb2C composite at 150°C is much higher than that of MgH2@Graphene. MgH2@Nb2C composites showed a higher hydrogen uptake rate and hydrogen uptake at 150°C than MgH2@Graphene composites at 200°C. It shows that the addition of two-dimensional layered metal carbide Nb2C leads to the better catalytic performance of MgH2 in terms of hydrogen uptake rate and hydrogen uptake capacity compared with the addition of graphene only. Figure 5B shows the isothermal hydrogen absorption curves at different temperatures for both the MgH2@Ti2C composite and the MgH2@Nb2C composite. It can be seen from the figure that the hydrogen uptake of MgH2@Ti2C composites and MgH2@Nb2C composites remained almost the same at 200°C for 60 min, but the hydrogen uptake rate of MgH2@Ti2C was somewhat faster; but at 150°C and 100°C, MgH2@Ti2C is not only faster and has higher hydrogen absorption capacity than MgH2@Nb2C.The isothermal hydrogen absorption properties of the MgH2@Ti2C composite material and the MgH2@Nb2C composites can differ significantly at different temperatures. Figure 5C shows the isothermal hydrogen release test of MgH2@Nb2C composite and MgH2@Graphene composite at different temperatures. It can be seen from the figure, the MgH2@Graphene composite has almost no hydrogen discharge behavior at 250°C, only 1 wt% at 280°C and 60 min, and only 3.4 wt% at 300°C and 60 mi. Relatively speaking, MgH2@ Nb2C composite has reached 4 wt% in 250°C and 50 min, which has far exceeded MgH2@Graphene in the discharge rate and amount. At 280°C and 300°C, MgH2@Nb2C composite is the same, reaching 6.4 wt%, However, the rate of hydrogen release accelerates substantially with increasing temperature. It is shown that the addition of the 2D layered metal carbide Nb2C improves MgH2 at the release rate and release capacity compared to only 2D graphene. Similarly, we conducted isothermal hydrogen desorption tests on MgH2@Nb2C and MgH2@Ti2C composites at different temperatures. The results are shown in Figure 5D. It can be seen from the figure that, within 60 min at 270°C and 300°C, the hydrogen emission amount of MgH2@Ti2C and MgH2@Nb2C is almost the same, but the hydrogen emission rate of MgH2@Ti2C is faster than that of MgH2@Nb2C. However, at 250°C, MgH2@Ti2C has a higher dehydrogenation rate and capacity than MgH2@Nb2C. The isothermal dehydrogenation properties of MgH2@Nb2C and MgH2@Ti2C are different at different temperatures.
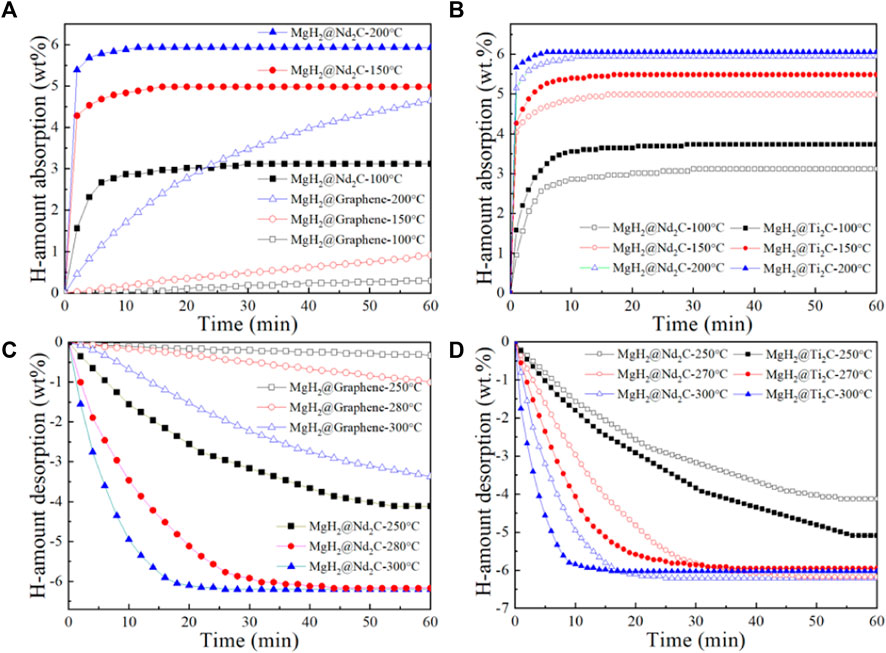
FIGURE 5. (A) MgH2@Nb2C, (B) MgH2@Ti2C isothermal hydrogen absorption curves at different temperatures, (C) MgH2@Nb2C, MgH2@Ti2C (D) Isothermal hydrogen desorption curves at different temperatures.
To verify the advantages of MgH2@Ti2C, MgH2@Nb2C, and MgH2@Graphene in terms of kinetic performance, we also used the JMAK (Johnson−Mehl−Avrami−Kolmogorov) model (Shahi et al., 2014) to calculate their apparent hydrogen release activation energies. Based on the JMAK model, the desorption kinetics can be expressed by the following equation:
where α is the reaction fraction corresponding to the beginning and completion of the reaction, η is the Avrami index of the order of the reaction, k is the rate constant, and t is time. Sample data for experiment, ln [−ln (1−α)] as a function of ln(t) figure in different temperature of each curve is linear, as shown in Figures 6A,B,D,E. After calculating the rate constant k, the apparent activation energy (Ea) of the dehydrogenation process is calculated according to the Arrhenius equation:
where k0 is pre-exponential factor, R is gas constant and T is absolute temperature. From the slopes of the straight lines as shown in Figures 6C,F. The Ea of MgH2@Nb2C is 112.19 kJ/mol, 11.79 kJ/mol lower than that of MgH2@Graphene (123.98 kJ/mol). The calculated Ea of MgH2@Nb2C is 111.49 kJ/mol, and the Ea of MgH2@Ti2C was 86.48 kJ/mol, decreasing by 25.01 kJ/mol. The results indicate that the two-dimensional layered metal carbides Ti2C and Nb2C reduce the apparent activation energy of MgH2 compared with the addition of only two-dimensional graphene. More importantly, the 2D carbon materials with different metal sites improve the hydrogen storage of MgH2 catalytic effects showed significant differences.
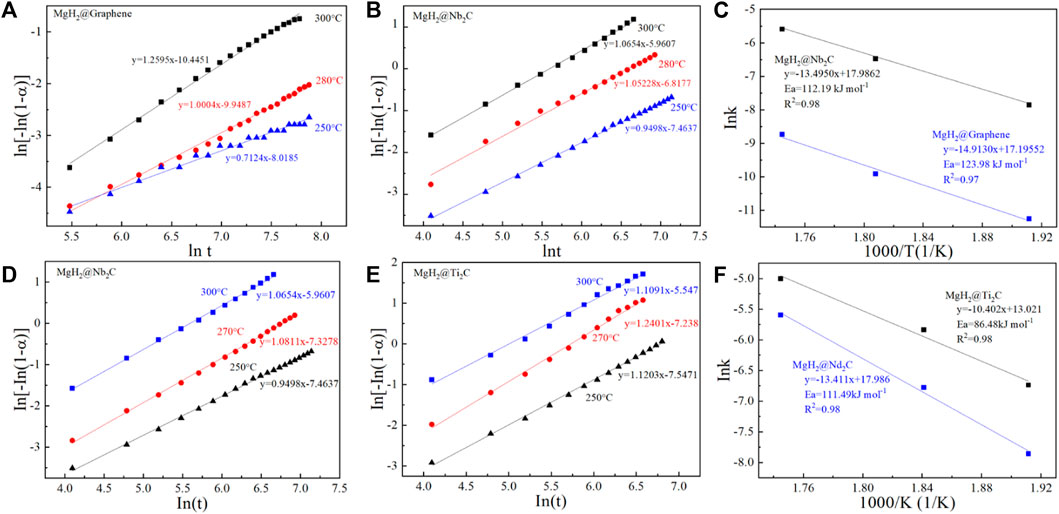
FIGURE 6. JMAK plots for the hydrogen desorption at different temperature (A) MgH2@Graphene, (B) MgH2@Nb2C and (C) corresponding Arrhenius plots, JMAK plots for the hydrogen desorption at different temperature (D) MgH2@Nb2C, (E) MgH2@Ti2C, and (F) corresponding Arrhenius plots.
Figures 7A,B show the isothermal hydrogen absorption and discharge cycle curves for MgH2@Nb2C and MgH2@Ti2C, where the hydrogen absorption temperature is 300°C and the hydrogen absorption pressure is 4 MPa, and the hydrogen discharge temperature is 300°C and the hydrogen discharge pressure is 10 Pa. The initial hydrogen discharge is 6.41 wt%, 6.39 wt%, and the final hydrogen absorption is 6.09 wt% and 6.19 wt%, respectively. The capacity retention was as high as 95% and 97% in isothermal hydrogen absorption and discharge cycles, respectively, with almost no decay, indicating that both MgH2@Nb2C and MgH2@Ti2C composites have good stability. The comparison of the two shows that the catalytic effect of transition metals and graphene on MgH2 is synergistic, and the catalytic effect of Ti is better than that of Nb, which makes the hydrogen uptake and discharge and kinetic performance of MgH2@Ti2C better than that of MgH2@Nb2C.
Difference in catalytic mechanisms of 2D carbon materials
The chemical valence state changes of Ti and C as well as Nb and C in the process of hydrogen absorption and release can be further analyzed by XPS. Figure 8A shows the XPS spectrum of Nb 3d with six peaks of 203.7/205.4, 204.0/207.0, and 206.8/209 eV for catalyst Nb2C. After the ball milling reaction with MgH2, it was found that both Nb2+ and Nb4+ peaks disappeared, Nb-C failed to disappear completely, and a new peak Nb0 was generated (corresponding to 202.6/205.5 eV), which indicates that the catalyst Nb2C produced metallic Nb during the high-energy ball milling process. However, the chemical price of the MgH2@Nb2C composite did not change during the first hydrogenation of Nb, but after the first hydrogen uptake, the Nb0 peak (corresponding to 202.6/205.5 eV) disappeared and a new Nb-H peak (corresponding to 203.2/206.1 eV) appeared. This indicates that the MgH2@Nb2C composite is hydrogenated by metal Nb to NbHX after the first hydrogen uptake. Similarly, Figure 8C shows the XPS spectrum of Ti 2p, which also illustrates that the MgH2@Ti2C composite is hydrogenated by metal Ti to TiHX after the first hydrogen uptake. Figures 8B,D shows the C 1s XPS spectrum. The results show that between the Ti2C and MgH2@Ti2C composites in different states and between the Nb2C and MgH2@Nb2C composites, only the Nb-C bond is broken with Ti-C and there is no change in C-C, indicating that the carbon layer is not decomposed. Investigation of the periodic table of elements shows that the electronegativity of Mg is ∼1.31 and that of H is ∼2.2, while the electronegativity of Ti is ∼1.54 and that of Nb is ∼1.60, between Mg and H, which can effectively weaken the Mg-H bond. In summary, after the reaction decomposition of catalyst Ti2C as well as Nb2C with MgH2, the zero-valent titanium/niobium grows in situ in Mg grains, and the carbon layer without decomposition will further form graphene, which can provide nucleation and growth of nano-MgH2, making the distribution of nano-MgH2 grains relatively uniform and effectively inhibiting the growth and agglomeration of MgH2 grains in the hydrogen cycle.
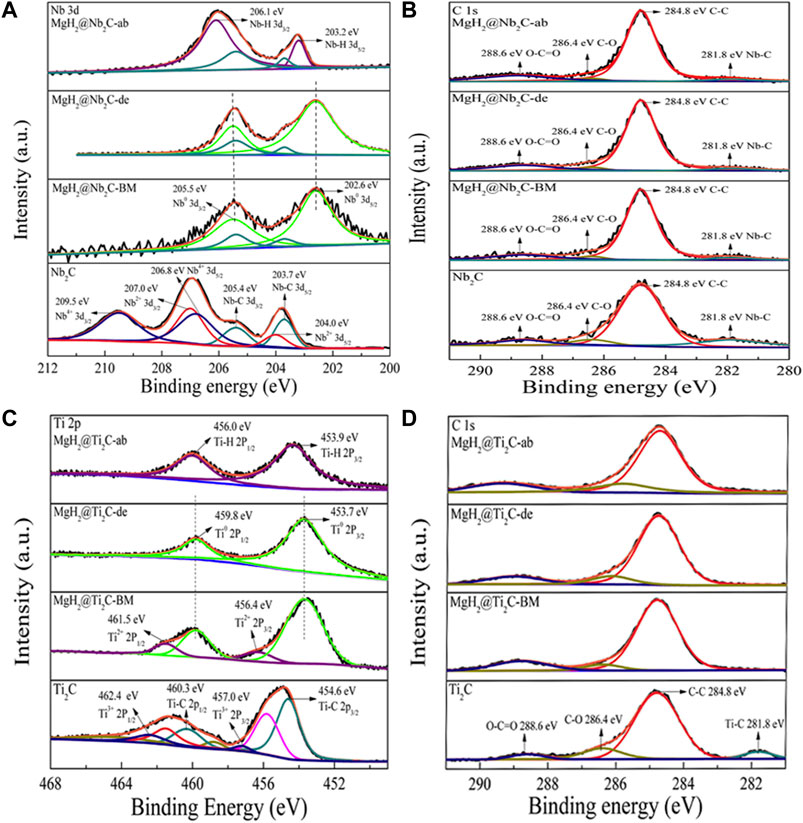
FIGURE 8. (A) Nb 3d and (B) C 1s XPS spectra of Nb2C and MgH2@ Nb2C samples at different states, (C) Ti 2p and (D) C 1s XPS spectra of Ti2C and MgH2@ Ti2C samples at different states.
To further explain the changes in the chemical composition of each metal and graphite during the hydrogen uptake and release process of MgH2@M2C (M is metal) composites, thus revealing the catalytic effect of 2D carbon materials with different metal sites on improving the hydrogen storage of MgH2, we combined XPS to map the flow of the materials in the hydrogen uptake and release stage. As shown in Figure 9, hydrogen stage, weakened MgH2 will preferentially decompose into magnesium grains and free hydrogen atoms, free hydrogen atoms on the MHX surface reunited into H2 molecules, then MHX will decompose into metal Nb and hydrogen atoms, also hydrogen atoms will be combined into H2 molecules. In contrast, in the hydrogen absorption phase, metal Nb first reacts with H2 to form MHX, the H2 molecule dissociates into hydrogen atoms on the surface of MHX, free hydrogen atoms react with magnesium through the niobium supply channel to form magnesium hydrogen bonds, and the formed MgH2 meeting further nucleates and grows into MgH2 grains.
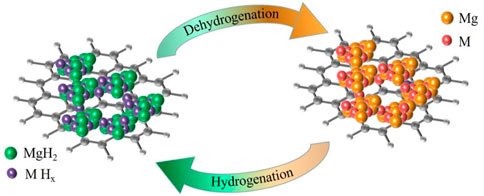
FIGURE 9. MgH2@M2C theoretical schematic diagram in the state of complete hydrogen absorption and complete hydrogen release.
Conclusion
In this paper, MgH2@Graphene, MgH2@Nb2C and MgH2@Ti2C composites were successfully prepared by ball milling reaction of two-dimensional layered metal carbides Nb2C and Ti2C with MgH2, and their hydrogen storage properties were tested. The results show that the apparent activation energy of MgH2@Nb2C composite is ∼111.49 kJ/mol, and that of MgH2@Ti2C composite is ∼86.48 kJ/mol, which is lower than ∼123.98 kJ/mol of MgH2@Graphene composite. In 30 cycles of hydrogen absorption and desorption, the hydrogen desorption capacity of MgH2@Ti2C composite is as high as 97.0%, and that of MgH2@Nb2C composite is as high as 95.0%, indicating that the addition of Ti2C has lower apparent activation energy and better cycle stability than that of Nb2C. The tiny M (Ti/Nb) crystals can act as a channel to facilitate hydrogen transport and improve the dehydrogenation performance of MgH2. The small nanocrystals distributed in the composites are a way to facilitate hydrogen transport and improve the dehydrogenation performance, while M2C is only a catalyst and not a reactant; the multivalent M atoms on the surface act as intermediates for electron movement between H− and Mg2+, making dehydrogenation easier. This also reveals the catalytic effect of 2D carbon materials with different metal sites on improving hydrogen storage of MgH2.
Data availability statement
The original contributions presented in the study are included in the article/supplementary material, further inquiries can be directed to the corresponding author.
Author contributions
KR carried out material preparation, sample characterization, performance testing, and wrote the paper. BW discussed the results and revised the manuscript in part.
Funding
This work was financially supported by the National College Students’ Innovation and Entrepreneurship Training Program of China (No. 202110360024).
Acknowledgments
I thank Prof. Yongtao Li for his assistance with supervising the experiments and revising the manuscript.
Conflict of interest
The authors declare that the research was conducted in the absence of any commercial or financial relationships that could be construed as a potential conflict of interest.
Publisher’s note
All claims expressed in this article are solely those of the authors and do not necessarily represent those of their affiliated organizations, or those of the publisher, the editors and the reviewers. Any product that may be evaluated in this article, or claim that may be made by its manufacturer, is not guaranteed or endorsed by the publisher.
References
Chen, P., and Zhu, M. (2008). Recent progress in hydrogen storage. Mat. TodayKidlingt. 11, 36–43. doi:10.1016/S1369-7021(08)70251-7
Chen, X., Zou, J., Zeng, X., and Ding, W. (2016). Hydrogen storage in Mg2Fe(Ni)H6 nanowires synthesized from coarse-grained Mg and nano sized γ-Fe(Ni) precursors. Int. J. Hydrogen Energy 41, 14795–14806. doi:10.1016/j.ijhydene.2016.06.024
Ding, X., Li, Y., Fang, F., Sun, D., and Zhang, Q. (2017). Hydrogen-induced magnesium–zirconium interfacial coupling: Enabling fast hydrogen sorption at lower temperatures. J. Mat. Chem. A 5, 5067–5076. doi:10.1039/C7TA00460E
Edalati, K., Uehiro, R., Ikeda, Y., Li, H.-W., Emami, H., Filinchuk, Y., et al. (2018). Design and synthesis of a magnesium alloy for room temperature hydrogen storage. Acta Mat. 149, 88–96. doi:10.1016/j.actamat.2018.02.033
Gao, H., Liu, Y., Zhu, Y., Zhang, J., and Li, L. (2019). Catalytic effect of sandwich-like Ti3C2/TiO2(A)-C on hydrogen storage performance of MgH2. Nanotechnology 31, 115404. doi:10.1088/1361-6528/ab5979
Gao, H., Shao, Y., Shi, R., Liu, Y., Zhu, J., Liu, J., et al. (2020). Effect of few-layer Ti3C2TX supported nano-Ni via self-assembly reduction on hydrogen storage performance of MgH2. ACS Appl. Mat. Interfaces 12, 47684–47694. doi:10.1021/acsami.0c15686
Grochala, W., and Edwards, P. P. (2004). Thermal decomposition of the non-interstitial hydrides for the storage and production of hydrogen. Chem. Rev. 104, 1283–1316. doi:10.1021/cr030691s
Hanada, N., Ichikawa, T., Hino, S., and Fujii, H. (2006). Remarkable improvement of hydrogen sorption kinetics in magnesium catalyzed with Nb2O5. J. Alloys Compd. 420, 46–49. doi:10.1016/j.jallcom.2005.08.084
Huang, T., Huang, X., Hu, C., Wang, J., Liu, H., Xu, H., et al. (2021). MOF-derived Ni nanoparticles dispersed on monolayer MXene as catalyst for improved hydrogen storage kinetics of MgH2. Chem. Eng. J. 421, 127851. doi:10.1016/j.cej.2020.127851
Jain, I. P., Lal, C., and Jain, A. (2010). Hydrogen storage in Mg: A most promising material. Int. J. Hydrogen Energy 35, 5133–5144. doi:10.1016/j.ijhydene.2009.08.088
Khan, D., Zou, J., Pan, M., Ma, Z., Zhu, W., Huang, T., et al. (2019). Hydrogen storage properties of nanostructured 2MgH2-Co powders: The effect of high-pressure compression. Int. J. Hydrogen Energy 44, 15146–15158. doi:10.1016/j.ijhydene.2019.04.077
Li, S., Jena, P., and Ahuja, R. (2006). Dehydrogenation mechanism in catalyst-activated MgH2. Phys. Rev. B 74, 132106. doi:10.1103/PhysRevB.74.132106
Lillo-Ródenas, M. A., Guo, Z. X., Aguey-Zinsou, K. F., Cazorla-Amorós, D., and Linares-Solano, A. (2008). Effects of different carbon materials on MgH2 decomposition. Carbon 46, 126–137. doi:10.1016/j.carbon.2007.10.033
Liu, T., Ma, X., Chen, C., Xu, L., and Li, X. (2015). Catalytic effect of Nb nanoparticles for improving the hydrogen storage properties of Mg-based nanocomposite. J. Phys. Chem. C 119, 14029–14037. doi:10.1021/acs.jpcc.5b03442
Lotoskyy, M., Denys, R., Yartys, V. A., Eriksen, J., Goh, J., Nyamsi, S. N., et al. (2018). An outstanding effect of graphite in nano-MgH2–TiH2 on hydrogen storage performance. J. Mat. Chem. A 6, 10740–10754. doi:10.1039/C8TA02969E
Lu, C., Zou, J., Zeng, X., and Ding, W. (2017). Hydrogen storage properties of core-shell structured Mg@TM(TM = Co, V) composites. Int. J. Hydrogen Energy 42, 15246–15255. doi:10.1016/j.ijhydene.2017.04.063
Ma, T., Isobe, S., Wang, Y., Hashimoto, N., and Ohnuki, S. (2013). Nb-gateway for hydrogen desorption in Nb2O5 catalyzed MgH2 nanocomposite. J. Phys. Chem. C 117, 10302–10307. doi:10.1021/jp4021883
Ma, X., Xie, X., Liu, P., Xu, L., and Liu, T. (2017). Synergic catalytic effect of Ti hydride and Nb nanoparticles for improving hydrogenation and dehydrogenation kinetics of Mg-based nanocomposite. Prog. Nat. Sci. Mater. Int. 27, 99–104. doi:10.1016/j.pnsc.2016.12.013
Ma, Z., Liu, J., Zhu, Y., Zhao, Y., Lin, H., Zhang, Y., et al. (2020). Crystal-facet-dependent catalysis of anatase TiO2 on hydrogen storage of MgH2. J. Alloys Compd. 822, 153553. doi:10.1016/j.jallcom.2019.153553
Nabgan, W., Tuan Abdullah, T. A., Mat, R., Nabgan, B., Gambo, Y., Ibrahim, M., et al. (2017). Renewable hydrogen production from bio-oil derivative via catalytic steam reforming: An overview. Renew. Sustain. Energy Rev. 79, 347–357. doi:10.1016/j.rser.2017.05.069
Novoselov, K. S., Geim, A. K., Morozov, S. V., Jiang, D., Zhang, Y., Dubonos, S. V., et al. (2004). Electric field effect in atomically thin carbon films. Science 306, 666–669. doi:10.1126/science.1102896
Ren, C., Fang, Z. Z., Zhou, C., Lu, J., Ren, Y., Zhang, X., et al. (2014). In situ X-ray diffraction study of dehydrogenation of MgH2 with Ti-based additives. Int. J. Hydrogen Energy 39, 5868–5873. doi:10.1016/j.ijhydene.2014.01.152
Santos, S. F., Ishikawa, T. T., Botta, W. J., and Huot, J. (2014). MgH2+FeNb nanocomposites for hydrogen storage. Mat. Chem. Phys. 147, 557–562. doi:10.1016/j.matchemphys.2014.05.031
Shahi, R. R., Bhatnagar, A., Pandey, S. K., Dixit, V., and Srivastava, O. N. (2014). Effects of Ti-based catalysts and synergistic effect of SWCNTs-TiF3 on hydrogen uptake and release from MgH2. Int. J. Hydrogen Energy 39, 14255–14261. doi:10.1016/j.ijhydene.2014.03.183
Shao, H., He, L., Lin, H., and Li, H.-W. (2018). Progress and trends in magnesium-based materials for energy-storage research: A review. Energy Technol. 6, 445–458. doi:10.1002/ente.201700401
Si, T.-Z., Zhang, X.-Y., Feng, J.-J., Ding, X.-L., and Li, Y.-T. (2021). Enhancing hydrogen sorption in MgH2 by controlling particle size and contact of Ni catalysts. Rare Met. 40, 995–1002. doi:10.1007/s12598-018-1087-x
Wang, K., Wu, G., Cao, H., Li, H., and Zhao, X. (2018). Improved reversible dehydrogenation properties of MgH2 by the synergetic effects of graphene oxide-based porous carbon and TiCl3. Int. J. Hydrogen Energy 43, 7440–7446. doi:10.1016/j.ijhydene.2018.02.195
Xia, G., Tan, Y., Chen, X., Sun, D., Guo, Z., Liu, H., et al. (2015). Monodisperse magnesium hydride nanoparticles uniformly self-assembled on graphene. Adv. Mat. 27, 5981–5988. doi:10.1002/adma.201502005
Yu, X., Tang, Z., Sun, D., Ouyang, L., and Zhu, M. (2017). Recent advances and remaining challenges of nanostructured materials for hydrogen storage applications. Prog. Mat. Sci. 88, 1–48. doi:10.1016/j.pmatsci.2017.03.001
Zhang, M., Xiao, X., Wang, X., Chen, M., Lu, Y., Liu, M., et al. (2019). Excellent catalysis of TiO2 nanosheets with high-surface-energy {001} facets on the hydrogen storage properties of MgH2. Nanoscale 11, 7465–7473. doi:10.1039/C8NR10275A
Zhang, Q. A., Liu, D. D., Wang, Q. Q., Fang, F., Sun, D. L., Ouyang, L. Z., et al. (2011). Superior hydrogen storage kinetics of Mg12YNi alloy with a long-period stacking ordered phase. Scr. Mat. 65, 233–236. doi:10.1016/j.scriptamat.2011.04.014
Zhou, C., Bowman, R. C., Fang, Z. Z., Lu, J., Xu, L., and Sun, P. (2019). Amorphous ticu based additives for improving hydrogen storage properties of magnesium hydride. ACS Appl. Mater Interfaces 11, 38868–38879. doi:10.1021/acsami.9b16076
Zhu, L., Liao, Y., Zhong, Y., Cui, J., Wang, D., and Wang, K. (2020a). Improved reversible dehydrogenation performance of MgH2 by the synergistic effects of porous boron nitride and NbF5. J. Energy Storage 29, 101418. doi:10.1016/j.est.2020.101418
Zhu, W., Panda, S., Lu, C., Ma, Z., Khan, D., Dong, J., et al. (2020b). Using a self-assembled two-dimensional MXene-based catalyst (2D-Ni@Ti3C2) to enhance hydrogen storage properties of MgH2. ACS Appl. Mat. Interfaces 12, 50333–50343. doi:10.1021/acsami.0c12767
Keywords: hydrogen storage, MgH2, MXene, 2D materials, catalysis
Citation: Ren K and Wang B (2022) Revealing the effect of 2D carbides with different metal sites for improving hydrogen storage in MgH2. Front. Chem. 10:1000408. doi: 10.3389/fchem.2022.1000408
Received: 22 July 2022; Accepted: 26 August 2022;
Published: 21 September 2022.
Edited by:
Ruguang Ma, Suzhou University of Science and Technology, ChinaCopyright © 2022 Ren and Wang. This is an open-access article distributed under the terms of the Creative Commons Attribution License (CC BY). The use, distribution or reproduction in other forums is permitted, provided the original author(s) and the copyright owner(s) are credited and that the original publication in this journal is cited, in accordance with accepted academic practice. No use, distribution or reproduction is permitted which does not comply with these terms.
*Correspondence: Kaixiang Ren, MjU3NTA5NTQwMEBxcS5jb20=