- 1Guizhou Academy of Tobacco Science, Upland Flue-cured Tobacco Quality and Ecology Key Laboratory, CNTC, Guiyang, China
- 2Guizhou Tobacco Quality Supervision and Test Station, Guiyang, China
- 3College of Biological, Chemical Science and Engineering, Jiaxing University, Jiaxing, China
- 4State Key Laboratory Breeding Base of Green Pesticide and Agricultural Bioengineering, Key Laboratory of Green Pesticide and Agricultural Bioengineering, Ministry of Education, State-Local Joint Laboratory for Comprehensive Utilization of Biomass, Center for Research and Development of Fine Chemicals, Guizhou University, Guiyang, China
With the increasing demand for fossil fuels, decreasing fossil fuel reserves and deteriorating global environment, humanity urgently need to explore new clean and renewable energy to replace fossil fuel resources. Biodiesel, as an environmentally friendly fuel that has attracted considerable attention because of its renewable, biodegradable, and non-toxic superiority, seems to be a solution for future fuel production. Tobacco (Nicotiana tabacum L.), an industrial crop, is traditionally used for manufacturing cigarettes. More importantly, tobacco seed is also widely being deemed as a typical inedible oilseed crop for the production of second-generation biodiesel. Advancements in raw material and enhanced production methods are currently needed for the large-scale and sustainable production of biodiesel. To this end, this study reviews various aspects of extraction and transesterification methods, genetic and agricultural modification, and properties and application of tobacco biodiesel, while discussing the key problems in tobacco biodiesel production and application. Besides, the proposals of new ways or methods for producing biodiesel from tobacco crops are presented. Based on this review, we anticipate that this can further promote the development and application of biodiesel from tobacco seed oil by increasing the availability and reducing the costs of extraction, transesterification, and purification methods, cultivating new varieties or transgenic lines with high oilseed contents, formulating scientific agricultural norms and policies, and improving the environmental properties of biodiesel.
Introduction
The industrial revolution results in the widespread use of fossil fuels, which constitute 80% of the energy supply. Additionally, various fossil fuel derivatives are also widely used in the production of industrial and agricultural products. In the past 2 decades, global fossil fuel consumption has continued to grow by 48.7%. According to the reports in 2019, the corresponding consumption reached 136,761 TW-hours (TWh), and oil, natural gas and coal were 53,620, 39,292 and 43,849, respectively (Smil, 2017). The global carbon balance has been broken with massive amounts of CO2 emitted from the use of fossil fuels. The carbon surplus of the atmosphere is the major driving force for the modern global environment and climate change issues such as global warming, extreme weather, etc. (Pao and Tsai, 2010; Pan et al., 2020). To mitigate the negative consequences of excess CO2 emissions, biofuels derived from waste have been recognized as a substitute for the traditional fossil fuels (Kim et al., 2019). They are carbon neutral, inexpensive and an environmentally friendly and renewable source of energy (Hill et al., 2006). Among the various types of biofuels, biodiesel has been characterized by lower agricultural inputs and more efficient conversion (Hill et al., 2006). Biodiesel has been widely commercialized due to its relatively mature production technology, simple and universal platform (Figure 1) (Liu et al., 2019). It is inferred that the global production of biodiesel will reach 46 billion liters in 2025 (Zhang et al., 2017).
Although biodiesel brings many benefits, the key basis of biodiesel production is feedstocks, and feedstocks are determined to play the most important role in the biodiesel value chain. The feedstock should fulfill three main requirements: low production costs, large-scale production and appropriate fatty acid content/composition for biodiesel (Mishra and Goswami, 2018). Moreover, their oil content and composition are highly important indicators, such as the feedstocks rich in monounsaturated fatty acids (MUFAs) are desirable for biodiesel but the composition of the saturated fatty acids (SFAs) is also shown to be of great importance (Stansell, et al., 2012). According to the source of feedstocks, we can categorize them into three biodiesel generations. First-generation biodiesel is currently being widely produced and is extracted from various feedstocks, such as edible rapeseed oil, sunflower oil, palm oil, soybeans, and animal fats. Second- and third-generation biodiesels, only a few of which are close to large-scale commercialization, are extracted from inedible lipid feedstocks such as terrestrial biomass (i.e., breeding crops and transgenic crops) and aquatic biomass (i.e., microalgae and macroalgae) (Vauhkonen et al., 2009; Chung et al., 2020; Mizik and Gyarmati, 2021). However, biodiesel produced from edible oils is more expensive than conventional fossil diesel. Biodiesel has over double the price of diesel fuel. The major economic factor to consider for input costs of biodiesel production is the feedstock, which is about 80% of the total operating cost (Demirbas, 2007). On the other hand, food shortages may occur due to the use of large amounts of edible oils in producing biodiesel, especially in poor countries. These drawbacks can be overcome by using inedible oils for biodiesel production like Aleurites trisperma, bitter almond, Brucea javanica seeds, Argemone Mexicana, wild mustard (Brassica Juncea L.) seed, and tobacco (Nicotiana tabacum L.) seed (Arumugam et al., 2017; Muthukumaran et al., 2017; Jain, 2019; Wang et al., 2019; Adepoju, 2020; Tlili et al., 2020). Generally, tobacco, Nicotiana tabacum, which is an annual plant in the Solanaceae family with leaves harvested to manufacture cigarettes, is widespread in China and North and South America, and the leaves are commonly harvested. In recent years, tobacco has been cultivated in approximately 130 countries and covered almost 3.4 million hectares in fields, wherein China alone provides over 40% of the world’s production (García-Martínez et al., 2017; Berbe and Matyka, 2020; Wu et al., 2020). It is reported that the N. tabacum varieties with the highest seed yields reach 1,171 kg per hectare, which corresponds to 432.9 kg of oil based on an effective oil biosynthesis mechanism for tobacco (Zdremnan and Zdremnan, 2006; García-Martínez et al., 2017). The oil content of tobacco seed often ranges from 30 to 43 wt% and consists mainly of unsaturated fatty acids (Giannelos et al., 2002; Banković-Ilić et al., 2012; Khan et al., 2014; Hu et al., 2020; Rajan et al., 2021). The major fatty acids in tobacco seed oil are stearic acid (2.1–3.3%), palmitic acid (8.72–12.3%), oleic acid (9.97–13.49%), and linoleic acid (64.38–79.0%), respectively (Koiwai et al., 1983; Mukhtar et al., 2007; Tian et al., 2020). As a kind of second-generation biodiesel, tobacco-based biodiesel production is very promising. Therefore, in this review, we elucidate the extraction and transesterification methods, genetic modification, properties and application, and agricultural strategies applied in tobacco biodiesel production, and then discuss the key problems and prospects for biodiesel production from tobacco.
Extraction Methods of Tobacco Seed Oil for Biodiesel Production
The tobacco seed oil has been traditionally extracted by mechanical pressing and solvent extraction, mainly with nonpolar solvents. Mechanical pressing, a relatively environmentally friendly extraction method, has been widely applied for the production of high-quality edible oils (Sannino et al., 2017). The whole process includes sample preparation and mechanical pressing stages followed by a solvent extraction procedure to recover the oil located in the press cake (Geow et al., 2021). Seed preparation involving preheating and microwaving destroy or soften the cellular structure of tobacco seeds, which significantly increases the oil yield (Sannino et al., 2017). The pressing equipment parameters including feeding rate, restriction dye diameter, temperature and rotation speed, affect the tobacco seed oil yield. The evaluation of extraction methods in the literature based on the oil yield provided by Soxhlet or accelerated solvent extraction (ASE) due to high oil recovery.
Oil extraction yield obtained from mechanical pressing were usually about 80% (w/w) of ASE, but Stanisavljević et al. reported a higher oil extraction yield, which may be related to pressing equipment type (Stanisavljević et al., 2009; Faugno et al., 2016; Sannino et al., 2017). The characteristics of several common methods used in tobacco seed oil extraction is shown in Table 1.
Soxhlet extraction is a classical method for extracting tobacco seed oil with high extraction efficiency (Maestri and Guzmán, 1995; Zlatanov, et al., 2007). Compared with Soxhlet extraction, ASE with a similar oil yield consumed less solvent and time (Faugno et al., 2016; Sannino et al., 2017). However, the milder extraction operations, such as ultrasonic extraction and maceration, showed a lower extraction efficiency (Stanisavljević et al., 2007b and 2009). The solvent extraction efficiency from tobacco seed oil depends on the comminution of the seed, the solvent, the seed-to-solvent ratio, and the extraction time and temperature. Specifically, milling greatly improves extraction efficiency by breaking down tobacco seed cells and augmenting the interfacial area for mass transfer. The oil yield obtained from ground tobacco seeds was shown to be 10 times that of native seeds during ultrasonic extraction, even though the oil yield of tobacco seeds repeatedly grounded was 6 times higher than that of native seed extracted by mechanical pressing (Stanisavljević et al., 2009). Supercritical fluid extraction was also used to extract tobacco seed oil with high recovery and without any residual organic solvents (Stanisavljević et al., 2007b; Majdi et al., 2012; Ashraf-Khorassani et al., 2015). It is worth emphasizing that pressure is the most important factor in supercritical fluid extraction. These extractions of tobacco seed oil mainly focus on laboratory- or small-scale operations. However, application at the industrial scale is still limited to date. Future research should study and develop more environmentally friendly extraction methods suitable for the large-scale production of tobacco seed oil.
Transesterification of Biodiesel in Tobacco Seed Oil
Also known as alcoholysis, transesterification is a process involving the breakage of old ester bonds and the formation of new ester bonds with another alcohol. This process is similar to the mechanism of hydrolysis (Srivastava and Prasad, 2000). Transesterification reactions have been widely applied to convert triglycerides to fatty acid methyl esters or fatty acid ether esters with reduced oil viscosity. The typical reaction with common methanol is shown in Figure 2 and is also called methanolysis. The transesterification reactions of the process are reversible, and the presence of a suitable catalyst can accelerate the forward conversion reaction. Although acid and enzyme have also been applied in transesterification, base is the most commonly used catalyst and it mainly consists of three steps. These consist of alkoxide formation, nucleophilic attack at the carbonyl carbon, and the formation of the alkyl ester, which needs to be repeated three times for TGAs. The mechanism of acid-catalyzed transesterification is a little different and consists of carbocation formation, nucleophilic attack of the alcohol, and the formation of the alkyl ester (Ma and Hanna, 1999; Meher et al., 2006). The transesterification process determines the cost and complexity of tobacco seed biodiesel production.
Transesterification efficiency is affected by various critical factors, such as the influence of free fatty acids (FFAs) and water, type and amount of catalyst, the molar ratio of alcohol to oil and type of alcohol, transesterification time and temperature, uniformity of mixing, and whether or not organic co-solvents are used, depending upon reaction conditions. The catalyst chosen is ultimately critical and determines the degree of influence by other factors (Meher et al., 2006). Therefore, research on high-efficiency, low-cost and recyclable catalysts is of high importance.
Transesterification for biodiesel production can be carried out catalytically and non-catalytically under supercritical conditions and enzyme-catalyzed reactions (Leung et al., 2010). Catalytic reactions can also be divided into homogeneous/heterogeneous alkali-catalyzed and homogeneous/heterogeneous acid-catalyzed reactions (Avhad and Marchetti, 2015). The application of transesterification with tobacco seeds is shown in Table 2. Generally, the content of FFAs in tobacco seeds is approximately 6% (Stanisavljević et al., 2007a). This high level would result in saponification and then the difficulty in transesterification because the FFA level exceeds 2% (Anguebes-Franseschi et al., 2016). To decrease acid levels, pre-esterification with homogeneous acid could be performed. FFA content was shown to decrease to approximately 1.00% after pre-esterification. This content met the standards for the alkali-catalyzed transesterification process into biodiesel (Murmu et al., 2017). Compared with other catalysts, alkali catalysts are more commonly used with tobacco seed oil. The common homogeneous and heterogeneous alkalis reported in the literature are KOH, NaOH, CH3ONa, and KHCO3/Al2O3 (Veljković et al., 2006; Parlak et al., 2009; Anastopoulos et al., 2011; Waheed et al., 2015; Hariram and Rajan, 2016; Karabas et al., 2016, 2019; Motojesi et al., 2017; Fornasier et al., 2018; Samuel et al., 2020; Santoso et al., 2021). Due to their low costs and high catalytic capacities, NaOH and KOH are the most commonly used homogeneous alkali catalysts. Moreover, KHCO3/Al2O3, a heterogeneous alkali catalyst, can be rapidly separated from the reaction solution with centrifugation or filtration in the subsequent purification process. Moreover, this solid heterogeneous catalyst can simultaneously catalyze transesterification and esterification reactions with little impact from the high contents of FFAs. Thus, these heterogeneous catalysts are very suitable for application with feedstocks containing high FFA contents. However, this reaction often requires a relatively long time because the uniformity of the three-phase transesterification reaction is poor (Kulkarni et al., 2006; Liu et al., 2008). The non-catalytic supercritical method with methanol is also applied in tobacco seed oil conversion to biodiesel. The supercritical transesterification method often requires much higher temperature and pressure in a sealed reactor with an external heater. The transesterification reaction starts during the heating and pressurization period. Compared with the conventional alkali-catalyzed method, this process has advantages in terms of environmental friendliness and ease of purification but requires more energy consumption due to the high temperature and pressure utilized (García-Martínez et al., 2017). Recently, enzyme catalysts for biodiesel production have become increasingly attractive since they do not undergo saponification, and the subsequent purification process is simple. However, these catalysts are less often used commercially because of relatively long transesterification times and high costs (Moazeni et al., 2019).
Role of Biotechnology in Biodiesel Production in Tobacco Seed Oil
Biotechnology is an important method for improving tobacco seed oil production and accumulation. In recent years, fatty acid and TAG accumulation in tobacco seeds has become a promising way to meet the increasing demand for renewable biofuels (Andrianov et al., 2010; Li et al., 2010; Hernández et al., 2012). The first step of fatty acid synthesis involves acetyl-CoA carboxylase (ACCase) catalysis of the carboxylation of acetyl-CoA to malonyl-CoA (Alonso et al., 2010; Fan et al., 2013). Phosphoenolpyruvate carboxylases (PEPCs) play important roles in fatty acid biosynthesis in seeds of oil plants by regulating carbon partitioning (Fan et al., 2013). During seed oil production and accumulation in oil plants, the increased mRNA level of PEPC indicates a potential role in partitioning carbohydrates to fatty acid biosynthesis (Azocar et al., 2010; Fan et al., 2013). Overexpression of JcPEPC1 in tobacco results in an increase in PEPC activity and fatty acid content, proving its important role in seed oil biosynthesis (Fan et al., 2013).
During TAG accumulation, diacylglycerol acyltransferases (DGATs) are the final enzymes employed in TAG biosynthesis, and they play a critical role in plant oleaginous seed production (Xu et al., 2014). In particular, overexpression of DGATs was shown to increase the accumulation of TAG in plant seeds (Li et al., 2010; Xu et al., 2014). To date, the DGAT1, DGAT2 and DGAT3 gene families that encode the DGAT enzymes have been identified in plants (Li et al., 2010; Hernández et al., 2012; Xu et al., 2014). Xu et al. (2014) reported that overexpression of JcDGAT1 and JcDGAT2 from J. curcas resulted in increases in seed oil contents of 32.8 and 31.8%, respectively, in transgenic tobacco.
Most recently, the CRISPR-Cas9 system has emerged as a programmable and versatile tool for genome editing in a wide variety of organisms (Tian et al., 2020 and 2021). Altering the fatty acid metabolic profile by raising the oleic acid content improves the properties of the biodiesel produced and accumulated from tobacco seed oil. Tobacco fad2–2 mutant seeds based on CRISPR-Cas9 gene-editing technology showed a sharp increase in oleic acid content from 11% to over 79%, whereas the linoleic acid content dropped from 72 to 7% (Tian et al., 2020). Additionally, lipid accumulation in tobacco seeds was dramatically enhanced by approximately 18 and 15% in two targeted knockout mutant lines, of which the NtAn1 gene of the TT8 homolog was created by CRISPR-Cas9 (Tian et al., 2021). Yang et al. (2006) employed a partial coding sequence of a microsomal ω-6 fatty acid desaturase gene (FAD2) associated with the first step in polyunsaturated fatty acid biosynthesis to make an hpRNA-producing construct to more specifically silence the endogenous FAD2 gene, thereby realizing a significant increase in the oleic acid level in tobacco seed lipids. In addition, Zhang L. et al. (2016) stated that the oleic acid content relative to the content of total seed lipids of transformants was drastically decreased in FAD2-silenced tobacco at a low temperature, and there was a corresponding increase in the content of polyunsaturated fatty acid. The reduction in DGAT1 transcript levels in transgenic tobacco mediated by hpRNA is correlated with a decrease in oil content of 9–49% in mature seeds of transgenic lines (Zhang et al., 2005).
Identifying important regulatory factors involved in tobacco seed oil biosynthesis may enhance our understanding of the process of oil biosynthesis and improve strategies for increasing oil production. It has also been reported that several transcription factors, such as LEC1, LEC2, and FUS3, are associated with embryo development and seed oil biosynthesis (Chen et al., 2014; Zhang M. et al., 2016). However, many genes, proteins, regulatory factors, and multiple pathways are associated with the processes of tobacco seed oil biosynthesis and accumulation (Figure 3), and the molecular mechanisms of fatty acid and TAG biosyntheses are not fully understood (Zhang M. et al., 2016; Zhou et al., 2019).
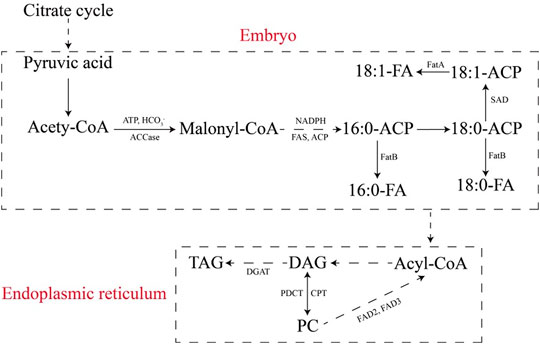
FIGURE 3. Fatty acid and TAG metabolic pathways in tobacco seeds. ACCase, acetyl-CoA carboxylase; FAS, fatty acid synthase; ACP, acyl carrier protein; FA, fatty acid; FatA/B, acyl-ACP thioesterases; DAG, diacyl-glycerol; TAG, triacylglycerol; PC, phosphatidylcholine; SAD, stearoyl-ACP desaturase; DGAT, diacylglycerol acyltransferase; PDCT, phosphatidylcholine:diacylglycerol cholinephosphotransferase; CPT, CDP-choline:1,2-diacylglycerol cholinephosphotransferase; FAD2, oleoyl desaturase; FAD3, linoleoyl desaturase (Zhang, et al., 2016b; Zhou et al., 2019).
Influencing Factors for Biodiesel Production During Tobacco Seed Oil Accumulation
During seed development, the contents of TAG, diacylglycerol, and sterol esters were shown to increase, while those of phosphatidylcholine, phosphatidylethanolamine, phosphatidylinositol, phosphatidylglycerol, monogalactosyldiacylglycerol, digalactosyldiacylglycerol, and sulfoquinovosyldiacylglycerol were shown to decrease, suggesting that the proportions of linoleate and linolenate decreased with concomitant increased proportions of stearate and oleate in the phospholipids (Koiwai et al., 1982). The contents of tobacco seed fatty acids in ovaries grown in the greenhouse were more than four-fold higher than those in mature ovaries cultured in vitro because of many cultural factors, such as mineral and sugar concentrations, plant hormone interactions, oxygen concentration, and culture temperatures (Toshiake et al., 2006). Lin et al. (2020) were able to increase the seed and oil yields and modify the fatty acid composition of tobacco plants by enhancing the level of nitrogen applied. However, the effects of many influencing factors (such as the ecological environment, heredity, exogenous hormones, etc.) and agricultural strategies (e.g., Fertilization, irrigation, weeding, diseases and pests control) on tobacco seed oil biosynthesis and accumulation associated with biodiesel production have not been revealed.
Properties and Application of Biodiesel From Tobacco Seed Oil
Tobacco seed oil cannot be used directly in diesel engines, primarily due to its high viscosity and low heat content (Sharma et al., 2008). Transesterification reaction converts triglycerides into methyl esters to meet the specifications of biodiesel standards. Many countries have implemented national standards to promote biodiesel quality and regulate biodiesel production (Yang et al., 2016). Generally, the evaluation of biodiesel properties is based on ASTM D6751-2015c and BS EN 14214-2012 + A1-2019 standards (BSI, 2019; ASTM D6751-20a, 2020). Biodiesel is characterized by considering its physicalchemical and fuel properties, such as density, kinematic viscosity, cloud and pour point, energy content, cetane index (Ashraful et al., 2014). It is worth emphasizing that carbon chain length and saturation of fatty acids are important factors affecting the properties of biodiesel (Knothe, 2005). Additionally, the fluidity of biodiesel is related to density, kinematic viscosity, and cloud and pour point. The energy content and cetane index influence the production of torque and power. The flashpoint and ignition point are important parameters for evaluating the safety of biodiesel. Biodiesel has relatively low contents of ash, sulfur, and carbon residue; thus, the amount of pollutants emitted is reduced. Biodiesel usually has limited stability and is likely to form polymers that may block the fuel supply system. The amounts of monoglycerides, diglycerides, triglycerides, and free and total glycerine are important indicators of biodiesel purity. The metal ion residues (Na+, K+, Ca2+, Mg2+) introduced by catalysts could cause deposition and wear of engine components. Water and methanol contents and acid values may also accelerate erosion of the injection system and increase carbon deposition in engines. An evaluation of the important properties of tobacco seed oil biodiesel (TSOBD) is shown in Table 3 (Veljković et al., 2006; Anastopoulos et al., 2011; Usta et al., 2011; Parlak et al., 2012; Rao B. S. et al., 2013, 2013c; Waheed et al., 2015; Hariram and Rajan, 2016; Guntur and Prasanthi, 2018; Karabas et al., 2019; He et al., 2021).
Generally, the flashpoint, density, acid value, sulfur content, and carbon residue of TSOBD are within or near the ranges recommended by European standards. Some parameters, such as oxidation stability, cetane index, iodine value, and cold filter plugging point, are correlated with the methyl ester content of TSOBD (Ramos et al., 2008). Variations in these indexes may be explained by the different characteristics of tobacco seeds and the whole biodiesel production process. Tobacco seed oil is rich in unsaturated fatty acids; thus, TSOBD has a higher iodine value and unsatisfactory oxidation stability (Usta et al., 2011), which can also be altered by the type and amount of catalyst used in the transesterification reaction (Parlak et al., 2012). The addition of antioxidants can enhance oxidation stability. TSOBD has a lower energy content and cetane index than fossil diesel, which can be improved by blending with other types of biodiesels or pure diesel (Usta, 2005; Anastopoulos et al., 2011). TSOBD addition can also improve the lubrication of engine fuel systems and extend the operating life of system components (Cesur et al., 2014).
Brake power, brake thermal efficiency (BTE), and brake special fuel consumption (BSFC) are used to evaluate the fuel characteristics of biodiesel (Ashraful et al., 2014). TSOBD blends are compatible with pure diesel uses and can increase combustion efficiency and show relatively high brake power and BTE and low BSFC in most cases. On the other hand, TSOBD blends in diesel engines can reduce CO and SO2 emissions while slightly increasing NOx emissions (Usta, 2005; He et al., 2021). The TSOBD mixing ratio (Parlak et al., 2012), injection opening pressure (Hountalas et al., 2003), injection time (Teoh et al., 2015), engine load (He et al., 2021), engine speed (Parlak et al., 2013), engine type (Rao et al., 2013c), and compression ratio (Guntur and Prasanthi, 2018) affect TSOBD blend properties and pollutant emissions. It is worth noting that a fuel blend with a low percentage of TSOBD is more efficient at a higher load than at a partial load (Usta, 2005). Compared to diesel, NOx emissions are slightly increased due to high temperatures and oxygen levels in the cylinder at relatively high load, but there is no significant difference in NOx emissions at partial load. In addition, Sharma et al. (2020) examined the impact of engine input parameters on the engine performance using the response surface methodology approach. Low heat rejection diesel engines with relatively high capacities to handle fuels with low calorific values can enhance TSOBD fuel characteristics while also producing more NOx emissions than conventional engines (Rao N. V. et al., 2013). The NOx emissions of variable compression ratio diesel engine using TSOBD decreased nearly 50% at 16:1 compression ratio compared to 18:1 compression ratio at half and full load (Guntur and Prasanthi, 2018). In addition, catalysts that convert NOx into N2, such as traditional three-way and new developed zeolite-based catalyst, can be introduced to reduce NOx emissions of diesel engine (Beale et al., 2015).
Future Directions
Although mechanical processing gives rise to relatively fast and inexpensive results, the small size of tobacco seeds leads to relatively high requirements for pressing equipment and pretreatment technology, which can subsequently raise production costs. The advantages of conventional extraction with solvents are low cost, simple equipment, no need for filtration, and high efficiency, but the disadvantages are environmental pollution and the application of high temperatures (Castro and Priego-Capote, 2010). The application of the supercritical fluid extraction process is based on the balance between economic benefits and environmental benefits. Recently, new extraction processes using renewable natural products or nonhazardous solvents have been applied to extractions of grape seed oil and sunflower oil (Sineiro et al., 1998; Passos et al., 2009). Enzyme extraction is an enzyme-assisted aqueous extraction that operates under moderate conditions and provides a high oil yield. In the future, processes for the extraction of tobacco seed oil should combine two or more extraction techniques into a complete process or modify available techniques to enhance oil yields. Moreover, more economical and environmentally friendly extraction processes should be utilized.
The ideal biodiesel transesterification catalyst should have the characteristics of high catalytic capacity, stability, minimal lixiviation, reusability, low cost, recyclability, etc. These are crucial for the selection of appropriate catalysts and reaction conditions in biodiesel production from tobacco seed oil. Heterogeneous catalysts are easy to separate from the reaction mixture, regenerate and reuse. Therefore, heterogeneous catalysts will likely replace traditional homogeneous catalysts for transesterification of tobacco seed oil in the near future. Compared with other transesterification methods, the enzymatic method has the following advantages: the catalyst (i.e., enzyme) is environmentally friendly; the operating conditions are moderate; the quality of the by-product glycerin is high; side effects such as saponification are few; the purity of the final product is high, and the enzyme method allows a relatively high FFA content and proceeds with one step. Hence, the enzymatic production of biodiesel is another important direction for the development of tobacco seed oil use. Although the catalysts for these two methods are relatively slow and expensive, novel heterogeneous and enzymatic catalysis may overcome these drawbacks.
Biotechnology, such as overexpression, silencing, knockout, and editing of genes associated with seed development, plays vital roles in the accumulation and production of tobacco seed oil in the future. Using the mining of genes and proteins involved in tobacco seed oil biosynthesis, the content of tobacco seed oil will be increased through metabolic pathways. Reorganization of acyl flux and coexpression of genes involved in lipid metabolism and TAG production accumulated more than 15% oil (dry weight) in tobacco leaves (Vanhercke et al., 2014). Tobacco seed oil contents differ among different tobacco varieties, so the yield of biodiesel from tobacco seeds can be increased by cultivating high-oil varieties. Additionally, grafting may also be an important way to increase biodiesel yields from tobacco seeds during planting. Strikingly, national policies and government support will also affect the development and research of tobacco seed oil and leaves for the production of biodiesel.
Recently, many countries have implemented higher standards and requirements for biodiesel fuel characteristics and pollution emissions. Further research should focus on modifications of TSOBD properties to meet diesel standards and assess the efficiency of TSOBD in an experimental engine setup, either alone or blended with diesel. The evaluation of biodiesel should involve all of the parameters specified in standards and provide for a good balance between engine performance and NOx emissions by optimizing engine operating parameters or designing new diesel engines.
Conclusions
Tobacco seed is identified as a promising feedstock for sustainable biodiesel production due to its suitable physicochemical composition, higher oil yields, and lower competition with food crops. However, securing sustainable and sufficient amounts of tobacco seed oil for large scale biodiesel production is not achieved yet. The tobacco seed for sustainable biodiesel production is significantly affected by various factors, which could be considered as varieties, cultivation, extraction, transesterification, application etc. Thus, future studies should focus on the higher-oil varieties with biotechnology, high-effective cultivation technique, economical and environmentally friendly extraction methods, novel heterogeneous or enzymatic catalyzed transesterifications and modifications of TSOBD physicochemical properties.
Author Contributions
Writing—original draft preparation, SW; Revising—manuscript, CG; Data curation, HP; Figure drawing, KW; Writing—reviewing, DL; Editing, supervision, KC; Writing—reviewing, supervision, HZ. All authors have read and agreed to the published version of the manuscript.
Funding
This work was financially supported by the Science and Technology Program of Science and Technology Department of Guizhou Province (No. 20191212 and 20162536). The judging criteria of maturity of tobacco leaves and application in different flavor areas in Bijie and Guizhou Kehe Platform Talents (2020)4004, GZU (Guizhou University) Found for Cultivation ((2020)73). The authors declare that this study received funding from Guizhou Provincial Tobacco Company (No. 201717 and 2021520000240025). The funder was not involved in the study design, collection, analysis, interpretation of data, the writing of this article or the decision to submit it for publication.
Conflict of Interest
The authors declare that the research was conducted in the absence of any commercial or financial relationships that could be construed as a potential conflict of interest.
Publisher’s Note
All claims expressed in this article are solely those of the authors and do not necessarily represent those of their affiliated organizations, or those of the publisher, the editors, and the reviewers. Any product that may be evaluated in this article, or claim that may be made by its manufacturer, is not guaranteed or endorsed by the publisher.
References
Adepoju, T. F. (2020). Optimization Processes of Biodiesel Production from Pig and Neem (Azadirachta indica a.Juss) Seeds Blend Oil Using Alternative Catalysts from Waste Biomass. Ind. Crops Prod. 149, 112334. doi:10.1016/j.indcrop.2020.112334
Andrianov, V., Borisjuk, N., Pogrebnyak, N., Brinker, A., Dixon, J., Spitsin, S., et al. (2010). Tobacco as a Production Platform for Biofuel: Overexpression ofArabidopsis DGATandLEC2genes Increases Accumulation and Shifts the Composition of Lipids in green Biomass. Plant Biotechnol. J. 8, 277–287. doi:10.1111/j.1467-7652.2009.00458.x
Anguebes-Franseschi, F., Córdova-Quiroz, A., Cerón-Bretón, J., Aguilar-Ucan, C., Castillo-Martínez, G., Cerón-Bretón, R., et al. (2016). Optimization of Biodiesel Production from African Crude Palm Oil (Elaeis Guineensis Jacq) with High Concentration of Free Fatty Acids by a Two-step Transesterification Process. Oje 06 (1), 13–21. doi:10.4236/oje.2016.61002
Arumugam, A., Thulasidharan, D., and Jegadeesan, G. B. (2018). Process Optimization of Biodiesel Production from Hevea Brasiliensis Oil Using Lipase Immobilized on Spherical Silica Aerogel. Renew. Energ. 116, 755–761. doi:10.1016/j.renene.2017.10.021
Ashraf-Khorassani, M., Coleman, W. M., Dube, M. F., Isaac, G., and Taylor, L. T. (2015). Synthesis, Purification, and Quantification of Fatty Acid Ethyl Esters after Trans-esterification of Large Batches of Tobacco Seed Oil. Btrge. Zur Tabakforschung 26 (5), 205–213. doi:10.1515/cttr-2015-0008
Ashraful, A. M., Masjuki, H. H., Kalam, M. A., Rizwanul Fattah, I. M., Imtenan, S., Shahir, S. A., et al. (2014). Production and Comparison of Fuel Properties, Engine Performance, and Emission Characteristics of Biodiesel from Various Non-edible Vegetable Oils: A Review. Energ. Convers. Manage. 80, 202–228. doi:10.1016/j.enconman.2014.01.037
ASTM D6751-20a (2020). License Agreement, Standard Specification for Biodiesel Fuel Blend Stock (B100) for Middle Distillate Fuels. ICS Code 75, 11. doi:10.1520/D6751-20A
Avhad, M. R., and Marchetti, J. M. (2015). A Review on Recent Advancement in Catalytic Materials for Biodiesel Production. Renew. Sustain. Energ. Rev. 50, 696–718. doi:10.1016/j.rser.2015.05.038
Awais, M., Musmar, S. e. A., Kabir, F., Batool, I., Rasheed, M. A., Jamil, F., et al. (2020). Biodiesel Production from Melia Azedarach and Ricinus Communis Oil by Transesterification Process. Catalysts 10 (4), 427. doi:10.3390/catal10040427
Azócar, L., Ciudad, G., Heipieper, H. J., and Navia, R. (2010). Biotechnological Processes for Biodiesel Production Using Alternative Oils. Appl. Microbiol. Biotechnol. 88 (3), 621–636. doi:10.1007/s00253-010-2804-z
Banković-Ilić, I. B., Stamenković, O. S., and Veljković, V. B. (2012). Biodiesel Production from Non-edible Plant Oils. Renew. Sustain. Energ. Rev. 16 (6), 3621–3647. doi:10.1016/j.rser.2012.03.002
Beale, A. M., Gao, F., Lezcano-Gonzalez, I., Peden, C. H. F., and Szanyi, J. (2015). Recent Advances in Automotive Catalysis for NOx Emission Control by Small-Pore Microporous Materials. Chem. Soc. Rev. 44 (20), 7371–7405. doi:10.1039/c5cs00108k
Berbeć, A. K., and Matyka, M. (2020). Biomass Characteristics and Energy Yields of Tobacco (Nicotiana Tabacum L.) Cultivated in Eastern Poland. Agriculture 10 (11), 551. doi:10.3390/agriculture10110551
BSI (2019). BS EN 14214:2012+A1:2019 Liquid Petroleum Products-Fatty Acid Methyl Esters (FAME) for Use in Diesel Engines and Heating Applications-Requirements and Test Methods. London, United Kingdom: BSI. ICS Code: 75.160.40.
Cesur, İ., Ayhan, V., Parlak, A., Savaş, Ö., and Aydin, Z. (2014). The Effects of Different Fuels on Wear between Piston Ring and Cylinder. Adv. Mech. Eng. 6, 503212–503218. doi:10.1155/2014/503212
Chen, M., Xuan, L., Wang, Z., Zhou, L., Li, Z., Du, X., et al. (2014). TRANSPARENT TESTA8 Inhibits Seed Fatty Acid Accumulation by Targeting Several Seed Development Regulators in Arabidopsis. Plant Physiol. 165 (2), 905–916. doi:10.1104/pp.114.235507
Chung, C.-c., Zhang, Y., Liu, L., Wang, Y., and Wei, Z. (2020). The Evolution of Biodiesel Policies in China over the Period 2000-2019. Processes 8 (8), 948. doi:10.3390/pr8080948
Dean, S. W., Anastopoulos, G., Deligiannis, A., Kalligeros, S., Karonis, D., Zannikos, F., et al. (2010). Synthesis of Biodiesel from Tobacco and Waste Frying Oil Using Heterogeneous KHCO3/Al2O3 Catalyst. J. ASTM Int. 7 (3), 293–311. doi:10.1520/JAI102574
Demirbas, A. (2007). Importance of Biodiesel as Transportation Fuel. Energy Policy 35 (9), 4661–4670. doi:10.1016/j.enpol.2007.04.003
Fan, Z., Li, J., Lu, M., Li, X., and Yin, H. (2013). Overexpression of Phosphoenolpyruvate Carboxylase from Jatropha Curcas Increases Fatty Acid Accumulation in Nicotiana Tabacum. Acta Physiol. Plant 35, 2269–2279. doi:10.1007/s11738-013-1264-3
Faugno, S., del Piano, L., Crimaldi, M., Ricciardiello, G., and Sannino, M. (2016). Mechanical Oil Extraction of Nicotiana Tabacum L. Seeds: Analysis of Main Extraction Parameters on Oil Yield. J. Agricult Engineer 47 (3), 142–147. doi:10.4081/jae.2016.539
Fornasier, F., Gomez, J. F. C., Sansone, F. D. C., Schneider, R. D. C. D. S., Costa, A. B. d., Moraes, J. A. R., et al. (2018). Biodiesel Production from Energy Tobacco. Orbital: Electron. J. Chem. 10 (2), 123–132. doi:10.17807/orbital.v10i2.1120
García-Martínez, N., Andreo-Martínez, P., Quesada-Medina, J., de los Ríos, A. P., Chica, A., Beneito-Ruiz, R., et al. (2017). Optimization of Non-catalytic Transesterification of Tobacco ( Nicotiana Tabacum ) Seed Oil Using Supercritical Methanol to Biodiesel Production. Energ. Convers. Manage. 131, 99–108. doi:10.1016/j.enconman.2016.10.078
Geow, C. H., Tan, M. C., Yeap, S. P., and Chin, N. L. (2021). A Review on Extraction Techniques and its Future Applications in Industry. Eur. J. Lipid Sci. Technol. 123 (4), 2000302. doi:10.1002/ejlt.202000302
Giannelos, P. N., Zannikos, F., Stournas, S., Lois, E., and Anastopoulos, G. (2002). Tobacco Seed Oil as an Alternative Diesel Fuel: Physical and Chemical Properties. Ind. Crops Prod. 16 (1), 1–9. doi:10.1016/S0926-6690(02)00002-X
Gowtham Rajan, A., Sivasubramanian, M., Gowthaman, S., and Ramkumar, P. (2021). Investigation of Physical and Chemical Properties of Tobacco Seed Oil Fatty Acid Methyl Ester for Biodiesel Production. Mater. Today Proc. 46, 7670–7675. doi:10.1016/j.matpr.2021.02.081
Guntur, R., and Prasanthi, G. (2018). Effect of Compression Ratio on Thermal Characteristics of VCR Diesel Engine Using Nicotiana Tabacum L. Seed Oil Methyl Ester. J. Eng. Sci. Technol. 13 (3), 558–572.
Hariram, V., and Rajan, A. G. (2016). Extraction of Tobacco (Nicotiana Tabacum L.) Seed Oil and Biodiesel Preparation through Two Stage Transesterification. Res. J. Pharm. Biol. Chem. Sci. 7 (2), 978–983.
He, A., Hu, L., Zhang, Y., Jiang, Y., Wang, X., Xu, J., et al. (2021). High-Efficiency Catalytic Transfer Hydrogenation of Biomass-Based 5-Hydroxymethylfurfural to 2,5-Bis(hydroxymethyl)furan over a Zirconium-Carbon Coordination Catalyst. ACS Sustain. Chem. Eng. 9 (46), 15557–15570. doi:10.1021/acssuschemeng.1c05618
Hernández, M. L., Whitehead, L., He, Z., Gazda, V., Gilday, A., Kozhevnikova, E., et al. (2012). A Cytosolic Acyltransferase Contributes to Triacylglycerol Synthesis in Sucrose-Rescued Arabidopsis Seed Oil Catabolism Mutants. Plant Physiol. 160 (1), 215–225. doi:10.1104/pp.112.201541
Hill, J., Nelson, E., Tilman, D., Polasky, S., and Tiffany, D. (2006). Environmental, Economic, and Energetic Costs and Benefits of Biodiesel and Ethanol Biofuels. Proc. Natl. Acad. Sci. 103 (30), 11206–11210. doi:10.1073/pnas.0604600103
Hountalas, D. T., Kouremenos, D. A., Binder, K. B., Schwarz, V., and Mavropoulos, G. C. “Effect of Injection Pressure on the Performance and Exhaust Emissions of a Heavy Duty DI Diesel Engine,” in Proceedings of the SAE 2003 World Congress & Exhibition, Detroit, Michigan, U.S.A, March 2003. doi:10.4271/2003-01-0340
Hu, L., Wu, Z., Jiang, Y., Wang, X., He, A., Song, J., et al. (2020). Recent Advances in Catalytic and Autocatalytic Production of Biomass-Derived 5-Hydroxymethylfurfural. Renew. Sustain. Energ. Rev. 134, 110317. doi:10.1016/j.rser.2020.110317
Jain, S. (2019). “The Production of Biodiesel Using Karanja (Pongamia Pinnata) and Jatropha (Jatropha Curcas) Oil,” in Biomass, Biopolymer-Based Materials, and Bioenerg. Editors D. Verma, E. Fortunati, S. Jain, and X. Zhang (Sawston, United Kingdom: Woodhead Publishing), 397–408. doi:10.1016/B978-0-08-102426-3.00017-5
Karabas, H., Boran, S., and Yazgan, H. R. (2016). Identification of Process Parameters of Tobacco Seed Oil Methyl Ester by Applied Multi Response Taguchi Technique. Fresen. Environ. Bull. 25, 3802–3807.
Karabas, H., Boran, S., and Yazgan, H. R. (2019). Optimization of Production Parameters of Tobacco Seed Oil Methyl Ester Using Multi-Response Taguchi Method and MANOVA. J. Chem. Soc. Pakistan 41 (3), 414.
Khan, T. M. Y., Atabani, A. E., Badruddin, I. A., Badarudin, A., Khayoon, M. S., and Triwahyono, S. (2014). Recent Scenario and Technologies to Utilize Non-edible Oils for Biodiesel Production. Renew. Sustain. Energ. Rev. 37 (3), 840–851. doi:10.1016/j.rser.2014.05.064
Kim, J.-H., Jung, J.-M., Cho, S.-H., Tsang, Y. F., Wang, C.-H., Lee, J., et al. (2019). Upgrading Bio-Heavy Oil via Esterification of Fatty Acids and Glycerol. J. Clean. Prod. 217, 633–638. doi:10.1016/j.jclepro.2019.01.289
Knothe, G. (2005). Dependence of Biodiesel Fuel Properties on the Structure of Fatty Acid Alkyl Esters. Fuel Process. Technol. 86 (10), 1059–1070. doi:10.1016/j.fuproc.2004.11.002
Koiwai, A., Suzuki, F., Matsuzaki, T., and Kawashima, N. (1982). Changes in Glycerolipids and Their Fatty Acid Composition during Maturation of Tobacco Seeds. Phytochemistry 21 (2), 305–308. doi:10.1016/S0031-9422(00)95256-7
Koiwai, A., Suzuki, F., Matsuzaki, T., and Kawashima, N. (1983). The Fatty Acid Composition of Seeds and Leaves of Nicotiana Species. Phytochemistry 22 (6), 1409–1412. doi:10.1016/S0031-9422(00)84024-8
Kulkarni, M. G., Gopinath, R., Meher, L. C., and Dalai, A. K. (2006). Solid Acid Catalyzed Biodiesel Production by Simultaneous Esterification and Transesterification. Green. Chem. 8 (12), 1056–1062. doi:10.1039/b605713f
Leung, D. Y. C., Wu, X., and Leung, M. K. H. (2010). A Review on Biodiesel Production Using Catalyzed Transesterification. Appl. Energ. 87 (4), 1083–1095. doi:10.1016/j.apenergy.2009.10.006
Li, R., Yu, K., Hatanaka, T., and Hildebrand, D. F. (2010). VernoniaDGATs Increase Accumulation of Epoxy Fatty Acids in Oil. Plant Biotechnol. J. 8 (2), 184–195. doi:10.1111/j.1467-7652.2009.00476.x
Lin, Y., Kong, D., Wang, Z., Chen, Y., Yang, Z., Wu, C., et al. (2020). Nitrogen Application Modifies the Seed and Oil Yields and Fatty Acid Composition of Nicotiana Tabacum. horts 55 (12), 1898–1902. doi:10.21273/HORTSCI15335-20
Liu, X., He, H., Wang, Y., Zhu, S., and Piao, X. (2008). Transesterification of Soybean Oil to Biodiesel Using Cao as a Solid Base Catalyst. Fuel 87 (2), 216–221. doi:10.1016/j.fuel.2007.04.013
Liu, X., Pan, H., Zhang, H., Li, H., and Yang, S. (2019). Efficient Catalytic Upgradation of Bio-Based Furfuryl Alcohol to Ethyl Levulinate Using Mesoporous Acidic MIL-101(Cr). ACS Omega 4, 8390–8399. doi:10.1021/acsomega.9b00480
Luque de Castro, M. D., and Priego-Capote, F. (2010). Soxhlet Extraction: Past and Present Panacea. J. Chromatogr. A 1217 (16), 2383–2389. doi:10.1016/j.chroma.2009.11.027
Ma, F., and Hanna, M. A. (1999). Biodiesel Production: a review1Journal Series #12109, Agricultural Research Division, Institute of Agriculture and Natural Resources, University of Nebraska-Lincoln.1. Bioresour. Technol. 70 (1), 1–15. doi:10.1016/S0960-8524(99)00025-5
Maestri, D. M., and Guzmán, C. A. (1995). A Comparative Study of Seed Lipid Components of Nicotianeae (Solanaceae). Biochem. Syst. Ecol. 23 (2), 201–207. doi:10.1016/0305-1978(95)93850-3
Majdi, S., Barzegar, M., Jabbari, A., and Alikhani, M. A. (2012). Supercritical Fluid Extraction of Tobacco Seed Oil and its Comparison with Solvent Extraction Methods. J. Agr. Sci. Tech. 14 (5), 1043–1051. doi:10.1111/j.1744-697X.2012.00258.x
Matsuzaki, T., Iwai, S., and Koiwai, A. (1988). Effects of Temperature on Seed Fatty Acid Composition in Ovary Culture of Tobacco. Agric. Biol. Chem. 52 (5), 1283–1285. doi:10.1080/00021369.1988.10868821
Meher, L., Vidyasagar, D., and Naik, S. (2006). Technical Aspects of Biodiesel Production by Transesterification-A Review. Renew. Sustain. Energ. Rev. 10 (3), 248–268. doi:10.1016/j.rser.2004.09.002
Mishra, V. K., and Goswami, R. (2018). A Review of Production, Properties and Advantages of Biodiesel. Biofuels 9 (2), 273–289. doi:10.1080/17597269.2017.1336350
Mizik, T., and Gyarmati, G. (2021). Economic and Sustainability of Biodiesel Production-A Systematic Literature Review. Clean. Technol. 3 (1), 19–36. doi:10.3390/cleantechnol3010002
Moazeni, F., Chen, Y.-C., and Zhang, G. (2019). Enzymatic Transesterification for Biodiesel Production from Used Cooking Oil, A Review. J. Clean. Prod. 216, 117–128. doi:10.1016/j.jclepro.2019.01.181
Motojesi, O., Ogunniyi, D. S., and Odetoye, T. E. (2017). Transesterification of Tobacco Seed Oil Using Some Agricultural Wastes as Catalysts. Nigeria: Unilorin Institutional Repository.
Mukhtar, A., Ullah, H., and Mukhtar, H. (2007). Fatty Acid Composition of Tobacco Seed Oil and Synthesis of Alkyd Resin. Chin. J. Chem. 25 (5), 705–708. doi:10.1002/cjoc.200790132
Murmu, R., Sutar, H., and Patra, S. (2017). Experimental Investigation and Process Optimization of Biodiesel Production from Kusum Oil Using Taguchi Method. Aces 07 (4), 464–476. doi:10.4236/aces.2017.74033
Muthukumaran, C., Praniesh, R., Navamani, P., Swathi, R., Sharmila, G., and Manoj Kumar, N. (2017). Process Optimization and Kinetic Modeling of Biodiesel Production Using Non-edible Madhuca Indica Oil. Fuel 195, 217–225. doi:10.1016/j.fue1.2017.01.06010.1016/j.fuel.2017.01.060
Pan, H., Liu, Y., Xia, Q., Zhang, H., Guo, L., Li, H., et al. (2020). Synergetic Combination of a Mesoporous Polymeric Acid and a Base Enables Highly Efficient Heterogeneous Catalytic One-Pot Conversion of Crude Jatropha Oil into Biodiesel. Green. Chem. 22 (5), 1698–1709. doi:10.1039/C9GC04135D
Pao, H.-T., and Tsai, C.-M. (2010). CO2 Emissions, Energy Consumption and Economic Growth in BRIC Countries. Energy Policy 38 (12), 7850–7860. doi:10.1016/j.enpol.2010.08.045
Parlak, A., Ayhan, V., Cesur, İ., and Kökkülünk, G. (2013). Investigation of the Effects of Steam Injection on Performance and Emissions of a Diesel Engine Fuelled with Tobacco Seed Oil Methyl Ester. Fuel Process. Technol. 116, 101–109. doi:10.1016/j.fuproc.2013.05.006
Parlak, A., Karabas, H., Ayhan, V., Yasar, H., Soyhan, H. S., and Ozsert, I. (2009). Comparison of the Variables Affecting the Yield of Tobacco Seed Oil Methyl Ester for KOH and NaOH Catalysts. Energy Fuels 23 (2), 1818–1824. doi:10.1021/ef800371g
Parlak, A., Karabaş, H., Özsert, I., Ayhan, V., and Cesur, I. (2012). Application of Taguchi's Methods to Investigate Factors Affecting Emissions of a Diesel Engine Running with Tobacco Oil Seed Methyl Ester. Ijvd 59 (2/3), 196–211. doi:10.1504/IJVD.2012.048693
Passos, C. P., Yilmaz, S., Silva, C. M., and Coimbra, M. A. (2009). Enhancement of Grape Seed Oil Extraction Using a Cell Wall Degrading Enzyme Cocktail. Food Chem. 115 (1), 48–53. doi:10.1016/j.foodchem.2008.11.064
Paula Alonso, A., Dale, V. L., and Shachar-Hill, Y. (2010). Understanding Fatty Acid Synthesis in Developing Maize Embryos Using Metabolic Flux Analysis. Metab. Eng. 12 (5), 488–497. doi:10.1016/j.ymben.2010.04.002
Ramos, M. J., Fernández, C. M., Casas, A., Rodríguez, L., and Pérez, Á. (2009). Influence of Fatty Acid Composition of Raw Materials on Biodiesel Properties. Bioresour. Technol. 100 (1), 261–268. doi:10.1016/j.biortech.2008.06.039
Rao, B. S., Murthy, P. V. K., Ramjee, E., and Krishna, M. V. S. (2013a). Studies on Exhaust Emissions and Combustion Characteristics of Tobacco Seed Oil in Crude Form and Biodiesel from a High Grade Low Heat Rejection Diesel Engine. J. Ind. Eng. Res. 3 (1), 27–36.
Rao, N. V., Krishna, M. V. S. M., and Murthy, P. V. K. (2013b). Effect of Injector Opening Pressure and Injection Timing on Performance Parameters of High-Grade Low Heat Rejection Diesel Engine with Tobacco Seed Oil Based Biodiesel. Int. J. Recent Technol. Eng. 2 (4), 70–78.
Rao, N. V., Krishna, M. V. S. M., and Murthy, P. V. K. (2013c). Investigations on Performance Parameters of Ceramic Coated Diesel Engine with Tobacco Seed Oil Biodiesel. Int. J. Adv. Eng. Technol. 6 (5), 2286–2300.
Samuel, O. D., Boye, T. E., and Enweremadu, C. C. (2020). Financial and Parametric Study of Biodiesel Production from Hemp and Tobacco Seed Oils in Modified Fruit Blender and Prediction Models of Their Fuel Properties with Diesel Fuel. Bioresour. Technol. Rep. 12, 100599. doi:10.1016/j.biteb.2020.100599
Sannino, M., del Piano, L., Abet, M., Baiano, S., Crimaldi, M., Modestia, F., et al. (2017). Effect of Mechanical Extraction Parameters on the Yield and Quality of Tobacco (Nicotiana Tabacum L.) Seed Oil. J. Food Sci. Technol. 54, 4009–4015. doi:10.1007/s13197-017-2865-4
Santoso, A., Putri, D. E. K., Rusdi, M., Sumari, S., Wijaya, A. R., and Rachman, I. B. (2021). The Effect of Basic Catalyst Concentration on Tobacco Oil Transesterification (Voor-Oogst) Using Ultra-sonic Wave and its Potential as Renewable Energy. AIP Conf. Proc. 2330 (1), 070008. doi:10.1063/5.0043406
Sharma, A., Singh, Y., Kumar Singh, N., Singla, A., Chyuan Ong, H., and Chen, W.-H. (2020). Effective Utilization of Tobacco (Nicotiana Tabaccum) for Biodiesel Production and its Application on Diesel Engine Using Response Surface Methodology Approach. Fuel 273, 117793. doi:10.1016/j.fuel.2020.117793
Sharma, Y. C., Singh, B., and Upadhyay, S. N. (2008). Advancements in Development and Characterization of Biodiesel: A Review. Fuel 87 (12), 2355–2373. doi:10.1016/j.fuel.2008.01.014
Sineiro, J., Domı́nguez, H., Núñez, M. J., and Lema, J. M. (1998). Optimization of the Enzymatic Treatment during Aqueous Oil Extraction from sunflower Seeds. Food Chem. 61 (4), 467–474. doi:10.1016/S0308-8146(97)00080-0
Smil, V. (2017). Energy Transitions: Global and National Perspectives. 2nd ed. Santa Barbara, CA, USA: ABC-CLIO.
Srivastava, A., and Prasad, R. (2000). Triglycerides-based Diesel Fuels. Renew. Sust. Energ. Rev. 4 (1), 111–133. doi:10.1016/S1364-0321(99)00013-1
Stanisavljevic, I., Lakicevic, S., Velickovic, D., Lazic, M., and Veljkovic, V. (2007a). The Extraction of Oil from Tobacco (Nicotiana Tabacum L.) Seeds. CI&CEQ 13 (1), 41–50. doi:10.2298/CICEQ0701041S
Stanisavljević, I. T., Lazić, M. L., and Veljković, V. B. (2007b). Ultrasonic Extraction of Oil from Tobacco (Nicotiana Tabacum L.) Seeds. Ultrason. Sonochem. 14 (5), 646–652. doi:10.1016/j.ultsonch.2006.10.003
Stanisavljević, I. T., Veličković, D. T., Todorović, Z. B., Lazić, M. L., and Veljković, V. B. (2009). Comparison of Techniques for the Extraction of Tobacco Seed Oil. Eur. J. Lipid Sci. Technol. 111 (5), 513–518. doi:10.1002/ejlt.200800232
Stansell, G. R., Gray, V. M., and Sym, S. D. (2012). Microalgal Fatty Acid Composition: Implications for Biodiesel Quality. J. Appl. Phycol. 24, 791–801. doi:10.1007/s10811-011-9696-x
Teoh, Y. H., Masjuki, H. H., Kalam, M. A., and How, H. G. (2015). Effect of Injection Timing and EGR on Engine-Out-Responses of a Common-Rail Diesel Engine Fueled with Neat Biodiesel. RSC Adv. 5 (116), 96080–96096. doi:10.1039/C5RA14831F
Tian, Y., Chen, K., Li, X., Zheng, Y., and Chen, F. (2020). Design of High-Oleic Tobacco (Nicotiana Tabacum L.) Seed Oil by CRISPR-Cas9-Mediated Knockout of NtFAD2-2. BMC Plant Biol. 20, 233. doi:10.1186/s12870-020-02441-0
Tian, Y., Liu, X., Fan, C., Li, T., Qin, H., Li, X., et al. (2021). Enhancement of Tobacco (Nicotiana Tabacum L.) Seed Lipid Content for Biodiesel Production by CRISPR-Cas9-Mediated Knockout of NtAn1. Front. Plant Sci. 11, 599474. doi:10.3389/fpls.2020.599474
Usta, N. (2005). An Experimental Study on Performance and Exhaust Emissions of a Diesel Engine Fuelled with Tobacco Seed Oil Methyl Ester. Energ. Convers. Manage. 46 (15-16), 2373–2386. doi:10.1016/j.enconman.2004.12.002
Usta, N., Aydoğan, B., Çon, A. H., Uğuzdoğan, E., and Özkal, S. G. (2011). Properties and Quality Verification of Biodiesel Produced from Tobacco Seed Oil. Energ. Convers. Manage. 52 (5), 2031–2039. doi:10.1016/j.enconman.2010.12.021
Vanhercke, T., El Tahchy, A., Liu, Q., Zhou, X. R., Shrestha, P., Divi, U. K., et al. (2014). Metabolic Engineering of Biomass for High Energy Density: Oilseed‐like Triacylglycerol Yields from Plant Leaves. Plant Biotechnol. J. 12 (2), 231–239. doi:10.1111/pbi.12131
Vauhkonen, V., Niemi, S., Hiltunen, E., Salminen, H. J., and Pasila, A. (2009). The First Generation Biodiesel: The Effects of Raw Material on Physical Properties, Oxidation Stability and Emissions. Int. Conf. Clean Electr. Power 117–123. doi:10.1109/ICCEP.2009.5212074
Veljkovic, V., Lakicevic, S., Stamenkovic, O., Todorovic, Z., and Lazic, M. (2006). Biodiesel Production from Tobacco (Nicotiana Tabacum L.) Seed Oil with A High Content of Free Fatty Acids. Fuel 85 (17-18), 2671–2675. doi:10.1016/j.fuel.2006.04.015
Waheed, M. A., Samuel, O. D., Bolaji, B. O., and Dairo, O. U. (2015). RSM Based Optimization of Biodiesel Production from Tobacco Seed Oil. Renewable Energy and Sustainable Development. New York: Nova Science Publishers, 85–101.
Wang, A., Li, H., Zhang, H., Pan, H., and Yang, S. (2019). Efficient Catalytic Production of Biodiesel with Acid-Base Bifunctional Rod-like Ca-B Oxides by the Sol-Gel Approach. Materials 12, 83. doi:10.3390/ma12010083
Wu, S., Guo, Y., Adil, M. F., Sehar, S., Cai, B., Xiang, Z., et al. (2020). Comparative Proteomic Analysis by Itraq Reveals that Plastid Pigment Metabolism Contributes to Leaf Color Changes in Tobacco (Nicotiana Tabacum) during Curing. Ijms 21 (7), 2394. doi:10.3390/ijms21072394
Xu, R., Yang, T., Wang, R., and Liu, A. (2014). Characterisation of DGAT1 and DGAT2 from Jatropha Curcas and Their Functions in Storage Lipid Biosynthesis. Funct. Plant Biol. 41 (3), 321–329. doi:10.1071/FP12388
Yang, C., Zhang, B., Zhang, B., Wu, J., Ding, Y., and Wu, Y. (2016). Standards and Protocols for Characterization of Algae-Based Biofuels. Tr Ren. Energ. 2 (2), 56–60. doi:10.17737/tre.2016.2.2.0022
Yang, M., Zheng, G., Zhang, F., and Xu, Y. (2006). FAD2-silencing Has Pleiotropic Effect on Polar Lipids of Leaves and Varied Effect in Different Organs of Transgenic Tobacco. Plant Sci. 170 (1), 170–177. doi:10.1016/j.plantsci.2005.08.022
Zdremnan, M., and Zdremnan, D. (2006). The Tobacco Oil and its Qualities. Horticulture 63, 388–392. doi:10.15835/buasvmcn-hort:1898
Zhang, F.-Y., Yang, M.-F., and Xu, Y.-N. (2005). Silencing of DGAT1 in Tobacco Causes a Reduction in Seed Oil Content. Plant Sci. 169 (4), 689–694. doi:10.1016/j.plantsci.2005.05.019
Zhang, H., Pan, H., and Yang, S. (2017). Upgrading of Cellulose to Biofuels and Chemicals with Acidic Nanocatalysts. Cnano 13 (5), 513–527. doi:10.2174/1573413713666170405161546
Zhang, L., Lu, H., Liu, C., Xue, F., Yang, J., Ma, L., et al. (2016a). Lipid Desaturation in Prokaryotic Pathway Abates the High-Oleic Phenotype of FAD2-Silenced Tobacco at Lower Temperature. J. Plant Biochem. Biotechnol. 25, 375–381. doi:10.1007/s13562-016-0349-7
Zhang, M., Cao, X., Jia, Q., and Ohlrogge, J. (2016b). FUSCA3activates Triacylglycerol Accumulation in Arabidopsis Seedlings and Tobacco BY2 Cells. Plant J. 88 (1), 95–107. doi:10.1111/tpj.13233
Zhou, X.-R., Bhandari, S., Johnson, B. S., Kotapati, H. K., Allen, D. K., Vanhercke, T., et al. (2019). Reorganization of Acyl Flux through the Lipid Metabolic Network in Oil-Accumulating Tobacco Leaves. Plant Physiol. 182 (2), 739–755. doi:10.1104/pp.19.00667
Keywords: biodiesel, tobacco seed, inedible oilseed crop, biodiesel property, biotechnology
Citation: Wu S, Gao C, Pan H, Wei K, Li D, Cai K and Zhang H (2022) Advancements in Tobacco (Nicotiana tabacum L.) Seed Oils for Biodiesel Production. Front. Chem. 9:834936. doi: 10.3389/fchem.2021.834936
Received: 14 December 2021; Accepted: 27 December 2021;
Published: 18 January 2022.
Edited by:
Yaqiong Su, Xi’an Jiaotong University, ChinaCopyright © 2022 Wu, Gao, Pan, Wei, Li, Cai and Zhang. This is an open-access article distributed under the terms of the Creative Commons Attribution License (CC BY). The use, distribution or reproduction in other forums is permitted, provided the original author(s) and the copyright owner(s) are credited and that the original publication in this journal is cited, in accordance with accepted academic practice. No use, distribution or reproduction is permitted which does not comply with these terms.
*Correspondence: Kai Cai, Y2Fpa2FpMTk4NjExMDRAZ21haWwuY29t; Heng Zhang, aHpoYW5nMjNAZ3p1LmVkdS5jbg==
†These authors have contributed equally to this work