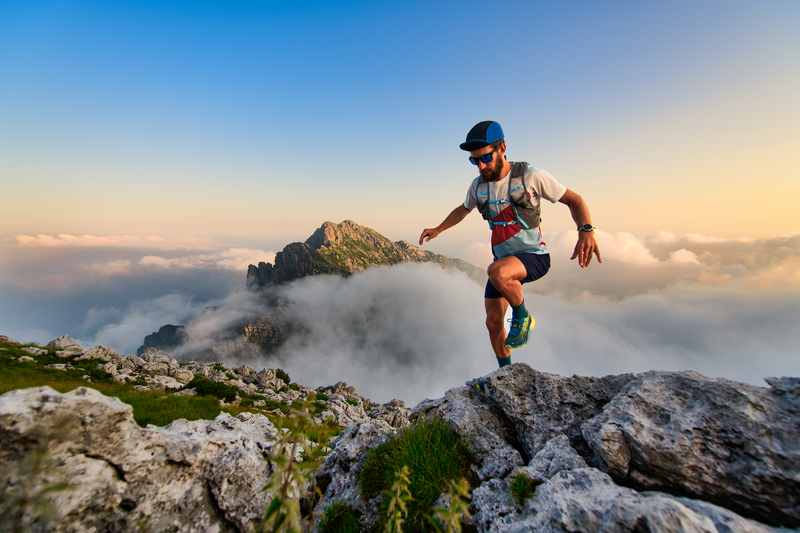
95% of researchers rate our articles as excellent or good
Learn more about the work of our research integrity team to safeguard the quality of each article we publish.
Find out more
REVIEW article
Front. Chem. , 02 February 2022
Sec. Supramolecular Chemistry
Volume 9 - 2021 | https://doi.org/10.3389/fchem.2021.832735
This article is part of the Research Topic Advances in Mechanically Bonded Molecules View all 9 articles
Molecular shuttles are typical molecular machines that could be applied in various fields. The motion modes of wheel components in rotaxanes could be strategically modulated by external stimuli, such as pH, ions, solvent, light, and so on. Light is particularly attractive because it is harmless and can be operated in a remote mode and usually no byproducts are formed. Over the past decade, many examples of light-driven molecular shuttles are emerging. Accordingly, this review summarizes the recent research progress of light-driven molecular shuttles. First, the light-driven mechanisms of molecular motions with different functional groups are discussed in detail, which show how to drive photoresponsive or non-photoresponsive molecular shuttles. Subsequently, the practical applications of molecular shuttles in different fields, such as optical information storage, catalysis for organic reactions, drug delivery, and so on, are demonstrated. Finally, the future development of light-driven molecular shuttle is briefly prospected.
Recently, mechanically interlocked molecules (MIMs) have received widespread attention for their well-defined and unique interlocked structures and appealing stimuli-responsive properties in various fields particularly in artificial molecular machines and nanoscience (Erbas-Cakmak et al., 2015; Stoddart 2017; Sun et al., 2019; Dattler et al., 2020; Cai et al., 2021). Rotaxane is one type of the most crucial representative of MIMs, which is often composed of an axle component interlocked by a macrocyclic wheel component (Ma and Tian, 2010; Guo et al., 2020). Conventionally, the axle component contains two bulky end groups as stoppers to protect the wheel component from dethreading. In addition, when the main axle has two or more different recognition stations, the macrocyclic wheel component can move reversibly along the axle chain between different binding sites under external stimuli, and thus a molecular shuttle is formed. For instance, for a two-station molecular shuttle, when two binding sites are the same, the wheel component will move along the axle between these two stations spontaneously, leading to a degenerate molecular shuttle. While two binding sites are different, depending on the binding affinities between binding sites and wheel component, two different equilibrating co-conformations would co-existed. In this case, by using specific stimuli to modulate the differences in binding affinities, the controllable shuttling of the wheel component between two binding sites would be feasible, resulting in a non-degenerate controllable molecular shuttle. Since the Stoddart group reported the first degenerate molecular shuttle in 1991 (Anelli et al., 1991) and the first non-degenerate controllable molecular shuttle in 1994 (Bissell et al., 1994), the investigations of molecular shuttles have been widely carried out. Research mainly focuses on two aspects: how to effectively control the shuttling processes and how to realize practical applications of molecular shuttles (Lee et al., 2009; Young et al., 2014; Corra et al., 2019; Wu et al., 2020).
Several methods, such as pH changes, redox stimuli, cation or anion bindings, solvent changes, and light irradiation have been envisioned to trigger motions in molecular shuttles, making them excellent candidates as novel molecular machines (Xue et al., 2015; Kassem et al., 2017; Wang Q. et al., 2018). Among these external stimuli, light is extraordinarily attractive since it offers several distinct advantages: light is directional and can be induced to a precise area, many photochemical reactions do not generate any byproducts, the wavelength could be well modulated to affect only certain functional groups, and remote regulation can be easily realized (Balzani et al., 2009; Silvi et al., 2011). The light irradiation could induce changes in molecular skeleton structures, initiate photochemical reactions, or trigger photoinduced electron transfer in specific systems, and thereby drives the macrocyclic wheels to shuttle between different binding sites (Berná et al., 2005; Chatterjee et al., 2006; Raiteri et al., 2008). Meanwhile, the reversible shuttling movements of macrocyclic wheels can lead to remarkable changes in the chemical microenvironment and modify the electrical or photophysical properties of rotaxanes, which can be exploited in optical information storage, catalysis, drug delivery, ion transport, and so on.
Light-driven molecular shuttles have developed rapidly in the last decades, and they play pivotal roles in more fields. In recent years, several reviews on photoactivated artificial molecular machines have been demonstrated (Bléger and Hecht, 2015; Qu et al., 2015; Wang et al., 2018a; Yu S. et al., 2019; Groppi et al., 2019; Baroncini et al., 2020; Corra et al., 2020). However, we find that the contents of light-driven molecular shuttles are only sporadically distributed. Considering the important contribution of light-driven molecular shuttles to the studies of supramolecular systems particularly molecular machines, this review systematically summarizes the common light-driven molecular shuttles according to different operating mechanisms and briefly introduces the practical applications based on them. Considering that there have already been literatures which reviewed the early research results (Ma et al., 2009; Silvi et al., 2009), more attention is mainly focused on the literature after 2010.
Azobenzene (AB) derivatives have been widely investigated as photoresponsive compounds (Chen et al., 2020). Their configurations can be changed between E and Z forms corresponding to visible light and UV irradiations, which can be utilized to construct light-responsive molecular shuttles. With AB as a photoresponsive unit in the main axle, the host macrocyclic molecules are mostly α-cyclodextrin (α-CD) in early studies (Murakami et al., 2005; Qu et al., 2005; Coskun et al., 2009). Developing other macrocycles to modify host-guest interactions is of great importance. During the past few years, several macrocycles such as cucurbituril and pillar [6]arene have been employed as wheel components for the construction of novel AB-based molecular shuttles, which would further expand their practical applications.
Zhu et al. (2012) reported a light-driven molecular shuttle based on cucurbit [7]uril ([3]rotaxane 1, Figure 1A). The [3]rotaxane 1 contains two cucurbit [7]uril macrocycles and a dumbbell component composed of an E-AB unit as well as two viologen units as two recognition sites. Under E-1 isomer state (corresponding to 430 nm irradiation), the shuttling scope of two cucurbit [7]uril rings was expanded from the viologen subunits to the phenyl subunits of AB unit. With 365 nm light irradiation, the shuttling equilibrium distribution in Z-1 was diverted from the AB units to the viologen units arising from the steric hindrance after the trans-to-cis photoisomerization of the AB unit.
FIGURE 1. Chemical structures of molecular shuttles based on azobenzene units and schematic representations of their light-driven shuttling processes. (A) [3]Rotaxane 1. Adapted with permission from Zhu et al. (2012). Copyright (2012) American Chemical Society. (B) [2]Rotaxane 2. Adapted with permission from Ogoshi et al. (2020). Copyright (2020) Royal Society of Chemistry. (C) [2]Rotaxanes 3a and 3b. Adapted with permission from Zhan et al. (2016). Copyright (2016) Royal Society of Chemistry. (D) Tristable [3]rotaxane 4. Adapted with permission from Li et al. (2021). Copyright (2021) Royal Society of Chemistry.
Ogoshi et al. (2020) designed a light-operated molecular shuttle ([2]rotaxane 2, Figure 1B) based on pillar [6]arene. In their study, the AB unit served as a photo-gate to modulate the shuttling behavior of pillar [6]arene macrocycle. In the E-2 form, the pillar [6]arene macrocycle could shuttle freely and reversibly between two recognition sites, while slow or no shuttling occurred in the Z-isomer. The above shuttling processes could be qualitatively characterized by 1H-NMR spectrum and spectral methods, such as UV-vis absorption spectrum and fluorescence spectrum.
Zhan et al. (2016) employed the famous “blue box”, namely cyclobis (paraquat-p-phenylene) (CBPQT4+) macrocycle, as an acceptor host unit to construct an AB-based molecular shuttle (Figure 1C). Initially, they designed and synthesized two [2]rotaxanes 3a and 3b (Figure 1C), which were composed of an AB subunit and a 1,5-dioxynaphthalene (DNP, for 3a) subunit or a 3,3′-difluorobenzidine (DFBZ, for 3b) subunit as donor recognition stations, respectively. The CBPQT4+ ring could be modulated to be trapped at the AB subunit or the DNP subunit (or the DFBZ subunit) upon the photoinduced trans-cis isomerization of the AB unit. Importantly, when the CBPQT4+ ring was residing on the DNP or the DFBZ site, the charge-transfer (CT) absorption bands of DNP⊂CBPQT4+ (λmax = 560 nm) or DFBZ⊂CBPQT4+ (λmax = 650 nm) complex were outside of the photochromically active absorption bands (365 and 420 nm), making it be potentially meaningful for the construction of non-destructive readout systems in optical information storage. Based on these results, they further synthesized a [3]rotaxane 4 (Figure 1D), in which the AB subunit and the naphthalene-derived azobenzene (NP-AB) subunit were linked by a DNP subunit (Li et al., 2021). The trans-to-cis isomerizations of AB (λ = 365 nm) and NP-AB (λ = 420–430 nm) units correspond to lights of different characteristic wavelengths, and thus [3]rotaxane 4 is a unique orthogonal photoresponsive tristable molecular shuttle, which is of great importance for realizing complicated functionality and advanced applications.
Attributed to the synthetic convenience and facile regulation of molecular recognition properties, the AB-based rotaxanes described above are the most studied light-driven molecular shuttles. However, due to the visible light responsive characteristic of the AB unit, the corresponding Z-isomer usually fades once exposed to visible light that exists widely in natural and artificial environments, thus limiting their applications in data storage, optical displays, etc. To address the problem of the visible light responsive property of the AB unit, the development of photofunctional groups that respond to wavelengths outside the visible light window is of great importance. Zhan et al. (2018) reported two bidirectional photoswitchable molecular shuttles ([2]rotaxanes 5a and 5b, Figure 2A) with long-term visible light stability with the aid of a stiff stilbene (SB) unit based on the trans−cis isomerization of the C=C double bond.
FIGURE 2. Chemical structures of molecular shuttles by virtue of a C=C double bond and schematic illustrations of their light-driven shuttling processes. (A) [2]Rotaxanes 5a and 5b. Adapted with permission from Zhan et al. (2018). Copyright (2018) Royal Society of Chemistry. (B) [1]Rotaxane 6. Adapted with permission from Wang et al. (2018). Copyright (2018) Royal Society of Chemistry. (C) [1]Rotaxane 7. Adapted with permission from Yu et al. (2019). Copyright (2019) American Chemical Society.
As displayed in Figure 2A, the SB unit is solely UV light sensitive, which can perform trans–cis photoisomerization upon distinguished wavelength irradiations. The SB unit, DNP (for 5a) or biphenyl (BP) (for 5b) units were incorporated into the axle components of [2]rotaxanes as different recognition stations, while the CBPQT4+ macrocycle was selected as a wheel component. Upon irradiation with UV light (λ = 340 nm), the SB unit underwent trans-to-cis photoisomerization, driving the CBPQT4+ macrocycle to shuttle from the SB unit to the DNP unit (45%) or the BP unit (48%). When irradiated by another UV light (λ = 375 nm), the CBPQT4+ ring moved back to the E-SB unit owing to the cis-to-trans isomerization of the SB unit (92 and 91% for 5a and 5b, respectively). Further time lapse absorption spectra indicated that both Z-5a and Z-5b could maintain long-term stability when their UV light irradiated solutions were exposed to visible light environment for several days.
The above-mentioned examples of photoisomerization of N=N or C=C based molecular shuttles are mostly exploited steric hindrances to block the translation motion of the macrocyclic wheel. Wang Y. et al. (2018) demonstrated another alternative method for gaining light-driven translation of one molecular subunit relative to the other one based on a [1]rotaxane 6 (Figure 2B). As shown in the figure, the pillar [5]arene macrocycle was connected with the SB unit by a proper linker in [1]rotaxane 6, so the SB unit could serve as a functional group to induce the movement of pillar [5]arene wheel and concomitantly act as a stopper to prevent the macrocycle from dethreading. The movement of pillar [5]arene unit arises from the contraction or elongation of the SB unit upon its trans-cis photoisomerization under distinguishable UV light irradiations.
Based on similar molecular design, Yu J.-J. et al. (2019) also demonstrated different motion modes could be combined in an MIM ([1]rotaxane 7, Figure 2C), and successfully achieved a four-step operating cycle. When irradiated with different UV lights, the trans-cis photoisomerization of the C=C double bond in the S−S molecular motor rendered the dibenzo-24-crown-8 (DB24C8) ring to shuttle between benzylalkylammonium hexafluorophosphate (BAA) and N-methyltriazolium hexafluorophosphate (MTA) recognition stations, giving unstable E-7 or unstable Z-7. The subsequent heating processes could induce helix inversion and convert the unstable species to the corresponding stable states.
The examples of 6 and 7 clearly provide evidence that a molecular motor can work against noncovalent interactions in MIMs and the movement of the rotaxane can be induced by a transmission mechanism, which gives deeper insights into the operating capacity in more complicated molecular machines.
Usually, the light-driven molecular shuttles would meet several problems, such as low positional integrity, only stable under special environment, and poor switching fidelity. Benefiting from the photoinduced trans−cis isomerization of the C=N double bond, Leigh et al. (2017) reported a molecular shuttle ([2]rotaxane 8, Figure 3) that could overcome all these problems. The highly directional hydrogen bonds between benzylic amide macrocycle and recognition sites (pyridyl-acyl hydrazone unit or succinic amide ester unit) were the main driving force. The pyridyl-acyl hydrazone unit could present trans-cis isomerization with extra light or thermal stimuli in high efficiency. In the Z-8 form (under 312 nm UV light stimulus), the pyridyl and hydrazone units would form a six-membered intramolecular hydrogen bond system. Owing to the significantly different binding affinities of E/Z forms of pyridyl-acyl hydrazone for a wheel component, the macrocyclic wheel could reside on the thread with high positional integrity (>95%). The isomerization efficiencies of both Z-8 and E-8 exceeded 90%, which represented one of the highest switching fidelities reported. Notably, in contrast to the trans-cis isomerizations of N=N and C=C double bonds, both of which could be solely operated by light with different wavelengths, the cis−to−trans isomerization of the abovementioned C=N double bond needs an external addition of strong polar solvent to break hydrogen bonds, which might have an adverse effect on multiple cycle operations.
FIGURE 3. Synthesis and light-driven switching processes of axle and molecular shuttle [2]rotaxane 8. Adapted with permission from Leigh et al. (2017). Copyright (2017) American Chemical Society.
For some specific functional groups, they can undergo photochemical bond-cleavages upon light irradiation and return to original states with different stimuli, such as light, heat, pH, and so on (Baroncini et al., 2020). These attractive properties are quite suitable for the construction of light-driven molecular shuttles. The pioneering works were from Abraham’s group who have successfully achieved light-driven molecular shuttles by means of the photoheterolysis of methoxy-substituted cycloheptatriene (Abraham et al., 2004) and methoxy-substituted acridane (Abraham et al., 2007).
Inspired by the photochemical bond-cleavage strategy, Gao et al. (2017) successfully triggered a novel molecular shuttle ([2]rotaxane 9, Figure 4A) by a photoactive carbonate ester moiety. As displayed in the figure, the symmetrical [2]rotaxane 9 has two succinimide units as identical recognition stations. A photoresponsive bulk barrier was chemically bonded into the middle of the axle to prevent the dynamic shuttling of the wheel component, leading to a “gated” state where the macrocycle has a definite location at one of two recognition sites. Upon irradiation of UV light, the bulk barrier was removed by the complete photoheterolysis of the carbonate ester, resulting in the subsequent recovery of fast shuttling movement of the macrocycle between two identical recognition sites, which was referred to as the “open” state ([2]rotaxane 10). The reverse open-to-gated process could be modulated via a reconnecting of the trigger into rotaxane by virtue of molecule 11.
FIGURE 4. Light-driven molecular shuttles based on photochemical reactions. (A) Photoheterolysis and rebonding reactions of [2]rotaxanes 9 and 10. Adapted with permission from Gao et al. (2017). Copyright (2017) American Chemical Society. (B) Reversible cycloaddition reactions of [2]rotaxanes 12 and 13. Adapted with permission from Yang et al. (2021). Copyright (2021) Elsevier.
Yang et al. (2021) reported a molecular shuttle ([2]rotaxane 12, Figure 4B) modulated by a photoinduced cycloaddition reaction. As illustrated in Figure 4B, the axle of rotaxane 12 bears a BP unit and a photoswitchable AB unit as different recognition stations, which are bridged via a naphthalene (NAP) subunit. In the “open” state, the CBPQT4+ macrocycle was initially trapped at the AB unit, and it could be reversibly triggered to shuttle between two recognition stations by the trans-cis isomerization of the AB unit upon UV/visible light. The free shuttling motion would be predominantly blocked by the introduction of steric hindrance generating from the green-light induced cycloaddition reaction between the NAP subunit and triazolinedione. Upon staying in the dark, the cycloaddition product autonomously underwent disassociation, contributing to the recovery of light-driven shuttling capability of the macrocycle.
These above-discussed examples clearly indicate that the construction of molecular shuttles via photochemical reactions can provide novel approaches to enhance the shuttling controllability of rotaxane. Notably, there are still some key issues that need to be addressed for a photochemical reaction strategy. For instance, the addition reaction is incomplete (i.e., 79% for 9) because it is a bimolecular process, while the reverse bond-cleavage process is very efficient due to it is a unimolecular reaction. The employment of more efficient and reversible photochemical reactions will be in great demand for the further expansion of this novel type of light-driven molecular shuttles.
Electron transfer is essentially a redox reaction and plays a key role in many important life processes. In addition to be driven by redox agents, many electron transfer processes could be actuated photochemically, which have been successfully demonstrated for light-driven molecular shuttles.
Brouwer et al. (2001) creatively reported a benzylic amide-based molecular shuttle ([2]rotaxanes 16, Figure 5A) which responded to photochemical stimuli. The molecular shuttle 16 consists of a benzylic amide ring interlocked onto an axle that bears a succinamide (succ) unit and a 3,6-di-tert-butyl-1,8-naphthalimide (ni) unit as two potential hydrogen bonding sites. The benzylic amide ring was first trapped at the succ station due to the bifurcated hydrogen bonds between the isophthalamide groups in the wheel component and the two amide carbonyls in the succ unit. After photoexcitation, the ni chromophore underwent intersystem crossing to its triplet (T) state, and subsequently formed an ni radical anion when reducted by an electron donor reagent (DABCO). The ni radical anion had an enhanced H-bond–accepting affinity for the benzylic amide ring, rendering the ring to move from the succ site to the ni site. The ni radical anion could be reversibly converted to the neutral state upon recombination with the DABCO radical cation, making the system return to the ground state and the amide macrocycle shuttle back to the succ site.
FIGURE 5. (A) Chemical structures of [2]rotaxanes 14∼17 and their light-driven shuttling behaviors between different states. Adapted with permission from Panman et al. (2010). Copyright (2010) AAAS Science. (B) Chemical structure of [2]rotaxane 18 and the proposed “harpooning” mechanism for the shuttling process. Adapted with permission from Kumpulainen et al. (2019). Copyright (2019) American Chemical Society. (C) Chemical structure of [2]rotaxane 19 and the photoswitchable feature based on radical–radical interactions. Adapted with permission from Li et al. (2011). Copyright (2011) Wiley-VCH Verlag GmbH & Co. KGaA.
The shuttle processes were originally confirmed by time resolution transient absorption spectroscopy and 1H-NMR spectroscopy, both of which did not allow for the inspection of singlet state with short lifetime. Subsequently, they developed an effective method to gain more insight into the shuttling mechanism using time-resolved vibrational spectroscopy by virtue of the distinguishable CO-stretch frequencies of carbonyl groups in different states (Panman et al., 2010; Panman et al., 2012). The shuttling of macrocycle was found to undergo two steps: photothermally driven escaping from the initial site (succ), followed by fast movement along the axle ending either at the succ unit or the ni unit. Through varying the length of the alkyl spacer (14∼17, n = 5, 9, 12, 16, Figure 5A), the rapid motion was further confirmed as a biased one-dimensional random walk mechanism (Panman et al., 2010).
Besides the alkyl spacer, the effect of solvent was also systematically investigated. In particular, the experiments were performed in viscous polymer solutions, and the photoinduced shuttling rates were found to be slowed down in polymer solutions (Jagesar et al., 2009). The retardation effect could be reasonably explained by a hydrodynamic scaling model, which was correlated to the presence of more entanglements of polymer chains in viscous solutions. In 2013, water was found to be acting as a molecular lubrication to significantly accelerate the shuttling rates, which was ascribed to the 3D hydrogen-bond network formed between the shuttling parts of molecular shuttle (Panman et al., 2013).
In 2019, the effect of axle and stopper on the shuttling rates was proved (Kumpulainen et al., 2019). As shown in Figure 5B, in comparison to 14∼17, the alkyl spacer and 3,6-di-tert-butyl-1,8-naphthalimide were replaced by a polyether segment (PE, C6O2) and a novel 3,6-dihydroxy-1,8-naphthalimide (dOH-NI) unit in [2]rotaxane 18. Both PE and dOH-NI units were proved to accelerate the shuttling rates, but the operating mechanisms were different. The acceleration of the PE unit was ascribed to the decrease of activation energy because of an early transition state, in which the benzylic amide ring was partly bonded to the ether functional group. The acceleration of the dOH-NI unit could be interpretated by a harpooning mechanism. In other words, the transition state involved a folded conformation on account of the hydrogen bonding interactions between macrocycle and dOH-NI unit.
Most of the above-mentioned molecular shuttles were performed in organic solvents. In consideration to gain more insight into the functions of biological molecular machines which are mainly operated in aqueous media, molecular shuttles operated in aqueous conditions are attractive. Li et al. (2011) demonstrated a light-driven molecular shuttle ([2]rotaxanes 19, Figure 5C) driven by radical–radical interactions in aqueous solution. The [2]rotaxane 19 contains a CBPQT4+ macrocycle and an axle bearing a DNP unit and a 4,4′-dialkylbipyridinium (BIPY2+) unit as distinct recognition sites. Meanwhile, the dumbbell component has an [Ru (bpy)3]2+ complex as one stopper. The CBPQT4+ macrocycle initially encircled the π-electron-rich DNP unit. Upon irradiation with visible light, all three BIPY2+ units were reducted to radical cation states (BIPY•+) by the excited [Ru (bpy)3]2+ complex, after which the Ru element was reducted from RuIII state to RuII state by a sacrificial reagent, such as triethanolamine (TEOA). The resultant diradical dicationic CBPQT2(•+) underwent movement to encircle the BIPY•+ unit owing to the stabilizing radical–radical interactions between the CBPQT2(•+) unit and the BIPY•+ unit, as well as the loss of D–A interactions between the DNP station and the CBPQT2(•+) macrocycle. When the BIPY•+ units were oxidized by external oxygen, the recovery of D–A interactions and the Coulombic repulsion between CBPQT4+ macrocycle and BIPY2+ unit rendered the ring to shuttle back to the DNP unit. All the processes could be operated in aqueous solution and were found to be reproducible with no evidence of obvious decomposition detected over five operating cycles.
Light-controlled switching of a non-photoresponsive rotaxane will be undoubtedly challenging since axle component and wheel component are both non-photoactive. However, light can change the microenvironments around rotaxanes, leading to an indirect switching of molecular shuttle. Yang et al. (2017) reported the first example of light-controlled operation of a non-photoresponsive molecular shuttle ([2]rotaxane 20, Figure 6) stemming from a photoinduced proton-transfer (PIPT) strategy.
FIGURE 6. (A) Chemical structure and schematic diagram of acid−base based molecular shuttle [2]rotaxane 20; (B) the light-driven shuttling of non-photoresponsive 20 by a photoacid 21. Adapted with permission from Yang et al. (2017). Copyright (2017) American Chemical Society.
As shown in Figure 6A, [2]rotaxane 20 is essentially an acid/base-switchable molecular shuttle, in which the dibenzo-24-crown-8 (DB24C8) macrocycle can be controllably switched between ammonium station and N-methyltriazolium station, corresponding to a protonated state and a deprotonated state, respectively. Compound 21 is an indazole-based photoacid that could behave as photoinduced acidity with good reversibility. After irradiation of a 1.2:1 mixture of 21 and 20a in DMSO-d6 solution for 5 min, 21 was completely converted to 22 and meanwhile released a proton to induce the shuttling movement of the macrocycle (Figure 6B), which was confirmed by fluorescent and NMR spectra. However, the inverse process was not implemented, which was presumably due to the strong binding affinity of 20b for proton.
To improve the reversibility of light-driven processes, several factors, such as the host-guest interactions between macrocycle and recognition sites, the proton binding capacity of recognition sites and the acidity of photoactive small molecule must be compatible. Shi et al. (2018) verified a reversible regulation of the PIPT process through rational molecular design. As illustrated in Figure 7, in [2]rotaxane 23a, 2,6-helic [6]arene macrocycle was selected as wheel component while alkyl group and 2-phenylpyridium served as two recognition stations. Besides, molecule 26 was chosen as photoacid. Initially, the macrocycle was bound to the alkyl group due to the C–H···π interactions in solution (CD3OD/CD2Cl2 = 11:5). After irradiation with 420 nm or sunlight for 5 min, the photoacid 26 could protonate the pyridine unit of 23a and therefore induce the macrocycle to shuttle to the pyridium site. Thanks to the appropriate host-guest interactions and proton binding capability of the pyridium site, the macrocycle moved back to the alkyl moiety after the solution was treated under dark conditions for 3.5 h. Therefore, the system enabled a reversible protonation and deprotonation cycle which was implemented by alternating the irradiation and dark conditions. Notably, the light-driven process of 23 could be operated more than 50 times with perfect reproducibility without any waste products. Apart from the switching motion of 23, the oscillation motion of 24 and the palindromic motion of 25 could also be accomplished by the PIPT strategy.
FIGURE 7. (A) Chemical structures of rotaxanes 23, 24, and 25; (B) principle of photogenerated acid; (C) the light-driven motion of [2]rotaxane 23 by PIPT strategy. Adapted with permission from Shi et al. (2018). Copyright (2018) Royal Society of Chemistry.
The aforementioned PIPT process involves a photoacid intermediate, which is unfavorable for pH-sensitive substrate. Tron et al. (2017) proved the regulation could be operated under the neutral state by virtue of a photoinduced cyclomerization reaction of anthracene. As depicted in Figure 8, compound 28 strongly bound with barbital (B) in its open form with six hydrogen bonds in the initial state. Photoirradiation of 28 induced an intramolecular anthracene cyclomerization reaction to produce 28c, and thus B was released and transferred to bind with two diamidopyridine recognition sites of [2]rotaxane 29 which had an affinity for B laying between 28 and 28c. Therefore, the amplitude of macrocycle shuttling was directly restricted to a small range. The reverse process was investigated by heating the system to 110°C, leading to the retrocyclomerization of 28c and the recovery of a large translational movement of macrocycle.
FIGURE 8. Schematic representation of the photoregulated translational movement of a macrocycle in [2]rotaxane 29 via compound 28. Adapted with permission from Tron et al. (2017). Copyright (2017) American Chemical Society.
Light-driven non-photoresponsive molecular shuttles are essentially photochemical reaction processes. In comparison to the abovementioned photochemical reaction strategy, the positions of the photochemical reactions are different. The photochemical reactions discussed in this section occur on other substrates, rather than rotaxanes, thus resulting in an indirect approach. However, these two approaches also have common characteristics: photochemical reactions involving the breaking of old bonds and the formation of new ones. Therefore, the whole process consists of several unimolecular reactions and bimolecular reactions. Different reactions will vary greatly in reaction rates, and thus some processes require additional operations, such as prolonging reaction time or heating.
Molecular shuttles can be switched between different bistable or multi-states in response to external stimuli, which is similar to contemporary integrated systems and devices, so light-driven molecular shuttles can be potentially utilized for optical information storage (Zhang et al., 2012). Apart from thermodynamically different stable states, a convenient, efficient, and reversible shuttling kinetics is also of great importance. Avellini et al. (2012) designed and synthesized a multi-stimuli responsive molecular shuttle ([2]rotaxane 30, Figure 9A) to investigate its photoinduced memory effect, which could be thermodynamically transformed between two states and kinetically controlled by photochemical means.
FIGURE 9. (A) Chemical structure of [2]rotaxane 30; (B) memory switching cycle of [2]rotaxane 304+ triggered by chemical and photochemical methods. Adapted with permission from Avellini et al. (2012). Copyright (2012) Wiley-VCH Verlag GmbH & Co. KGaA.
The [2]rotaxane 304+ contains a CBPQT4+ macrocycle as a wheel component, a tetrathiafulvalene (TTF) unit and a DNP unit as the initial and second recognition stations, and a 3,5,3′,5′-tetramethylazobenzene (TMeAB) unit which could be switched between Z and E configurations upon light-stimuli. A “write-lock-erase” experiment was conducted to investigate the light- and redox-induced memory switching cycle of [2]rotaxane 304+. As can be seen in Figure 9B, the switching cycle was composed of four sections, and detailed experimental results suggested that [2]rotaxane 304+ could serve as a bistable memory material under reasonable kinetic control. After the oxidation writing session, the light irradiation successfully locked the data and protected the nonoxidized species from accidental writing, and then the oxidized rotaxanes could be reducted back to the initial state without losing the written information for several hours. In other words, the written data could be stored for a remarkably long time under dark conditions and room temperature until the thermal-induced cis-to-trans isomerization of TMeAB occurred, which was different from other thermodynamically controlled molecular shuttles in which the metastable states co-conformations usually had short lifetimes.
Gaining catalysts with switchable activity to simulate the enzymatically driven processes is a growing research topic nowadays. MIMs can be controllably switched between different states and thus they are promising candidates for novel switchable catalysts. Notably, pH-driven molecular shuttles have been successfully employed for controlling the catalytic activities of several particular reactions (Blanco et al., 2014a; Blanco et al., 2014b; Eichstaedt et al., 2017). In 2017, Martinez-Cuezva et al. (2017) first reported a light-driven molecular shuttle ([2]rotaxane 31, Figure 10A) acted as a cocatalyst component for chalcogeno-Baylis–Hillman reaction.
FIGURE 10. (A) Chemical structure and light-driven switching of [2]rotaxane 31; (B) schematic representation of the photoswitchable catalyst of 31. Adapted with permission from Martinez-Cuezva et al. (2017). Copyright (2017) Royal Society of Chemistry.
The molecular shuttle 31 is a tetralactam-based [2]rotaxane which contains four carbonyl groups that can form hydrogen bonds with a guest molecule. One recognition site of the axle is composed of a photoactive fumaramide, and the other is a sulfur-containing thiodiglycolamide which has two carbonyl groups as hydrogen bonding acceptors and an embedded sulfur atom as catalytic active center. The [2]rotaxane 31 was utilized as cocatalyst to catalyze the Baylis–Hillman reaction in CH2Cl2 solution (Figure 10B). When exposed to UV light of 312 nm, the macrocycle was chiefly located at the fumaramide site and the thiodiglycolamide was exposed to the surrounding reaction substrate, thus the sulfur atom could reveal catalytic activity, leading to a better control of the reaction (E/Z = 80:20). Upon light irradiation at 254 nm, the photoisomerization of trans C=C double bond switched the fumaramide unit to its cis counterpart maleamide and the macrocycle was mainly positioned at the thiodiglycolamide unit owing to the intramolecular hydrogen bond. Therefore, the sulfur atom was locked inside the ring and the catalytic reaction produced a 1:1 mixture of both isomers. Notably, the reaction conversion using the two-station [2]rotaxane decreased in comparison to those obtained using noninterlocked catalysts, but this example first showed that the utilization of photoswitchable interlocked catalysts could be meaningful to control the stereochemical issue of an organic reaction.
Apart from the strategy of locking catalytic active center, Dommaschk et al. (2019) demonstrated a local symmetry breaking approach to construct photoswitchable catalysts. As depicted in Figure 11A, the axle of [2]rotaxane 33 contains a 2,5-disubstituted pyrrolidine unit which bears a pyridyl-acyl hydrazone and a glycine amide attached to either side of it. The pyridyl-acyl hydrazone unit is photoactive since it can present trans-cis isomerization under different light stimuli. Initially, both E- and Z-32 could be treated as pseudo-meso structures owing to the nearly symmetrical environment in the axle. Interestingly, the macrocycle would break the local symmetry of the pyrrolidine unit when the free axle was assembled into rotaxane. The [2]rotaxane presented mainly “enhanced-(R)” or “enhanced-(S)” form when the tetralactam-based macrocycle was bound to the E-acyl hydrazone unit or the glycine amide unit under pH or light irradiation stimuli. Both the free thread 32 and [2]rotaxane 33 were applied to catalyze the conjugate addition of aldehydes to vinyl sulfones (Figure 11B). Both E-32 and Z-32 did not show notable selectivity (2–14% ee of 35), whereas the rotaxane 33 exhibited selectivity closer to 40% ee with the switching position of the macrocycle. Therefore, it strategically enabled the successful achievement of an anti-divergent catalysis with a single photoswitchable rotaxane catalyst.
FIGURE 11. (A) Chemical structure of thread E-32 and shuttling behavior of [2]rotaxane 33 based on the trans–cis photoisomerization of C=N double bond; (B) the asymmetric catalysis of [2]rotaxane 33 for addition reaction. Adapted with permission from Dommaschk et al. (2019). Copyright (2019) Wiley-VCH Verlag GmbH & Co. KGaA.
Mesoporous silica nanoparticles (MSNPs) have been applied for drug delivery, but several challenges remain to be resolved: achieving released drugs without side effects, realizing controlled release in vivo, and improving release efficiency (Ambrogio, et al., 2011). Yan et al. (2012) introduced a photothermal-responsive molecular shuttle to functionalize MSNPs that could successfully overcome these issues. As illustrated in Figure 12, the MSNPs were covalently attached with α-CD based [2]rotaxane 36 which contained AB and triazole/ethylene glycol units as different recognition sites, and a naphthalene disulfonate to provide solubility and as a stopper unit. The remote-controlled drug release is contributed by the shuttling movement of α-CD ring originating from the photothermal-driven trans–cis isomerization of an AB unit. First, the α-CD ring was trapped at the E-AB unit, which was away from the MSNPs surface. Subsequently, the α-CD ring shuttled to the triazole/ethylene glycol unit owing to the trans-to-cis isomerization of the AB unit upon UV light irradiation, and the drugs were effectively blocked into the pores of nanoparticles. The high capping efficiency was confirmed by the release of RhB-loaded MSNPs in the dark for 24 h, since a negligible amount of RhB was detected to leach. Finally, the cis-to-trans isomerization of an AB unit under visible light or heating stimuli made the a-CD ring shift back to the E-AB unit, resulting in drug delivery. Furthermore, curcumin-loaded MSNPs were successfully injected into zebrafish embryos for drug release in vivo, and thermal-triggered release of curcumin was shown to be more effective. These results suggest that the MSNPs decorated with photothermal-responsive molecular shuttle are excellent drug carriers for therapeutic applications.
FIGURE 12. Graphic diagram of the injection of drug-containing MSNPs functionalized with [2]rotaxane 36 into zebrafish larvae for drug delivery in vivo. Adapted with permission from Yan et al. (2012). Copyright (2012) Wiley-VCH Verlag GmbH & Co. KGaA.
Ion transports across the lipid bilayers play crucial roles in many biological processes. Developing artificial carriers or channels to simulate the ion transports in living organisms would improve the understanding of working mechanisms of life processes. An artificial pH-driven molecular shuttle operating in lipid bilayers for ion transport has been reported, but the gated ion transport is not implemented (Chen et al., 2018). Inspired by the working mechanism of Channelrhodopsins (ChRs), Wang et al. (2021) designed a light-driven molecular shuttle ([2]rotaxane 37, Figure 13) with an attractive light-gated ion transport behavior.
FIGURE 13. (A) Molecular structures of molecular shuttle 37, amphiphilic thread 38, and macrocycle 39; (B) the possible mechanism for light-gated ion transport of 37 across biomembranes. Adapted with permission from Wang et al. (2021). Copyright (2021) Wiley-VCH Verlag GmbH & Co. KGaA.
In their study, the [2]rotaxane 37 consisted of an AB-based amphiphilic axle (38) with two ammonium ions as recognition sites as well as a wheel component (39) bearing a benzo [18]crown-6 (B18C6) macrocycle as a K+ selective receptor. The space length (3.43 nm) between the two ammonium stations of 37 was close to the thickness of the phospholipid bilayer, thus it enabled the proper membrane-spanning movement of rotaxane. Large unilamellar lipid vesicles (LUVs) assay and lipid bilayer membranes (BLMs) experiments were conducted to explore the ion transport activity as well as the gated behavior of 37. The experimental results indicated that the ring could reversibly shuttle between two ammonium stations when the AB unit was in its E-form (corresponding to visible light irradiation), leading to the efficient K+ ion transport. After UV light irradiation, the corresponding Z-37 exhibited much lower transmembrane activity owing to the limited movement of the macrocycle in the Z-isomer. Notably, this example is the first MIM used for light-gated ion transport based on a synthetic molecular shuttle.
By combining the molecular shuttle and doubly interlocked rotaxane dimers (i.e., molecular [c2]daisy chains), the resultant MIMs can behave with stimuli-responsive contraction and extension motions. Such systems have been coined as “molecular muscles” owing to it is similar to the sarcomeres present in muscles (Bruns and Stoddart, 2014; Antoine et al., 2019). In early reports, Dawson et al. (2008) and Li et al. (2010) had already proved that single molecular [c2]daisy chains with stilbene or AB moieties could be utilized as light-driven molecular muscles in solutions.
To overcome the challenge of macroscopic molecular actuators, Iwaso et al. (2016) designed a polymer network (Figure 14A, 40) crosslinked with AB and α-CD based molecular [c2]daisy chains. Hydrogel and xerogel were prepared from 40, and a photoresponsive volume change of hydrogel was first investigated. Upon UV light exposure, obvious volume reduction of the resultant polymer gels was observed, which could recover to the initial state after irradiation with visible light for 3 h (Figure 14B). When hydrogel and xerogel were carried out photoresponsive actuation experiments, both of them bended toward the UV light source, which was attributed to the sliding movement of the molecular [c2]daisy chain units triggered by the trans-to-cis photoisomerization of the AB unit (Figure 14C). Thanks to the higher density of photo-responsive molecular muscle units, the response time of xerogel upon UV light irradiation was very rapid (7° per second), which was more than 10,000 times faster than that of hydrogel (7° for 3 h). More importantly, the xerogel of 40 was successfully applied as a mechanical arm to lift a real substance using UV light irradiation as external stimulus to produce actual work. However, the 40 xerogel did not realize reversible switching by light irradiation.
FIGURE 14. (A) Chemical structure of crosslinking [c2]daisy chains 40; (B) schematic illustration of the light-driven volume change of 40 hydrogel; (C) the bending mechanism of 40 hydrogel upon photoirradiation. Adapted with permission from Iwaso et al. (2016). Copyright (2016) Springer Nature.
To further enhance the responsive speed and reversibility of macroscopic motions, the αCD-AB molecular [c2]daisy chain was subsequently replaced by a more sensitive cross-linking αCD-stilbene [c2]daisy chain (Figure 15A, 41) (Ikejiri et al., 2018). The trans-stilbene moieties in the [c2]daisy chains could reversibly form dimeric aggregation and be dissociated by photoisomerization (Figure 15B). When subjected to 350 nm irradiation, the 41-based dry gel (molecular weight: 20,000) rapidly bended to 26.0° within 3 s, and it returned to its original state within 3 s upon 280 nm irradiation, leading to a reversible actuation process (Figure 15C). Actually, the hydrophobic feature of the stilbene unit might improve the reversible switching ability of [c2]daisy chains. Finally, the dry gels could perform quick and heavy mechanical work upon light irradiation, which picked up an object 15 times heavier than its own weight.
FIGURE 15. (A) Synthesis of cross-linking [c2]daisy chains 41; (B) the photoisomerization diagram of stilbene-based 41; (C) the repeatable bending of 41 xerogel by UV with different wavelengths. Adapted with permission from Ikejiri et al. (2018). Copyright (2018) American Chemical Society.
As typical molecular machines, molecular shuttles could produce nanoscale mechanical shuttling motions of wheel components in response to external stimuli, making them privileged platforms for the construction of artificial molecular machines. In particular, light is a very attractive resource to drive molecular shuttles owing to its clean, cheap, directional, and controllable features, thus light-responsive molecular shuttles have attracted more attention. The processes of light-driven molecular shuttle motions involve several mechanisms: photoinduced isomerization, photochemical reaction, and photoinduced electron transfer. The photoinduced isomerization could be gained based on N=N double bond, C=C double bond, or C=N double bond. Several reversible photochemical reactions have been successful in constructing molecule shuttles, and one obvious feature of photochemical reactions is that they would release or require the addition of small molecular reagents to complete the whole reversible processes. Moreover, photochemical reactions can sometimes change the microenvironments surrounding rotaxanes, which could be applied to drive non-photoresponsive molecular shuttles. The photoinduced electron transfers usually exist in donor-acceptor systems and need sacrifice reagents to assist supplementary completion. Over the past decade, researchers pay more attention to realize the practical applications of light-driven molecular shuttles. The light-driven molecular shuttles have been applied in optical information storage, drug delivery, catalysts for organic synthesis, ion transport, and molecular muscles, which opens a wide door to the nanoscience.
Although great achievements have been realized in this area, the further expansion of the applications of light-driven molecular shuttles will still be in great demand. Some key issues remain to be addressed: 1) improving the conversion efficiency, residence time, and switching ratio of different states; 2) expanding the reaction types, improving catalytic efficiency and selectivity, and realizing multiple recycling of catalysts; 3) achieving a wider range of biological applications, such as improving not only the controllability and types of drug release, but also the efficiency, sensitivity, and selectivity of ion transport processes; and 4) constructing proper communication bridges between microscopic molecular shuttles and macroscopic devices, and fabricating practical devices based on light-driven molecular shuttles.
All authors listed have made a substantial, direct, and intellectual contribution to the work, and approved it for publication.
This work has been supported in part by the Chongqing Science and Technology Commission (CSTC) (No. cstc2020jcyj-msxmX0821 and No. cstc2020jcyj-msxmX0711), the Chongqing Municipal Education Commission (No. KJQN201900845 and No. KJQN201800833), and Scientific Research Foundation for Advanced Talents, Chongqing Technology and Business University (No. 1956054).
The authors declare that the research was conducted in the absence of any commercial or financial relationships that could be construed as a potential conflict of interest.
All claims expressed in this article are solely those of the authors and do not necessarily represent those of their affiliated organizations, or those of the publisher, the editors, and the reviewers. Any product that may be evaluated in this article, or claim that may be made by its manufacturer, is not guaranteed or endorsed by the publisher.
Abraham, W., Buck, K., Orda-Zgadzaj, M., Schmidt-Schäffer, S., and Grummt, U.-W. (2007). Novel Photoswitchable Rotaxanes. Chem. Commun. 2007, 3094–3096. doi:10.1039/b702737k
Abraham, W., Grubert, L., Grummt, U. W., and Buck, K. (2004). A Photoswitchable Rotaxane with a Folded Molecular Thread. Chem. Eur. J. 10, 3562–3568. doi:10.1002/chem.200400243
Ambrogio, M. W., Thomas, C. R., Zhao, Y.-L., Zink, J. I., and Stoddart, J. F. (2011). Mechanized Silica Nanoparticles: a New Frontier in Theranostic Nanomedicine. Acc. Chem. Res. 44, 903–913. doi:10.1021/ar200018x
Anelli, P. L., Spencer, N., and Stoddart, J. F. (1991). A Molecular Shuttle. J. Am. Chem. Soc. 113, 5131–5133. doi:10.1021/ja00013a096
Antoine, A., Moulin, E., Fuks, G., and Giuseppone, N. (2019). [c2]Daisy Chain Rotaxanes as Molecular Muscles. CCS Chem. 1, 83–96. doi:10.31635/ccschem.019.20180023
Avellini, T., Li, H., Coskun, A., Barin, G., Trabolsi, A., Basuray, A. N., et al. (2012). Photoinduced Memory Effect in a Redox Controllable Bistable Mechanical Molecular Switch. Angew. Chem. Int. Ed. 51, 1611–1615. doi:10.1002/anie.201107618
Balzani, V., Credi, A., and Venturi, M. (2009). Light Powered Molecular Machines. Chem. Soc. Rev. 38, 1542–1550. doi:10.1039/b806328c
Baroncini, M., Silvi, S., and Credi, A. (2020). Photo- and Redox-Driven Artificial Molecular Motors. Chem. Rev. 120, 200–268. doi:10.1021/acs.chemrev.9b00291
Berná, J., Leigh, D. A., Lubomska, M., Mendoza, S. M., Pérez, E. M., Rudolf, P., et al. (2005). Macroscopic Transport by Synthetic Molecular Machines. Nat. Mater 4, 704–710. doi:10.1038/nmat1455
Bissell, R. A., Córdova, E., Kaifer, A. E., and Stoddart, J. F. (1994). A Chemically and Electrochemically Switchable Molecular Shuttle. Nature 369, 133–137. doi:10.1038/369133a0
Blanco, V., Leigh, D. A., Lewandowska, U., Lewandowski, B., and Marcos, V. (2014a). Exploring the Activation Modes of a Rotaxane-Based Switchable Organocatalyst. J. Am. Chem. Soc. 136, 15775–15780. doi:10.1021/ja509236u
Blanco, V., Leigh, D. A., Marcos, V., Morales-Serna, J. A., and Nussbaumer, A. L. (2014b). A Switchable [2]rotaxane Asymmetric Organocatalyst that Utilizes an Acyclic Chiral Secondary Amine. J. Am. Chem. Soc. 136, 4905–4908. doi:10.1021/ja501561c
Bléger, D., and Hecht, S. (2015). Visible‐Light‐Activated Molecular Switches. Angew. Chem. Int. Ed. 54, 11338–11349. doi:10.1002/anie.201500628
Brouwer, A. M., Frochot, C., Gatti, F. G., Leigh, D. A., Mottier, L., Paolucci, F., et al. (2001). Photoinduction of Fast, Reversible Translational Motion in a Hydrogen-Bonded Molecular Shuttle. Science 291, 2124–2128. doi:10.1126/science.1057886
Bruns, C. J., and Stoddart, J. F. (2014). Rotaxane-based Molecular Muscles. Acc. Chem. Res. 47, 2186–2199. doi:10.1021/ar500138u
Cai, Y., Yan, X., Wang, S., Zhu, Z., Cen, M., Ou, C., et al. (2021). Pillar[5]arene-based 3D Hybrid Supramolecular Polymer for green Catalysis in Water. Inorg. Chem. 60, 2883–2887. doi:10.1021/acs.inorgchem.0c03645
Chatterjee, M. N., Kay, E. R., and Leigh, D. A. (2006). Beyond Switches: Ratcheting a Particle Energetically Uphill with a Compartmentalized Molecular Machine. J. Am. Chem. Soc. 128, 4058–4073. doi:10.1021/ja057664z
Chen, M., Liang, S., Liu, C., Liu, Y., and Wu, S. (2020). Reconfigurable and Recyclable Photoactuators Based on Azobenzene-Containing Polymers. Front. Chem. 8, 706. doi:10.3389/fchem.2020.00706
Chen, S., Wang, Y., Nie, T., Bao, C., Wang, C., Xu, T., et al. (2018). An Artificial Molecular Shuttle Operates in Lipid Bilayers for Ion Transport. J. Am. Chem. Soc. 140, 17992–17998. doi:10.1021/jacs.8b09580
Corra, S., Curcio, M., Baroncini, M., Silvi, S., and Credi, A. (2020). Photoactivated Artificial Molecular Machines that Can Perform Tasks. Adv. Mater. 32, 1906064. doi:10.1002/adma.201906064
Corra, S., de Vet, C., Groppi, J., La Rosa, M., Silvi, S., Baroncini, M., et al. (2019). Chemical On/off Switching of Mechanically Planar Chirality and Chiral Anion Recognition in a [2]rotaxane Molecular Shuttle. J. Am. Chem. Soc. 141, 9129–9133. doi:10.1021/jacs.9b00941
Coskun, A., Friedman, D. C., Li, H., Patel, K., Khatib, H. A., and Stoddart, J. F. (2009). A Light-Gated STOP−GO Molecular Shuttle. J. Am. Chem. Soc. 131, 2493–2495. doi:10.1021/ja809225e
Dattler, D., Fuks, G., Heiser, J., Moulin, E., Perrot, A., Yao, X., et al. (2020). Design of Collective Motions from Synthetic Molecular Switches, Rotors, and Motors. Chem. Rev. 120, 310–433. doi:10.1021/acs.chemrev.9b00288
Dawson, R. E., Lincoln, S. F., and Easton, C. J. (2008). The Foundation of a Light Driven Molecular Muscle Based on Stilbene and α-cyclodextrin. Chem. Commun. 2008, 3980. doi:10.1039/b809014a
Dommaschk, M., Echavarren, J., Leigh, D. A., Marcos, V., and Singleton, T. A. (2019). Dynamic Control of Chiral Space through Local Symmetry Breaking in a Rotaxane Organocatalyst. Angew. Chem. Int. Ed. 58, 14955–14958. doi:10.1002/anie.201908330
Eichstaedt, K., Jaramillo-Garcia, J., Leigh, D. A., Marcos, V., Pisano, S., and Singleton, T. A. (2017). Switching between Anion-Binding Catalysis and Aminocatalysis with a Rotaxane Dual-Function Catalyst. J. Am. Chem. Soc. 139, 9376–9381. doi:10.1021/jacs.7b04955
Erbas-Cakmak, S., Leigh, D. A., McTernan, C. T., and Nussbaumer, A. L. (2015). Artificial Molecular Machines. Chem. Rev. 115, 10081–10206. doi:10.1021/acs.chemrev.5b00146
Gao, C., Luan, Z.-L., Zhang, Q., Yang, S., Rao, S.-J., Qu, D.-H., et al. (2017). Triggering a [2]rotaxane Molecular Shuttle by a Photochemical Bond-Cleavage Strategy. Org. Lett. 19, 1618–1621. doi:10.1021/acs.orglett.7b00393
Groppi, J., Baroncini, M., Venturi, M., Silvi, S., and Credi, A. (2019). Design of Photo-Activated Molecular Machines: Highlights from the Past Ten Years. Chem. Commun. 55, 12595–12602. doi:10.1039/c9cc06516d
Guo, H., Ye, J., Zhang, Z., Wang, Y., Yuan, X., Ou, C., et al. (2020). Pillar[5]arene-based [2]rotaxane: Synthesis, Characterization, and Application in a Coupling Reaction. Inorg. Chem. 59, 11915–11919. doi:10.1021/acs.inorgchem.0c01752
Ikejiri, S., Takashima, Y., Osaki, M., Yamaguchi, H., and Harada, A. (2018). Solvent-free Photoresponsive Artificial Muscles Rapidly Driven by Molecular Machines. J. Am. Chem. Soc. 140, 17308–17315. doi:10.1021/jacs.8b11351
Iwaso, K., Takashima, Y., and Harada, A. (2016). Fast Response Dry-type Artificial Molecular Muscles with [c2]daisy Chains. Nat. Chem 8, 625–632. doi:10.1038/nchem.2513
Jagesar, D. C., Fazio, S. M., Taybi, J., Eiser, E., Gatti, F. G., Leigh, D. A., et al. (2009). Photoinduced Shuttling Dynamics of Rotaxanes in Viscous Polymer Solutions. Adv. Funct. Mater. 19, 3440–3449. doi:10.1002/adfm.200900668
Kassem, S., van Leeuwen, T., Lubbe, A. S., Wilson, M. R., Feringa, B. L., and Leigh, D. A. (2017). Artificial Molecular Motors. Chem. Soc. Rev. 46, 2592–2621. doi:10.1039/c7cs00245a
Kumpulainen, T., Panman, M. R., Bakker, B. H., Hilbers, M., Woutersen, S., and Brouwer, A. M. (2019). Accelerating the Shuttling in Hydrogen-Bonded Rotaxanes: Active Role of the Axle and the End Station. J. Am. Chem. Soc. 141, 19118–19129. doi:10.1021/jacs.9b10005
Lee, C.-F., Leigh, D. A., Pritchard, R. G., Schultz, D., Teat, S. J., Timco, G. A., et al. (2009). Hybrid Organic-Inorganic Rotaxanes and Molecular Shuttles. Nature 458, 314–318. doi:10.1038/nature07847
Leigh, D. A., Marcos, V., Nalbantoglu, T., Vitorica-Yrezabal, I. J., Yasar, F. T., and Zhu, X. (2017). Pyridyl-acyl Hydrazone Rotaxanes and Molecular Shuttles. J. Am. Chem. Soc. 139, 7104–7109. doi:10.1021/jacs.7b03307
Li, H., Fahrenbach, A. C., Coskun, A., Zhu, Z., Barin, G., Zhao, Y.-L., et al. (2011). A Light-Stimulated Molecular Switch Driven by Radical-Radical Interactions in Water. Angew. Chem. Int. Ed. 50, 6782–6788. doi:10.1002/anie.201102510
Li, S., Taura, D., Hashidzume, A., and Harada, A. (2010). Light-Switchable Janus [2]Rotaxanes Based on α-Cyclodextrin Derivatives Bearing Two Recognition Sites Linked with Oligo(ethylene Glycol). Chem. Asian J. 5, 2281–2289. doi:10.1002/asia.201000169
Li, W.-X., Yin, Y.-F., Duan, H.-Y., Liu, L.-J., Kong, L.-C., Zhan, T.-G., et al. (2021). An Orthogonal Photoresponsive Tristable [3]rotaxane with Non-destructive Readout. Org. Chem. Front. 8, 1482–1489. doi:10.1039/d0qo01441a
Ma, X., and Tian, H. (2010). Bright Functional Rotaxanes. Chem. Soc. Rev. 39, 70–80. doi:10.1039/b901710k
Ma, X., Wang, Q. C., and Tian, H. (2009). Photo-Driven Molecular Shuttles. Prog. Chem. 21, 106–115. Article number: 1005-281X(2009)01-0106-10.
Martinez-Cuezva, A., Saura-Sanmartin, A., Nicolas-Garcia, T., Navarro, C., Orenes, R.-A., Alajarin, M., et al. (2017). Photoswitchable Interlocked Thiodiglycolamide as a Cocatalyst of a Chalcogeno-Baylis-Hillman Reaction. Chem. Sci. 8, 3775–3780. doi:10.1039/c7sc00724h
Murakami, H., Kawabuchi, A., Matsumoto, R., Ido, T., and Nakashima, N. (2005). A Multi-Mode-Driven Molecular Shuttle: Photochemically and Thermally Reactive Azobenzene Rotaxanes. J. Am. Chem. Soc. 127, 15891–15899. doi:10.1021/ja053690l
Ogoshi, T., Kotera, D., Fa, S., Nishida, S., Kakuta, T., Yamagishi, T.-a., et al. (2020). A Light-Operated Pillar[6]arene-Based Molecular Shuttle. Chem. Commun. 56, 10871–10874. doi:10.1039/d0cc03945d
Panman, M. R., Bakker, B. H., den Uyl, D., Kay, E. R., Leigh, D. A., Buma, W. J., et al. (2013). Water Lubricates Hydrogen-Bonded Molecular Machines. Nat. Chem 5, 929–934. doi:10.1038/nchem.1744
Panman, M. R., Bodis, P., Shaw, D. J., Bakker, B. H., Newton, A. C., Kay, E. R., et al. (2010). Operation Mechanism of a Molecular Machine Revealed Using Time-Resolved Vibrational Spectroscopy. Science 328, 1255–1258. doi:10.1126/science.1187967
Panman, M. R., Bodis, P., Shaw, D. J., Bakker, B. H., Newton, A. C., Kay, E. R., et al. (2012). Time-resolved Vibrational Spectroscopy of a Molecular Shuttle. Phys. Chem. Chem. Phys. 14, 1865–1875. doi:10.1039/c1cp22146a
Qu, D.-H., Wang, Q.-C., and Tian, H. (2005). A Half Adder Based on a Photochemically Driven [2]rotaxane. Angew. Chem. Int. Ed. 44, 5296–5299. doi:10.1002/anie.200501215
Qu, D.-H., Wang, Q.-C., Zhang, Q.-W., Ma, X., and Tian, H. (2015). Photoresponsive Host-Guest Functional Systems. Chem. Rev. 115, 7543–7588. doi:10.1021/cr5006342
Raiteri, P., Bussi, G., Cucinotta, C. S., Credi, A., Stoddart, J. F., and Parrinello, M. (2008). Unravelling the Shuttling Mechanism in a Photoswitchable Multicomponent Bistable Rotaxane. Angew. Chem. Int. Ed. 47, 3536–3539. doi:10.1002/anie.200705207
Shi, Q., Meng, Z., Xiang, J.-F., and Chen, C.-F. (2018). Efficient Control of Movement in Non-photoresponsive Molecular Machines by a Photo-Induced Proton-Transfer Strategy. Chem. Commun. 54, 3536–3539. doi:10.1039/C8CC01570H
Silvi, S., Venturi, M., and Credi, A. (2009). Artificial Molecular Shuttles: from Concepts to Devices. J. Mater. Chem. 19, 2279–2294. doi:10.1039/b818609j
Silvi, S., Venturi, M., and Credi, A. (2011). Light Operated Molecular Machines. Chem. Commun. 47, 2483–2489. doi:10.1039/c0cc03829f
Stoddart, J. F. (2017). Mechanically Interlocked Molecules (MIMs)-Molecular Shuttles, Switches, and Machines (Nobel Lecture). Angew. Chem. Int. Ed. 56, 11094–11125. doi:10.1002/anie.201703216
Sun, S., Lu, D., Huang, Q., Liu, Q., Yao, Y., and Shi, Y. (2019). Reversible Surface Activity and Self-Assembly Behavior and Transformation of Amphiphilic Ionic Liquids in Water Induced by a Pillar[5]arene-Based Host-Guest Interaction. J. Colloid Interf. Sci. 533, 42–46. doi:10.1016/j.jcis.2018.08.051
Tron, A., Pianet, I., Martinez-Cuezva, A., Tucker, J. H. R., Pisciottani, L., Alajarin, M., et al. (2017). Remote Photoregulated Ring Gliding in a [2]rotaxane via a Molecular Effector. Org. Lett. 19, 154–157. doi:10.1021/acs.orglett.6b03457
Wang, C., Wang, S., Yang, H., Xiang, Y., Wang, X., Bao, C., et al. (2021). A Light‐Operated Molecular Cable Car for Gated Ion Transport. Angew. Chem. Int. Ed. 60, 14836–14840. doi:10.1002/anie.202102838
Wang, L., and Li, Q. (2018a). Photochromism into Nanosystems: towards Lighting up the Future Nanoworld. Chem. Soc. Rev. 47, 1044–1097. doi:10.1039/c7cs00630f
Wang, Q., Chen, D., and Tian, H. (2018b). Artificial Molecular Machines that Can Perform Work. Sci. China Chem. 61, 1261–1273. doi:10.1007/s11426-018-9267-3
Wang, Y., Tian, Y., Chen, Y.-Z., Niu, L.-Y., Wu, L.-Z., Tung, C.-H., et al. (2018c). A Light-Driven Molecular Machine Based on Stiff Stilbene. Chem. Commun. 54, 7991–7994. doi:10.1039/c8cc04542a
Wu, Y., Frasconi, M., Liu, W.-G., Young, R. M., Goddard, W. A., Wasielewski, M. R., et al. (2020). Electrochemical Switching of a Fluorescent Molecular Rotor Embedded within a Bistable Rotaxane. J. Am. Chem. Soc. 142, 11835–11846. doi:10.1021/jacs.0c03701
Xue, M., Yang, Y., Chi, X., Yan, X., and Huang, F. (2015). Development of Pseudorotaxanes and Rotaxanes: from Synthesis to Stimuli-Responsive Motions to Applications. Chem. Rev. 115, 7398–7501. doi:10.1021/cr5005869
Yan, H., Teh, C., Sreejith, S., Zhu, L., Kwok, A., Fang, W., et al. (2012). Functional Mesoporous Silica Nanoparticles for Photothermal-Controlled Drug Delivery In Vivo. Angew. Chem. Int. Ed. 51, 8373–8377. doi:10.1002/anie.201203993
Yang, J.-X., Li, Z., Gu, X.-H., Zhan, T.-G., Cui, J., and Zhang, K.-D. (2021). A Photogated Photoswitchable [2]rotaxane Based on Orthogonal Photoreactions. Tetrahedron 92, 132284. doi:10.1016/j.tet.2021.132284
Yang, L.-P., Jia, F., Cui, J.-S., Lu, S.-B., and Jiang, W. (2017). Light-controlled Switching of a Non-photoresponsive Molecular Shuttle. Org. Lett. 19, 2945–2948. doi:10.1021/acs.orglett.7b01184
Young, P. G., Hirose, K., and Tobe, Y. (2014). Axle Length Does Not Affect Switching Dynamics in Degenerate Molecular Shuttles with Rigid Spacers. J. Am. Chem. Soc. 136, 7899–7906. doi:10.1021/ja412671k
Yu, J.-J., Zhao, L.-Y., Shi, Z.-T., Zhang, Q., London, G., Liang, W.-J., et al. (2019a). Pumping a Ring-Sliding Molecular Motion by a Light-Powered Molecular Motor. J. Org. Chem. 84, 5790–5802. doi:10.1021/acs.joc.9b00783
Yu, S., McClenaghan, N. D., and Pozzo, J.-L. (2019b). Photochromic Rotaxanes and Pseudorotaxanes. Photochem. Photobiol. Sci. 18, 2102–2111. doi:10.1039/c9pp00057g
Zhan, T.-G., Yin, H.-H., Zheng, S.-T., Lin, W.-C., Shen, N.-L., Cui, J., et al. (2018). Toward Bidirectional Photoswitchable Colored Photochromic Molecules with Visible Light Stability. Chem. Commun. 54, 9356–9359. doi:10.1039/c8cc05294h
Zhan, T.-G., Yun, M.-Y., Lin, J.-L., Yu, X.-Y., and Zhang, K.-D. (2016). Dual Absorption Spectral Changes by Light-Triggered Shuttling in Bistable [2]rotaxanes with Non-destructive Readout. Chem. Commun. 52, 14085–14088. doi:10.1039/c6cc08314e
Zhang, T., Mu, L., She, G., and Shi, W. (2012). Light-driven Molecular Shuttles Modified on Silicon Nanowires. Chem. Commun. 48, 452–454. doi:10.1039/c1cc16339f
Keywords: molecular shuttles, photoisomerization, photochemical reaction, photoinduced electron transfer, catalysis, drug delivery, ion transport
Citation: Yao B, Sun H, Yang L, Wang S and Liu X (2022) Recent Progress in Light-Driven Molecular Shuttles. Front. Chem. 9:832735. doi: 10.3389/fchem.2021.832735
Received: 10 December 2021; Accepted: 23 December 2021;
Published: 02 February 2022.
Edited by:
Wei Wang, East China Normal University, ChinaReviewed by:
Yong Yao, Nantong University, ChinaYing Han, Yangzhou University, ChinaCopyright © 2022 Yao, Sun, Yang, Wang and Liu. This is an open-access article distributed under the terms of the Creative Commons Attribution License (CC BY). The use, distribution or reproduction in other forums is permitted, provided the original author(s) and the copyright owner(s) are credited and that the original publication in this journal is cited, in accordance with accepted academic practice. No use, distribution or reproduction is permitted which does not comply with these terms.
*Correspondence: Bin Yao, eWFvYmlucGt1QDEyNi5jb20=
Disclaimer: All claims expressed in this article are solely those of the authors and do not necessarily represent those of their affiliated organizations, or those of the publisher, the editors and the reviewers. Any product that may be evaluated in this article or claim that may be made by its manufacturer is not guaranteed or endorsed by the publisher.
Research integrity at Frontiers
Learn more about the work of our research integrity team to safeguard the quality of each article we publish.