Corrigendum: High-Sensitive Surface Plasmon Resonance Imaging Biosensor Based on Dual-Wavelength Differential Method
- 1Key Laboratory of Optoelectronic Devices and Systems of Ministry of Education and Guangdong Province, College of Physics and Optoelectronics Engineering, Shenzhen University, Shenzhen, China
- 2Department of Biomedical Engineering, The Chinese University of Hong Kong, Shatin, Hong Kong SAR, China
- 3Aston Institute of Photonic Technologies, Aston University, Birmingham, United Kingdom
- 4Department of Bioengineering and COMSET, Clemson University, Clemson, SC, United States
Intensity interrogation surface plasmon resonance (ISPR) sensing has a simple schematic design and is the most widely used surface plasmon resonance technology at present. However, it has relatively low sensitivity, especially for ISPR imaging (ISPRi). In this paper, a new technique for the real-time monitoring of biomolecule binding on sensor surfaces via ISPRi detection is described. The technique is based on the interrogation of the differential value of two intensities at two specific wavelengths from the reflected light spectrum. In addition, we also optimized the selection of dual-wavelength parameters under different circumstances to achieve the highest sensitivity. The new technique achieved a refractive index resolution (RIR) of 2.24 × 10–6 RIU, which is far beyond that of traditional ISPRi technique. Moreover, our new ISPRi technique also realized the real-time detection of high-throughput biomolecular binding. This study is expected to promote the development of faster and more accurate SPRi technologies.
Introduction
Surface plasmon resonance (SPR) has become a powerful tool for exploring biomolecular interactions in the last decades (Homola, 2008; Schasfoort et al., 2018; Bockova et al., 2019). Four practical interrogation SPR sensing techniques, namely, intensity, wavelength, angle, and phase interrogation SPR modes, have been widely reported (Homola et al., 1999; Liu et al., 2005; Huang et al., 2012). In the wavelength interrogation SPR mode, the incident angle is fixed, and the SPR spectral profile and resonance wavelength can be obtained by scanning the incidence wavelength or using a spectrometer for analyzing the reflected beam. Angular interrogation SPR usually utilizes monochromatic light as excitation light, and the shift of the SPR dip can be monitored through the continuous scanning of the SPR angular spectrograph. In the phase interrogation SPR mode, the SPR phase shift can be obtained by detecting the phase difference between the signal beam and reference beam.
In intensity interrogation SPR (ISPR) mode, the incidence wavelength and angle are usually fixed, and the reflected light intensity is directly monitored to translate the refractive index variation of the sample. This technology avoids the usage of scanning or modulator devices, such as wavelength scanning devices, angle scanning devices, and phase modulators. Compared with the other three interrogation modes, ISPR mode has a simple structure design, easy operational procedure, and cost-effectiveness (Zeng et al., 2017). Moreover, the detecting speed of the ISPR system solely depends on the speed of the light intensity detector, therefore, it has great application potential in 2D real-time biochemical reaction monitoring. In addition, in terms of producibility, ISPR technology, such as Biacore from GE Healthcare and SPR chips from GWC Technologies, is also the most commonly used method in commercial SPR instruments (Nilvebrant, 2018). These instruments use ISPR for DNA hybridization analysis (Mudgal et al., 2020), protein interaction analysis (Nilvebrant, 2018), and cellular analysis (Zhou X. L. et al., 2020).
In addition, sensitivity is a vital parameter in biochemical detection, Researchers have developed many techniques to increase the sensitivity for detecting biomolecular interactions at lower concentrations with lesser sample consumption. And for a typical SPR sensor, a smaller refractive index resolution (RIR) gives a higher system sensitivity. The RIR of a typical ISPR at present is
Principle of the Dual-Wavelength Differential Method
For a Kretschmann-based SPR scheme with gold film (48 nm thickness) on the prism made by SF11, the change in refractive index is from 1.333 RIU to 1.333019 RIU in
As it’s illustrated in Figure 1, we simulated the SPR spectral curve under different incident wavelengths. The SPR spectral curve shows two linear regions, in which the reflected light intensity varies linearly with the refractive index. When the refractive index increases, the reflected light intensity increases in one linear region (blue arrow) and decreases in another linear region (red arrow). We can monitor the intensities in the two linear regions at the same time, and make a subtraction
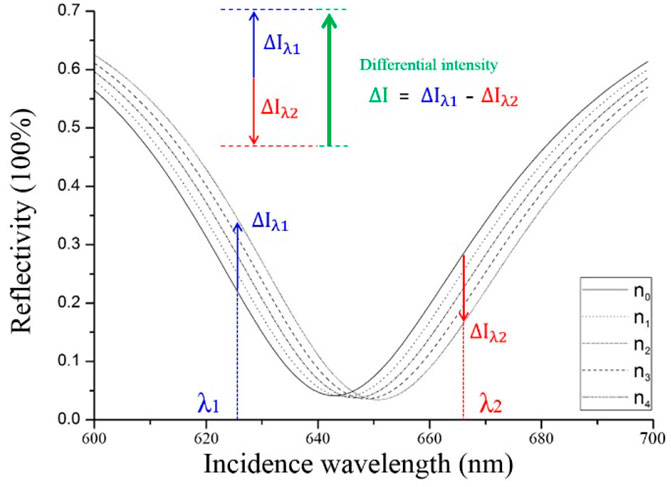
FIGURE 1. Simulated SPR spectral curves of different sample refractive indexes.
In addition, dual-wavelength selection needs to be optimized to obtain the highest sensitivity amplification. Based on the simulation results of intensity difference
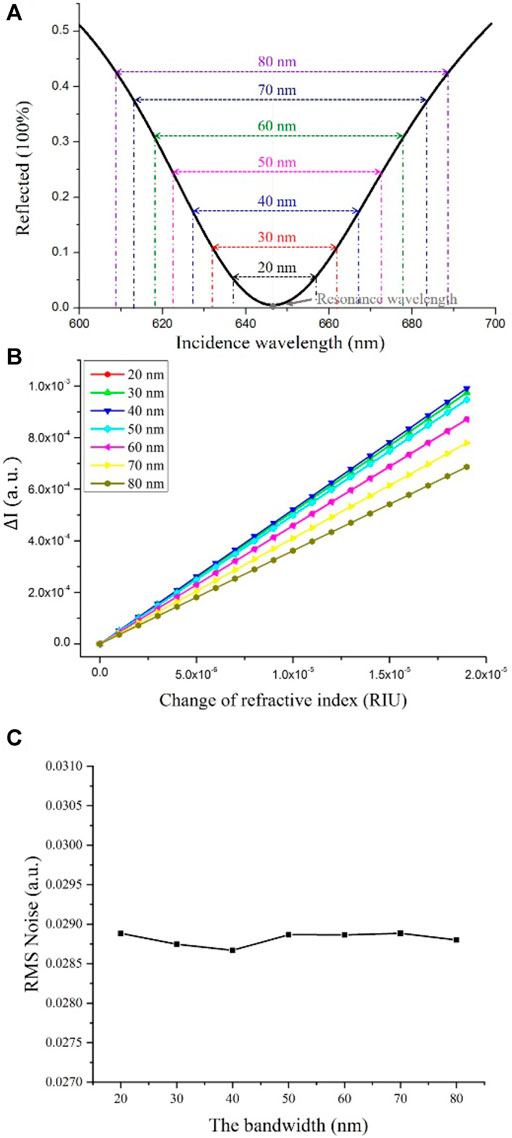
FIGURE 2. Simulation of intensity amplification optimization and influence of light source noise study. (A) Schematic diagram of dual-wavelength selection; (B) Dual-wavelength differential value
Setup
Figure 3 shows the optical path of the system. A 100 W halogen lamp (GCI-060101, Daheng Optics, Beijing, China) was used as the excitation light source. Divergent light from multimode fiber passes through the collimating lens groups of L1 and L2, and diaphragm aperture to produce parallel lights, then enters into the sensing module. And the sensing module includes prism, sensor chip, and flow cell. The parallel light is coupled into the prism to excite the SPR effect on the sensor chip in the gold film.
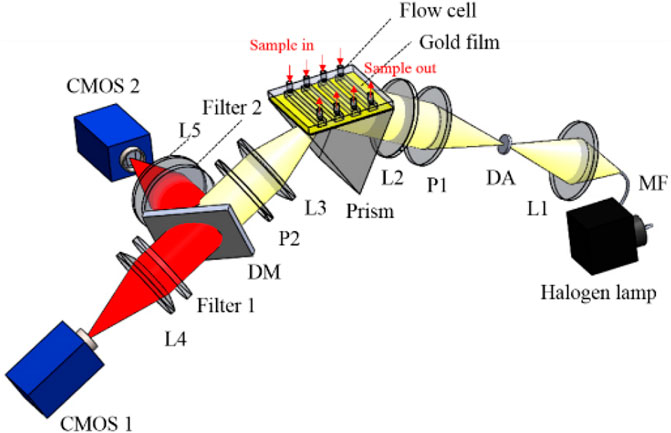
FIGURE 3. Schematic of ISPRi system based on Kreschmann configuration. L1–L5, lenses; DA, diaphragm aperture; MF, multimode fiber; DM, dichroic mirror; P1 and P2, polarizers.
After the reflected light passes through L3 and P2, it is split by a 635 nm low-pass dichroic mirror (FF635-Di01-
Results and Discussions
We detected the intensity shift induced by small variations in saline water concentration to determine the refractive index resolution of our system. We tested a series of saline water with concentrations of 0–2% in increments of 0.5% by volume, which correspond to the refractive indexes ranging from 1.3330 RIU to 1.3367 RIU. During the test, the incident angle was fixed to 54.9°, which corresponds to the resonance wavelength of 630 nm and sample’s refractive index of 1.3330 RIU. Before the test, the system obtained the image of the whole sensor surface, and we evenly divided the entire sensing surface into 3 × 3 array sensor sites. And the nine sites were monitored simultaneously. The results are illustrated in Figure 4A.
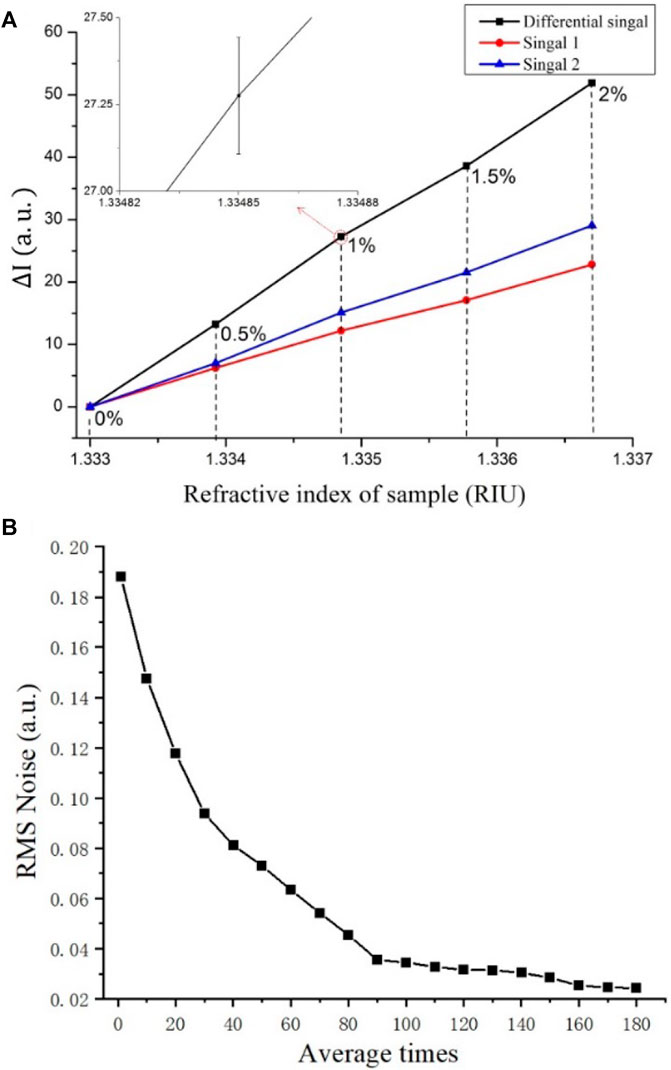
FIGURE 4. Sensitivity test results. (A) Resonance wavelength shift obtained from low-concentration saline water; (B) Relation between averaging number and RMS noise.
We also detected the noise of our ISPRi system. Noise is reduced by averaging multiple acquisitions. The relationship between average times and RMS noise is shown in Figure 4B. RMS noise was reduced with the increase in averaging number and tended to be stable and small when the averaging number exceeded 100 times. In our experiments, averaging number was set to 100 to ensure best performance. The frame rates of the two CMOSs were 100 fps, so the imaging time for acquiring one SPR image is 1 s. Therefore, the real-time refractive index monitoring ability of our SPR system is verified.
And the refractive index resolution (RIR) of the system can be calculated from Eq. 2 (Zeng et al., 2020a; Ng et al., 2013)
where
In addition, we also tested the biosensing capability of our ISPRi sensor in detecting the interactions between two kinds of protein molecules. The biomolecule binding events on the sensing surface is indicated by the change in refractive light intensity (Mudgal et al., 2020). The sensor chip was rinsed with deionized water and dried with nitrogen before being placed on the prism for the biological experiment. The chip was attached to the coupling prism using a small drop of refractive index matching oil. And a four-channel PDMS flow chamber was adopted for sample injection.
For the pretreatment of the sensing surface, the antigen of human IgG was bound to the gold film via physical absorption process and real-time monitoring was performed (Zeng et al., 2020b; Miyan et al., 2021; Zhou et al., 2022). First, phosphate-buffered saline (PBS, 0.01 M, pH 7.4) was separately injected into the four channels and flushed for 5 min. Second, human IgG (100 μg/ml) was injected into each channel simultaneously in a flow rate of 10 μL/min until the image intensities were stabilized. Then, PBS was injected and flushing. After the images are stable, bovine serum albumin solution in the concentration of 10 mg/ml was injected into the four channels for 5 min to occupy the possible vacancy sites without the binding of human IgG. Therefore, the nonspecific binding of goat anti-human IgG to the surface in the following procedure can be prevented. Then, the PBS solution are injected to each channel for 10 min so as to obtain the baseline for the following bio-experiments.
In the biological binding process. The four channels were injected with PBS buffer and goat anti-human IgG in concentrations of 1, 2, and 5 μg/ml respectively. The channel with PBS was used as the reference channel, and the three other channels were the reaction channels. The differential intensities of all channels were recorded. After the intensity became stationary, PBS was injected to wash the nonspecific binding of goat anti-human IgG to the sensing surface.
The real-time monitoring reflected the light intensities of the four channels as shown in Figure 5. The black curve is the reference channel, and the curves with different colors are the reaction channels. The
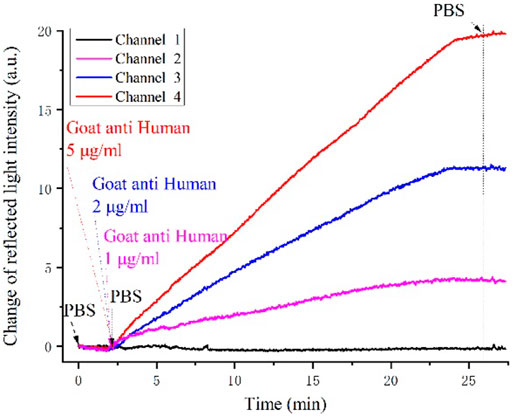
FIGURE 5. Real-time intensity variation in the antigen-antibody interaction between goat anti-human IgG and human IgG.
Another unique advantage of our system is its SPR imaging ability. The SPR image of the entire sensing surface can be obtained simultaneously during the detection. As illustrated in Figure 6, the color of each pixel represents the intensity shift, which indicates the biomolecule binding situation on the corresponding sensing site after the reaction. The light intensity is shown in the false color map. Figures 6A,B are the images of the single-wavelength intensity shifts of
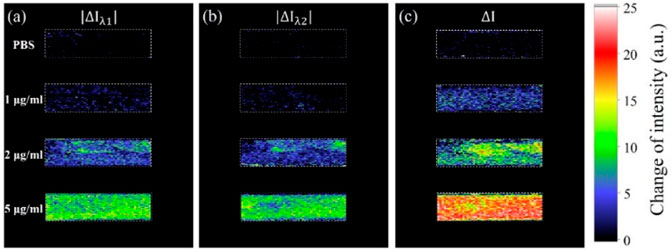
FIGURE 6. SPR Intensity changes of the four channels treated with PBS and 1, 2, and 5 μg/ml human IgG. (A,B) are the images of the intensity based on single wavelength of
Conclusion
In summary, we developed a versatile real-time ISPRi system, which has improved sensitivity based on the differential light intensities from two selected wavelengths. This technique has several advantages compared with traditional ISPR sensors. The proposed technique achieved a higher sensitivity and higher detection throughput in a low-cost optical configuration without using any mechanical moving parts or optical scanning devices compared with other sensors. The results have shown that the SPR refractive index resolution of the scheme is
Data Availability Statement
The raw data supporting the conclusions of this article will be made available by the authors, without undue reservation.
Author Contributions
YZ carried out the experimental work and JC carried out the simulation part of the project. JZ, WS, WK, JQ, H-HP, KZ and BG provide experimental help to YZ. YZ and JC finished writing the manuscript, JC and YS revised it.
Funding
This work was supported by projects from the National Natural Science Foundation of China (Grant Nos. 61775148 and 61905145), Guangdong Natural Science Foundation and Province Project (Grant No. 2021A1515011916), and Shenzhen Science and Technology R and D and Innovation Foundation (Grant Nos. JCYJ20200109105608771 and JCYJ20180305124754860).
Conflict of Interest
The authors declare that the research was conducted in the absence of any commercial or financial relationships that could be construed as a potential conflict of interest.
Publisher’s Note
All claims expressed in this article are solely those of the authors and do not necessarily represent those of their affiliated organizations, or those of the publisher, the editors and the reviewers. Any product that may be evaluated in this article, or claim that may be made by its manufacturer, is not guaranteed or endorsed by the publisher.
Acknowledgments
We would like to thank the rest of the team for the fruitful discussion of this project.
References
Bocková, M., Slabý, J., Špringer, T., and Homola, J. (2019). Advances in Surface Plasmon Resonance Imaging and Microscopy and Their Biological Applications. Annu. Rev. Anal. Chem. 12, 151–176. doi:10.1146/annurev-anchem-061318-115106
Homola, J., Yee, S. S., and Gauglitz, G. (1999). Surface Plasmon Resonance Sensors Review. Sens. Actuators B: Chem. 54 (1-2), 3–15. doi:10.1016/S0925-4005(98)00321-9
Homola, J. (2008). Surface Plasmon Resonance Sensors for Detection of Chemical and Biological Species. Chem. Rev. 108, 462–493. doi:10.1021/cr068107d
Huang, Y. H., Ho, H. P., Wu, S. Y., and Kong, S. K. (2012). Detecting Phase Shifts in Surface Plasmon Resonance: A Review. Adv. Opt. Tech. 2012, 1–12. doi:10.1155/2012/471957
Liu, X., Song, D., Zhang, Q., Tian, Y., Ding, L., and Zhang, H. (2005). Wavelength-modulation Surface Plasmon Resonance Sensor. Trends Anal. Chem. 24, 887–893. doi:10.1016/j.trac.2005.05.010
Miyan, R., Wang, X., Zhou, J., Zeng, Y., Qu, J., Ho, H.-P., et al. (2021). Phase Interrogation Surface Plasmon Resonance Hyperspectral Imaging Sensor for Multi-Channel High-Throughput Detection. Opt. Express 29 (20), 31418–31425. doi:10.1364/OE.433052
Mudgal, N., Saharia, A., Choure, K. K., Agarwal, A., and Singh, G. (2020). Sensitivity Enhancement with Anti-reflection Coating of Silicon Nitride (Si3N4) Layer in Silver-Based Surface Plasmon Resonance (SPR) Sensor for Sensing of DNA Hybridization. Appl. Phys. A. 126, 126. doi:10.1007/s00339-020-04126-9
Ng, S. P., Loo, F. C., Wu, S. Y., Kong, S. K., Wu, C. M. L., and Ho, H. P. (2013). Common-path Spectral Interferometry with Temporal Carrier for Highly Sensitive Surface Plasmon Resonance Sensing. Opt. Express 21 (17), 20268–20273. doi:10.1364/OE.21.020268
Nilvebrant, J. (2018). Kinetic Analysis and Epitope Binning Using Surface Plasmon Resonance. Meth. Mol. Biol. 1785, 187–205. doi:10.1007/978-1-4939-7841-0_12
Puiu, M., and Bala, C. (2016). SPR and SPR Imaging: Recent Trends in Developing Nanodevices for Detection and Real-Time Monitoring of Biomolecular Events. Sensors 16 (6), 870. doi:10.3390/s16060870
Schasfoort, R., Abali, F., Stojanovic, I., Vidarsson, G., and Terstappen, L. (2018). Trends in SPR Cytometry: Advances in Label-free Detection of Cell Parameters. Biosensors 8, 102. doi:10.3390/bios8040102
Wong, C. L., and Olivo, M. (2014). Surface Plasmon Resonance Imaging Sensors: A Review. Plasmonics 9, 809–824. doi:10.1007/s11468-013-9662-3
Yuk, J. S., Jung, J.-W., Jung, S.-H., Han, J.-A., Kim, Y.-M., and Ha, K.-S. (2005). Sensitivity of Ex Situ and In Situ Spectral Surface Plasmon Resonance Sensors in the Analysis of Protein Arrays. Biosens. Bioelectron. 20, 2189–2196. doi:10.1016/j.bios.2004.07.034
Zeng, Y., Hu, R., Wang, L., Gu, D., He, J., Wu, S.-Y., et al. (2017). Recent Advances in Surface Plasmon Resonance Imaging: Detection Speed, Sensitivity, and Portability. Nanophotonics 6, 1017–1030. doi:10.1515/nanoph-2017-0022
Zeng, Y., Zhou, J., Wang, X., Cai, Z., and Shao, Y. (2019). Wavelength-scanning Surface Plasmon Resonance Microscopy: A Novel Tool for Real Time Sensing of Cell-Substrate Interactions. Biosens. Bioelectron. 145, 111717. doi:10.1016/j.bios.2019.111717
Zeng, Y., Wang, X., Zhou, J., Miyan, R., Qu, J., Ho, H.-P., et al. (2020a). Phase Interrogation SPR Sensing Based on white Light Polarized Interference for Wide Dynamic Detection Range. Opt. Express 28, 3442–3450. doi:10.1364/OE.382242
Zeng, Y., Wang, X., Zhou, J., Miyan, R., Qu, J., Ho, H.-P., et al. (2020b). High-throughput Imaging Surface Plasmon Resonance Biosensing Based on Ultrafast Two-point Spectral-Dip Tracking Scheme. Opt. Express 28 (14), 20624–20633. doi:10.1364/oe.396656
Zhou, J., Zeng, Y., Wang, X., Wu, C., Cai, Z., Gao, B. Z., et al. (2020a). The Capture of Antibodies by Antibody-Binding Proteins for ABO Blood Typing Using SPR Imaging-Based Sensing Technology. Sensors Actuators B: Chem. 304, 127391. doi:10.1016/j.snb.2019.127391
Zhou, X. L., Yang, Y., Wang, S., and Liu, X. W. (2020b). Surface Plasmon Resonance Microscopy: From Single‐Molecule Sensing to Single‐Cell Imaging. Angew. Chem. Int. Ed. 59, 1776–1785. doi:10.1002/anie.201908806
Zhou, J., Wang, X., Chen, J. Y., Zeng, Y., Gu, D., Gao, B. Z., et al. (2022). Polymeric Microsphere Enhanced Surface Plasmon Resonance Imaging Immunosensor for Occult Blood Monitoring. Sensors Actuators B: Chem. 350, 130858. doi:10.1016/j.snb.2021.130858
Zybin, A., Grunwald., C., Mirsky., V. M., Kuhlmann, J., Wolfbeis, O. S., and Niemax, K. (2005). Double-Wavelength Technique for Surface Plasmon Resonance Measurements: Basic Concept and Applications for Single Sensors and Two-Dimensional Sensor Arrays. Anal. Chem. 77 (8), 2393–2399. doi:10.1021/ac048156v
Keywords: surface plasmon, biosensing and bioimaging, surface plasmon sensors, biophotonics and plasmonics, biomolecule interaction
Citation: Zeng Y, Zhou J, Sang W, Kong W, Qu J, Ho H-P, Zhou K, Gao BZ, Chen J and Shao Y (2021) High-Sensitive Surface Plasmon Resonance Imaging Biosensor Based on Dual-Wavelength Differential Method. Front. Chem. 9:801355. doi: 10.3389/fchem.2021.801355
Received: 25 October 2021; Accepted: 15 November 2021;
Published: 08 December 2021.
Edited by:
Chao He, University of Oxford, United KingdomReviewed by:
Le Liu, Tsinghua University, ChinaJianan He, Shenzhen Academy of Inspection and Quarantine, China
Copyright © 2021 Zeng, Zhou, Sang, Kong, Qu, Ho, Zhou, Gao, Chen and Shao. This is an open-access article distributed under the terms of the Creative Commons Attribution License (CC BY). The use, distribution or reproduction in other forums is permitted, provided the original author(s) and the copyright owner(s) are credited and that the original publication in this journal is cited, in accordance with accepted academic practice. No use, distribution or reproduction is permitted which does not comply with these terms.
*Correspondence: Jiajie Chen, cjj@szu.edu.cn; Yonghong Shao, shaoyh@szu.edu.cn