- Max Planck Institute for Polymer Research, Mainz, Germany
Thermally-activated delayed fluorescence (TADF) is a concept which helps to harvest triplet excitations, boosting the efficiency of an organic light-emitting diode. TADF can be observed in molecules with spatially separated donor and acceptor groups with a reduced triplet-singlet energy level splitting. TADF materials with balanced electron and hole transport are attractive for realizing efficient single-layer organic light emitting diodes, greatly simplifying their manufacturing and improving their stability. Our goal here is to computationally screen such materials and provide a comprehensive database of compounds with a range of emission wavelengths, ionization energies, and electron affinities.
Introduction
For obtaining efficient organic light-emitting diodes (OLEDs), it is convenient to tune individual processes, such as charge injection, balanced hole and electron transport, and triplet and singlet exciton harvesting, by using dedicated layers. Every new material adds a degree of freedom and hence flexibility to the OLED design. For instance, doped charge transport layers ensure Ohmic injection, an appropriate host material balances transport inside the emitting layer, and the phosphorescent emitter ensures triplet harvesting. However, every new emitter requires optimization of the surrounding layers, with respect to energy levels, triplet energies, and charge-transport properties, complicating the OLED design.
Recently, it was demonstrated that a complex multilayer design can be substituted by a simple single-layer architecture (Kotadiya et al., 2019a) without compromising the balanced and trap-free electron and hole transport. The ohmic charge injection and the absence of heterojunctions resulted in extremely low operating voltages and thus power efficiency in a single-layer OLED utilizing thermally activated delayed fluorescence, which helps to convert triplet into singlet excitons (Uoyama et al., 2012; Godumala et al., 2019). An external quantum efficiency of 19% was achieved. Owing to the broad recombination zone and low operating voltages, one of the key features of the single-layer device is the improved device stability, which can be used to design a stable blue OLED, a grand challenge in OLED research (Heimel et al., 2018; Paterson et al., 2019, 2020). In view of this, it would be useful to understand if the single-layer design can be employed for blue OLEDs: the issue here is the trap-free transport for both holes and electrons, which sets limits on the transport gap. In this paper, we first formulate the chemical design rules for TADF emitters with ambipolar transport. Using these rules, we then computationally pre-screen a set of molecules comprised of acceptor, donor, and bridge blocks and grade them according to the predicted emission wavelength.
Design Criteria
Singlet-Triplet Energy Splitting
The important task of a TADF emitter is to convert triplet into singlet excitations. To do this, the reverse intersystem crossing rate,
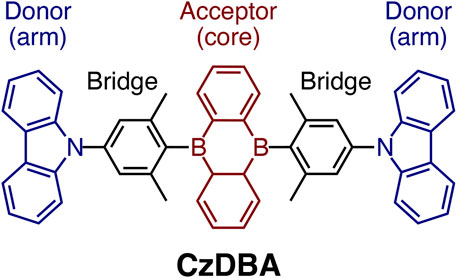
FIGURE 1. The molecular structure of a prototypical single-layer TADF emitter, 5,10-bis(4-(9H-carbazol-9-yl)-2,6-dimethylphenyl)-5,10-dihydroboran-threne (CzDBA). It features a D-
Ambipolar Trap-free Transport
To ensure a broad recombination zone within the emission layer, the thin film of the TADF emitter should provide balanced and trap-free transport of holes and electrons. To realize this, one needs to select compounds with an ionization energy (IE) and electron affinity (EA) lying within the trap-free energy window (Kotadiya et al., 2019b), i.e., with ionization energy (IE) < 6.5 eV and electron affinity (EA) > 2.5 eV. These criteria ensure that contaminants such as oxygen or water do not serve as energetic traps for holes and electrons.
Small Energetic Disorder
From a dipolar glass model, the energetic disorder present in a disordered molecular solid is proportional to the dipole moment of the composing molecule. Therefore, thin organic films with molecules with a small dipole moment (D) normally have a narrower density of states (Novikov and Vannikov, 2009; Lin et al., 2019; Mondal et al., 2021; Stankevych et al., 2021). This design criteria can be enforced by selecting centrosymmetric molecules only of the D-
Building Blocks
With these design rules in mind, and in view of the successful example of CzDBA, we build and characterize a database of emitters that fulfill the aforementioned criteria. To construct the emitters, we start with 97 potential donor and acceptor building blocks, all shown in the Supplementary Note S1. All of them are (quasi-)linear, composed of three (fused) rings and are reported in literature (synthesizable). These building blocks are further pre-screened to ensure the desired donor-acceptor architecture in an emitter. The pre-screening proceeds as follows: knowing that the IE and EA of CzDBA is already quite close to the boundary of the trap-free window (Kotadiya et al., 2019a; Liu et al., 2021) we take the IECz and EADBA as the pre-screening criteria for donors and acceptors, respectively. Only the fragments possessing IE < IECz + 0.2 eV (EA > EADBA - 0.2 eV) will be chosen as “trap-free” donors (acceptors) and enter the next round, see Supplementary Figure S2. The molecular structures of donors and acceptors that pass the prescreening step are summarized in Figure 2. To build the emitter molecules, only the building blocks with the inversion symmetry are used as core fragments. These are shown in dark colors in Figure 2. This choice helps to fulfill the centrosymmetric requirement for the entire molecule.
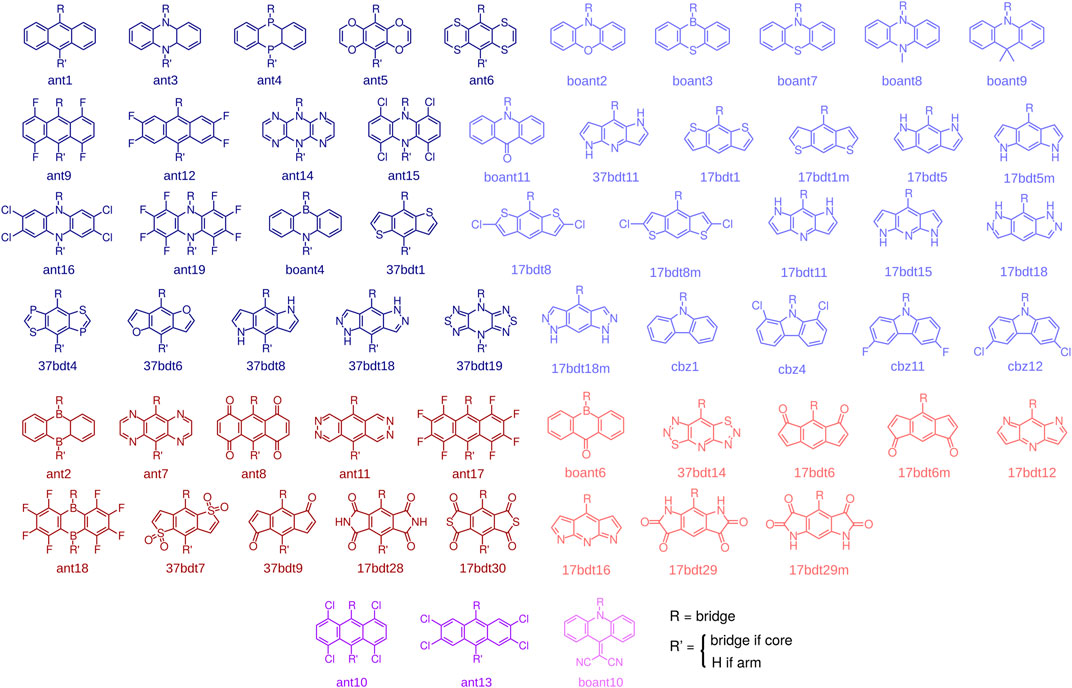
FIGURE 2. Donors (blue), acceptors (red), and building blocks that can serve both as donors and acceptors (purple). The blocks with inversion symmetry (dark colors) can be used as either core or arm fragments. The building blocks without inversion symmetry (light colors) can only be used as arm fragments. We also included boant4, which is not centrosymmetric, as a core fragment to increase the number of compounds in the database.
Computational Workflow
Using the selected building blocks, we constructed the database of D-
To obtain reliable predictions of solid-state IE, EA and excited-state energy, we followed the cost-effective
For compounds that pass the screening criteria, the density-of-states distributions for holes and electrons were computed via multi-scale simulations, that include morphology generation using molecular-dynamics simulations, followed by polarizable force-field evaluation of the solid-state contributions to the gas-phase energy levels (Rühle et al., 2011; Poelking and Andrienko, 2016; Andrienko, 2018; Mondal et al., 2021). The entire workflow is illustrated in Figure 3.
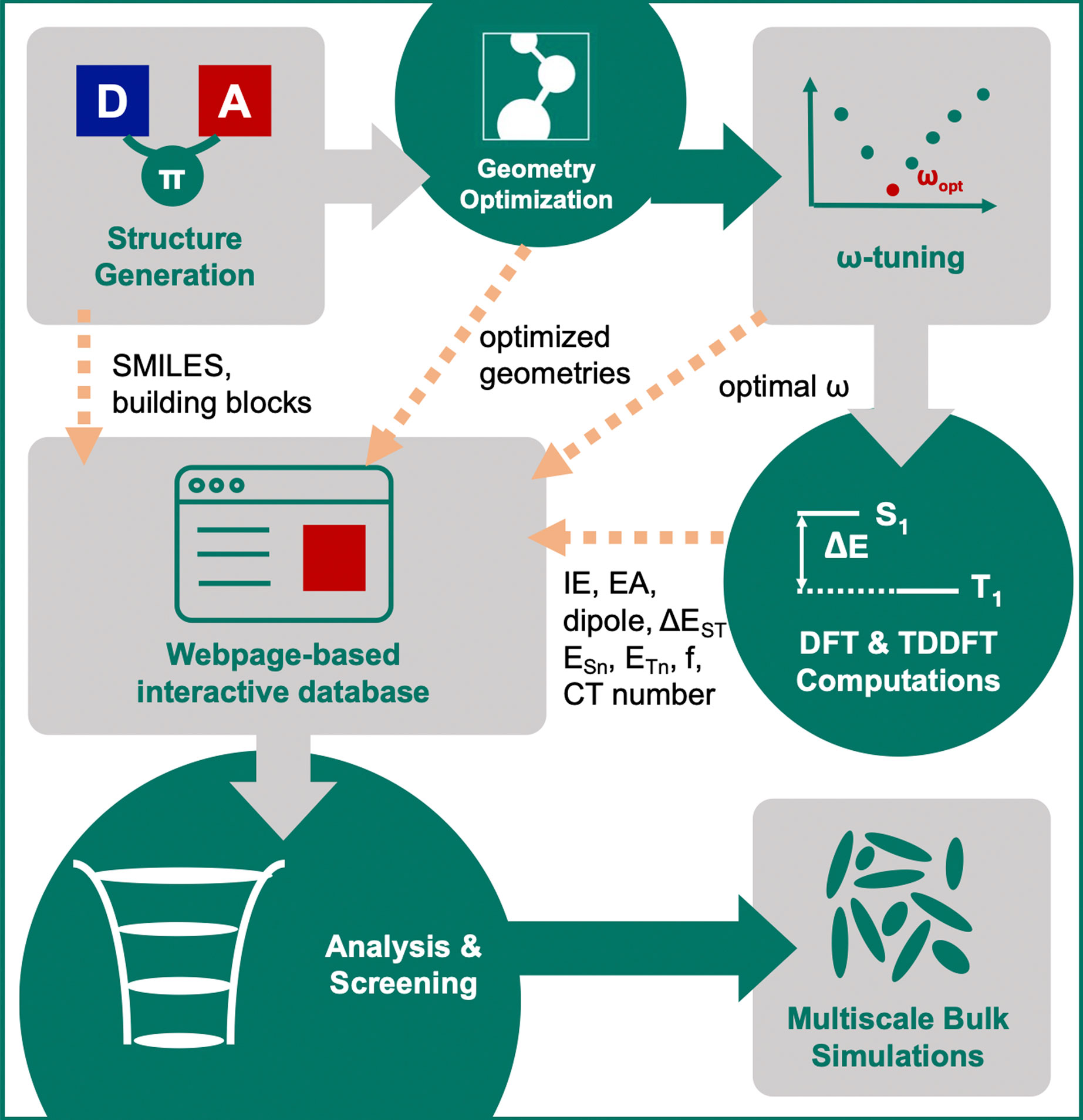
FIGURE 3. Illustration of the computational workflow for virtual screening of single-layer TADF emitters.
Results and Discussion
Compounds With Small Singlet-Triplet Splitting
The combination of the core and the arm fragments gives in total 441 A-
The IE and EA of all compounds either lies within the “trap-free window” or close to the borderline of the window, showing that the effectiveness of prescreening of the building blocks. Therefore, we put our emphasis on the small
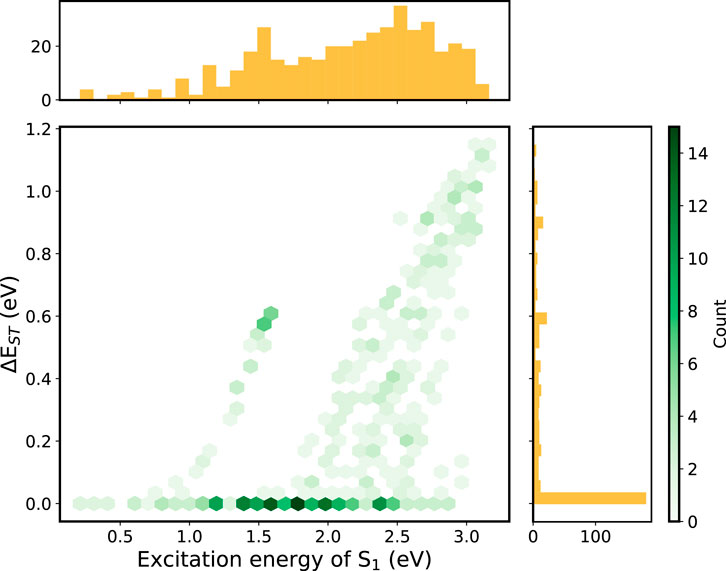
FIGURE 4. 2D histogram constructed using the descriptors (
Among these small-
Analysis of the Excited-State Character
To better understand the origin of the large
Figure 5 depicts the 2D histogram based on the CT numbers of T1 and S1 states for the 227 A-
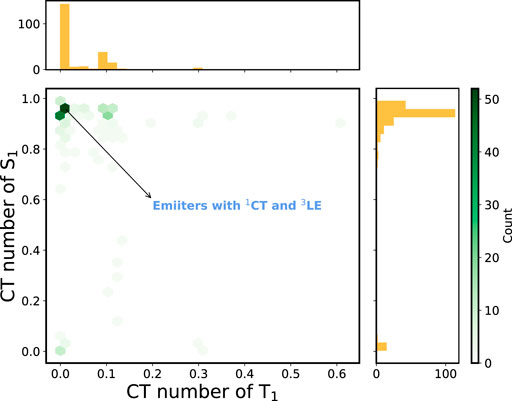
FIGURE 5. 2D histogram constructed using the CT numbers of T1 and S1 states of the A-
The emergence of the LE states can be explained utilizing the frontier molecular orbital (FMO) energies of the constituent building blocks (Blaskovits et al., 2020), which is illustrated in Figure 6A. The competition between the CT excitation and LE excitation depends on the relative ordering of the FMOs. In this context, we can define two descriptors,
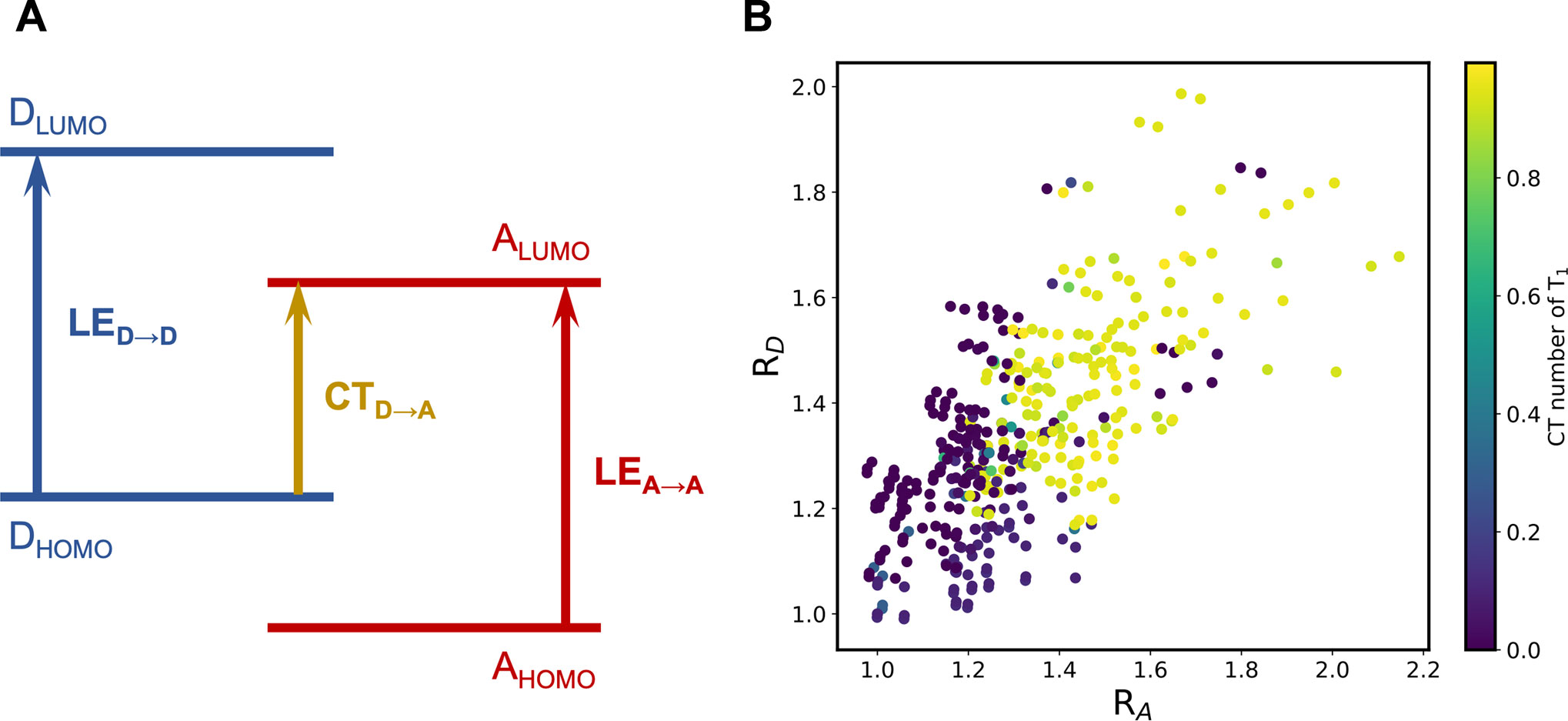
FIGURE 6. (A) Schematic representation of the relation between the competence of LE and CT states and the relative order of FMO energies; (B) RD-RA scatter plots colored by the CT number of the T1 state of the A-
Compounds With Tn States Lying Close to S1
The S1 and T1 states of most molecules that pass the first screening step (
In addition, higher triplet states (Tn with
Overall, around 100 molecules pass the criteria (49 A-
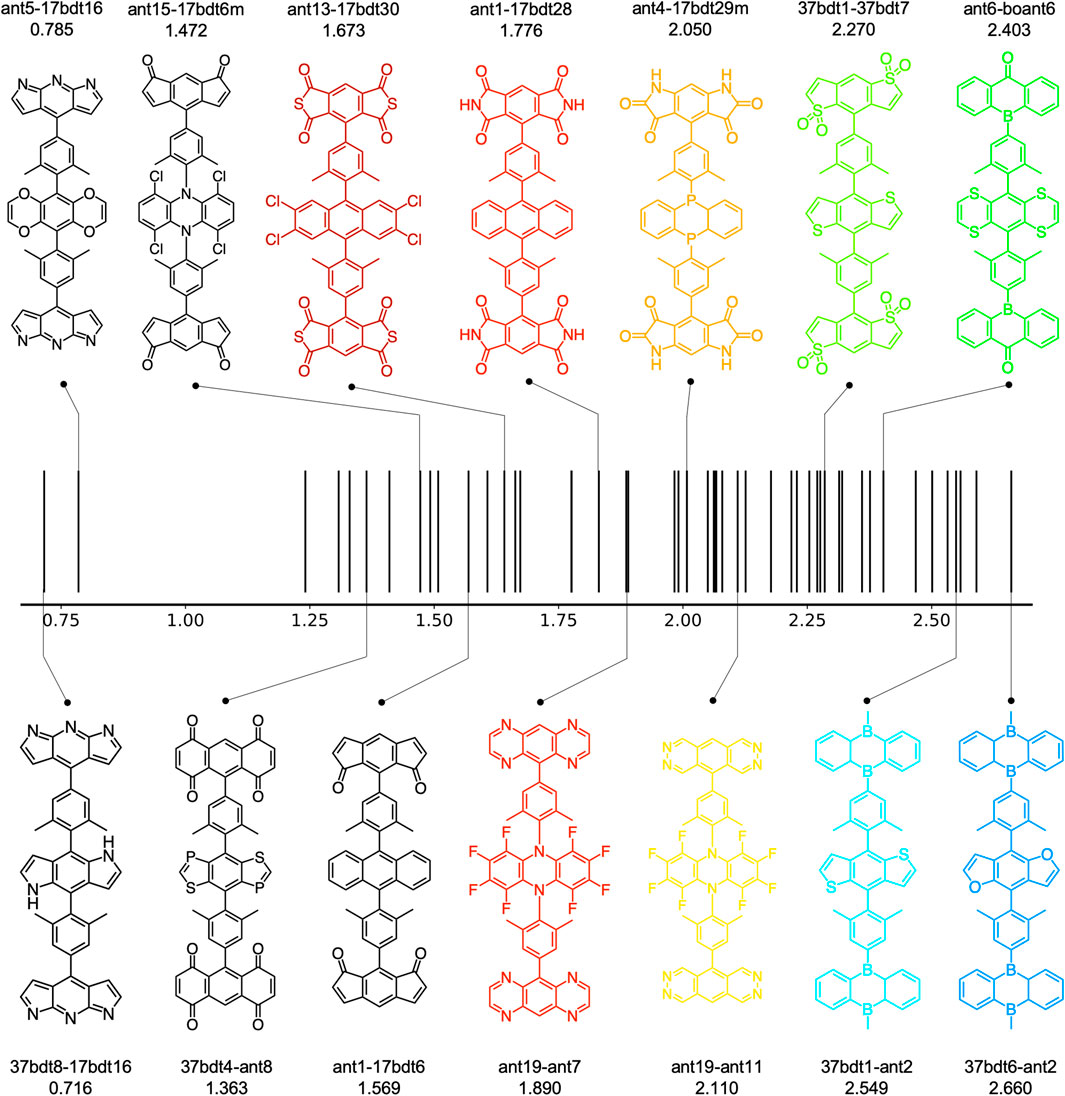
FIGURE 7. The estimated EL spectrum maximum of 49 A-
Charge Carrier Density of States
More sophisticated solid-state simulations can then be performed for the much smaller molecular dataset, which is now only ∼10% of the initial number of compounds. As a proof of concept, we computed the charge carrier density of states for the blue A-
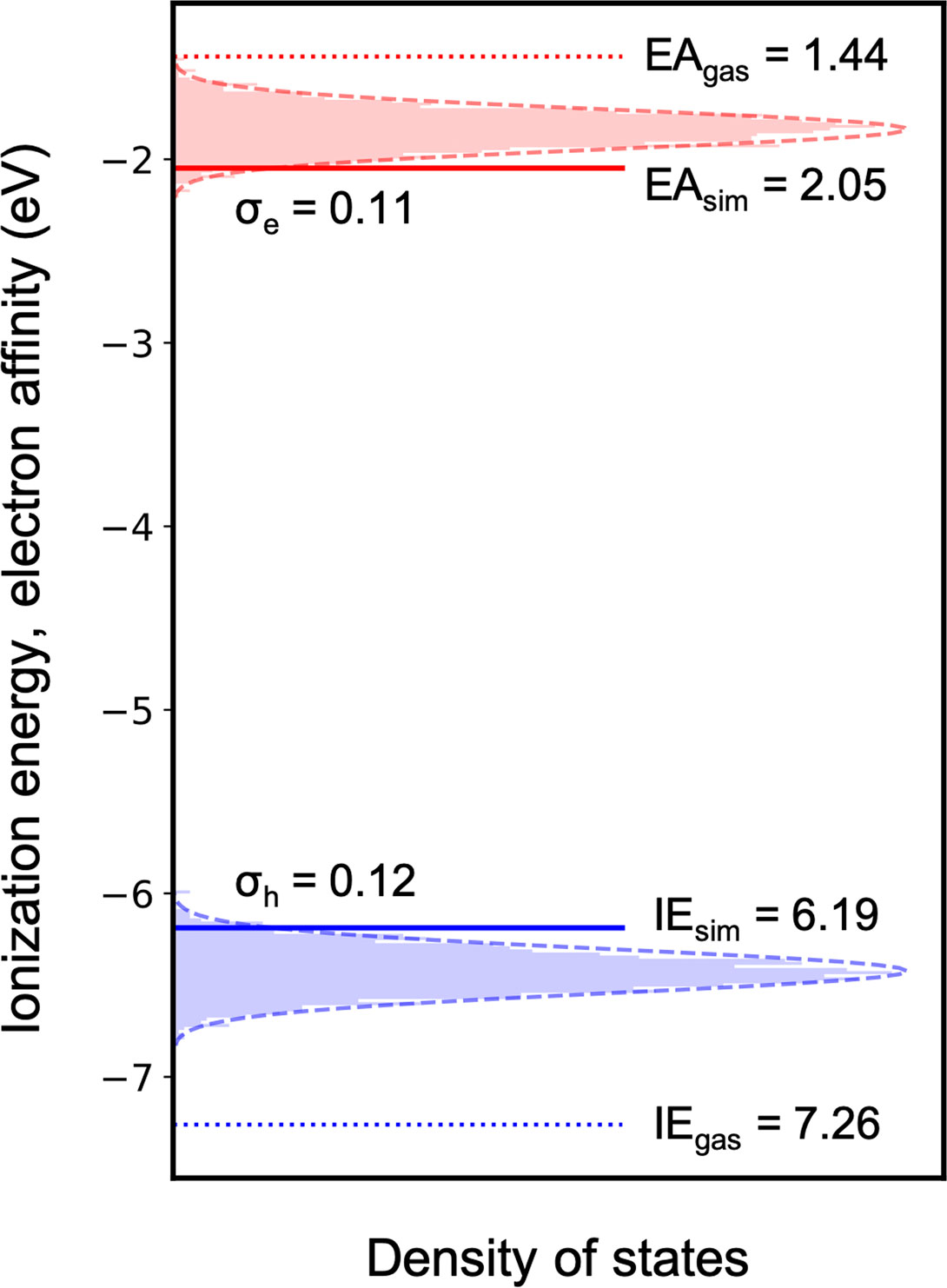
FIGURE 8. Simulated ionization energy and electron affinity distribution in an amorphous 37bdt1-ant2 film.
Conclusion
To summarize, we have provided clear design rules for single-layer OLED materials comprising TADF:
1. Molecular gas-phase ionization energies and electron affinities within the ∼ 6.2 eV to ∼ 2.0 eV range. These are calculated using implicit solvent with the dielectric constant of 3 and ensure trap-free transport of electrons and holes.
2. Small molecular dipole moment. This condition is imposed by the molecular symmetry and ensures a narrow density-of-states distribution in the solid state.
3. Small singlet-triplet splitting. This is provided by the orthogonal alignment of the bridge and the core units, as well as the suitable level alignment between the HOMO and LUMO of the donor and acceptor units. This is required for efficient reverse intersystem crossing.
4. Different character of singlet and triplet excitations to ensure sufficient spin-orbit coupling that enables reverse intersystem crossing.
Using the suggested design rules, we have proposed a set of TADF emitters with a broad range of emission wavelengths, from infrared to sky-blue. We hope that the suggested structures can serve as a clear guide towards further development of efficient and stable single-layer OLEDs.
Data Availability Statement
The datasets presented in this study can be found in online repository: https://gitlab.mpcdf.mpg.de/materials/tadf-screening.
Author Contributions
K-HL conceived the study, performed force-field parameterization, MD simulations, data analysis and prepared the online git repository and the first draft of the manuscript. DA supervised the work, discussed results and contributed to writing. G-JW and PB discussed results and contributed to writing.
Funding
This publication is based on work supported by the KAUST Office of Sponsored Research (OSR) under award nos. OSR-2018-CARF/CCF-3079 and OSR-CRG2018-3746.
Conflict of Interest
The authors declare that the research was conducted in the absence of any commercial or financial relationships that could be construed as a potential conflict of interest.
Publisher’s Note
All claims expressed in this article are solely those of the authors and do not necessarily represent those of their affiliated organizations, or those of the publisher, the editors and the reviewers. Any product that may be evaluated in this article, or claim that may be made by its manufacturer, is not guaranteed or endorsed by the publisher.
Acknowledgments
DA acknowledges the KAUST PSE Division for hosting his sabbatical in the framework of the Division’s Visiting Faculty program. DA acknowledges funding by the Deutsche Forschungsgemeinschaft (DFG, German Research Foundation) for financial support through the collaborative research centers TRR 146, SPP 2196, and grant number 460766640. K-HL acknowledges the financial support from the Swiss NSF Early Postdoc Mobility fellowship (Grant no. P2ELP2_195156). The authors thank Wenlan Liu, Mukunda Mandal, Christoph Scherer, Leanne Paterson and Naoimi Kinaret for fruitful discussions and proof-reading of the manuscript.
Supplementary Material
The Supplementary Material for this article can be found online at: https://www.frontiersin.org/articles/10.3389/fchem.2021.800027/full#supplementary-material
References
Andrienko, D. (2018). “Multiscale Concepts in Simulations of Organic Semiconductors,” in Handbook of Materials Modeling : Methods: Theory and Modeling. Editors W. Andreoni,, and S. Yip (Cham: Springer International Publishing), 1–12. doi:10.1007/978-3-319-42913-7_39-1
Blaskovits, J. T., Fumanal, M., Vela, S., and Corminboeuf, C. (2020). Designing Singlet Fission Candidates from Donor-Acceptor Copolymers. Chem. Mater. 32, 6515–6524. doi:10.1021/acs.chemmater.0c01784
de Silva, P., Kim, C. A., Zhu, T., and Van Voorhis, T. (2019). Extracting Design Principles for Efficient Thermally Activated Delayed Fluorescence (TADF) from a Simple Four-State Model. Chem. Mater. 31, 6995–7006. doi:10.1021/acs.chemmater.9b01601
El‐Sayed, M. A. (1963). Spin-Orbit Coupling and the Radiationless Processes in Nitrogen Heterocyclics. J. Chem. Phys. 38, 2834–2838. doi:10.1063/1.1733610
Gibson, J., Monkman, A. P., and Penfold, T. J. (2016). The Importance of Vibronic Coupling for Efficient Reverse Intersystem Crossing in Thermally Activated Delayed Fluorescence Molecules. ChemPhysChem 17, 2956–2961. doi:10.1002/cphc.201600662
Godumala, M., Choi, S., Cho, M. J., and Choi, D. H. (2019). Recent Breakthroughs in Thermally Activated Delayed Fluorescence Organic Light Emitting Diodes Containing Non-Doped Emitting Layers. J. Mater. Chem. C 7, 2172–2198. doi:10.1039/C8TC06293E
Heimel, P., Mondal, A., May, F., Kowalsky, W., Lennartz, C., Andrienko, D., et al. (2018). Unicolored Phosphor-Sensitized Fluorescence for Efficient and Stable Blue OLEDs. Nat. Commun. 9, 4990. doi:10.1038/s41467-018-07432-2
Kotadiya, N. B., Blom, P. W. M., and Wetzelaer, G.-J. A. H. (2019a). Efficient and Stable Single-Layer Organic Light-Emitting Diodes Based on Thermally Activated Delayed Fluorescence. Nat. Photon. 13, 765–769. doi:10.1038/s41566-019-0488-1
Kotadiya, N. B., Mondal, A., Blom, P. W. M., Andrienko, D., and Wetzelaer, G.-J. A. H. (2019b). A Window to Trap-Free Charge Transport in Organic Semiconducting Thin Films. Nat. Mater. 18, 1182–1186. doi:10.1038/s41563-019-0473-6
Lin, K.-H., Prlj, A., Yao, L., Drigo, N., Cho, H.-H., Nazeeruddin, M. K., et al. (2019). Multiarm and Substituent Effects on Charge Transport of Organic Hole Transport Materials. Chem. Mater. 31, 6605–6614. doi:10.1021/acs.chemmater.9b00438
Liu, W., Kotadiya, N. B., Blom, P. W. M., Wetzelaer, G. J. A. H., and Andrienko, D. (2021). Molecular Origin of Balanced Bipolar Transport in Neat Layers of the Emitter CzDBA. Adv. Mater. Technol. 6, 2000120. doi:10.1002/admt.202000120
Mondal, A., Paterson, L., Cho, J., Lin, K.-H., van der Zee, B., Wetzelaer, G.-J. A. H., et al. (2021). Molecular Library of OLED Host Materials-Evaluating the Multiscale Simulation Workflow. Chem. Phys. Rev. 2, 031304. doi:10.1063/5.0049513
Novikov, S. V., and Vannikov, A. V. (2009). Hopping Charge Transport in Disordered Organic Materials: Where Is the Disorder? J. Phys. Chem. C 113, 2532–2540. doi:10.1021/jp808578b
Paterson, L., Mondal, A., Heimel, P., Lovrincic, R., May, F., Lennartz, C., et al. (2019). Perspectives of Unicolored Phosphor‐Sensitized Fluorescence. Adv. Electron. Mater. 5, 1900646. doi:10.1002/aelm.201900646
Paterson, L., May, F., and Andrienko, D. (2020). Computer Aided Design of Stable and Efficient OLEDs. J. Appl. Phys. 128, 160901. doi:10.1063/5.0022870
Plasser, F. (2020). TheoDORE: A Toolbox for a Detailed and Automated Analysis of Electronic Excited State Computations. J. Chem. Phys. 152, 084108. doi:10.1063/1.5143076
Poelking, C., and Andrienko, D. (2016). Long-Range Embedding of Molecular Ions and Excitations in a Polarizable Molecular Environment. J. Chem. Theor. Comput. 12, 4516–4523. doi:10.1021/acs.jctc.6b00599
Rühle, V., Lukyanov, A., May, F., Schrader, M., Vehoff, T., Kirkpatrick, J., et al. (2011). Microscopic Simulations of Charge Transport in Disordered Organic Semiconductors. J. Chem. Theor. Comput. 7, 3335–3345. doi:10.1021/ct200388s
Stankevych, A., Vakhnin, A., Andrienko, D., Paterson, L., Genoe, J., Fishchuk, I., et al. (2021). Density of States of OLED Host Materials from Thermally Stimulated Luminescence. Phys. Rev. Appl. 15, 044050. doi:10.1103/PhysRevApplied.15.044050
Sun, H., Ryno, S., Zhong, C., Ravva, M. K., Sun, Z., Körzdörfer, T., et al. (2016). Ionization Energies, Electron Affinities, and Polarization Energies of Organic Molecular Crystals: Quantitative Estimations from a Polarizable Continuum Model (PCM)-Tuned Range-Separated Density Functional Approach. J. Chem. Theor. Comput. 12, 2906–2916. doi:10.1021/acs.jctc.6b00225
Sun, H., Hu, Z., Zhong, C., Chen, X., Sun, Z., and Brédas, J.-L. (2017). Impact of Dielectric Constant on the Singlet-Triplet Gap in Thermally Activated Delayed Fluorescence Materials. J. Phys. Chem. Lett. 8, 2393–2398. doi:10.1021/acs.jpclett.7b00688
Uoyama, H., Goushi, K., Shizu, K., Nomura, H., and Adachi, C. (2012). Highly Efficient Organic Light-Emitting Diodes from Delayed Fluorescence. Nature 492, 234–238. doi:10.1038/nature11687
Weissenseel, S., Drigo, N. A., Kudriashova, L. G., Schmid, M., Morgenstern, T., Lin, K.-H., et al. (2019). Getting the Right Twist: Influence of Donor-Acceptor Dihedral Angle on Exciton Kinetics and Singlet-Triplet Gap in Deep Blue Thermally Activated Delayed Fluorescence Emitter. J. Phys. Chem. C 123, 27778–27784. doi:10.1021/acs.jpcc.9b08269
Keywords: TADF, computer screening, OLED, chemical design, single-layer
Citation: Lin K-H, Wetzelaer G-JAH, Blom PWM and Andrienko D (2021) Virtual Screening of TADF Emitters for Single-Layer OLEDs. Front. Chem. 9:800027. doi: 10.3389/fchem.2021.800027
Received: 22 October 2021; Accepted: 17 November 2021;
Published: 16 December 2021.
Edited by:
Paul Winget, Schrodinger, United StatesReviewed by:
Juan-Carlos Sancho-Garcia, University of Alicante, SpainXiankai Chen, City University of Hong Kong, Hong Kong SAR, China
Copyright © 2021 Lin, Wetzelaer, Blom and Andrienko. This is an open-access article distributed under the terms of the Creative Commons Attribution License (CC BY). The use, distribution or reproduction in other forums is permitted, provided the original author(s) and the copyright owner(s) are credited and that the original publication in this journal is cited, in accordance with accepted academic practice. No use, distribution or reproduction is permitted which does not comply with these terms.
*Correspondence: Kun-Han Lin, bGlua0BtcGlwLW1haW56Lm1wZy5kZQ==; Denis Andrienko, ZGVuaXMuYW5kcmllbmtvQG1waXAtbWFpbnoubXBnLmRl