- Chongqing Key Laboratory of Photoelectric Functional Materials, College of Physics and Electronic Engineering, Chongqing Normal University, Chongqing, China
Metal oxide semiconductor gas sensing materials have attracted great research interest in the gas sensor field due to their outstanding physical and chemical properties, low cost, and easy preparation. Among them, two-dimensional hexagonal tungsten trioxide (2D h-WO3) is especially interesting because of its high sensitivity and selectivity to some gases. We firstly introduce the characteristics of 2D h-WO3 gas sensing materials and discuss the effects of microstructure, oxygen vacancy, and doping modification on the gas sensing properties of 2D h-WO3 mainly. Finally, we explore the application of 2D h-WO3 gas sensing materials and propose some research directions.
Introduction
As a critical component of the intelligent detection system, the gas sensor (Lee et al., 2018) has been widely used in environmental monitoring (Ji et al., 2019a), respiratory analysis, explosive gases, and automobile exhaust detection. Based on different working mechanisms, the developed gas sensors include semiconductor gas sensors (Morrison, 1987a; Zhang et al., 2021), polymer gas sensors (Zee and Judy, 2001), and electrochemical gas sensors (Tierney and Kim, 1993). Among them, the semiconductor gas sensors can also be divided into resistive and non-resistive types, while the resistive semiconductor gas sensors have advantages of high sensitivity and easy preparation (Seiyama et al., 1962). Meanwhile, compared with carbon and other organic gas sensing materials, the resistive metal oxide gas sensors (Nazemi et al., 2019) have become the research hotspot due to their high responsivity (Demarne and Grisel, 1988) and excellent selectivity (Morrison, 1987b). As a highly sensitive metal oxide gas sensing material, tungsten trioxide (WO3) has attracted extensive attention because of its unique physical and chemical properties (Salje and Viswanathan, 1975), and its applications in photocatalysis (Dong et al., 2017) and electrochromic (Adhikari and Sarkar, 2014).
WO3 is a typical metal oxide semiconductor with various phase transition structures, while different phases can induce different gas sensitivity. The stable structures at room temperature are m-WO3 and h-WO3. In recent years, as the most stable structure, m-WO3 has attracted much attention (Hübner et al., 2010; Oison et al., 2011), but bulk m-WO3 gas sensors are not sensitive to some gases at 25°C–500°C, such as CO (Ahsan et al., 2012) and H2S (Szilágyi et al., 2010). Therefore, it is urgent to improve the gas sensitivity of WO3 at room temperature effectively. Xu et al. (2008) found that the sensitivity of h-WO3 almost linearly increases with CO concentration at room temperature. Szilágyi et al. (2010) found that h-WO3 becomes more sensitive than m-WO3 compared to m-WO3 when the concentration of H2S is 10 ppm. Meanwhile, the large hexagonal and trigonal tunnel structures of h-WO3 result in it having a high specific surface area (as shown in Figure 1) (Balaji et al., 2009), indicating that h-WO3 is an excellent candidate material for gas sensors.
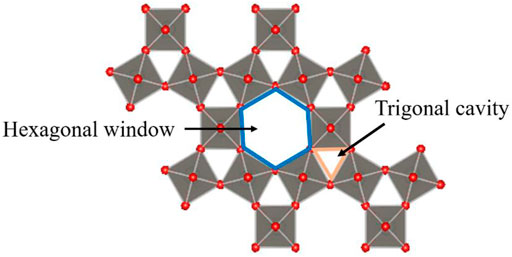
FIGURE 1. The hexagonal window and trigonal cavity of the hexagonal WO3(001) surface (Balaji et al., 2009).
To effectively improve the gas sensitivity of the material, various preparation methods have been used to prepare WO3 nanomaterials on various dimensions (0D, 1D, 2D, and 3D) (Qin et al., 2010; Zhang et al., 2010; D'Arienzo et al., 2014). Among them, 2D nanomaterials are widely used because of their high specific surface area and abundant oxygen vacancies (Yang et al., 2016; Liu et al., 2017; Yang et al., 2017). The unique characteristics of 2D WO3 nanostructure compared with the bulk material include (1) higher specific surface area, which will provide more interaction area between tested gases and gas sensor surface molecules; (2) quantum confinement effects, due to the inherently small size of nanostructure materials, that can significantly affect charge transport, electronic band structure, and optical properties (Zheng et al., 2011). Based on this, we mainly review the effects of microstructure, oxygen vacancy, and doping modification on the gas sensing performance of 2D h-WO3 and explore the application prospect of the 2D h-WO3 gas sensor.
Characteristics of 2D h-WO3 Gas Sensing Material
As a kind of metal oxide semiconductor, 2D h-WO3 gas sensing material has been an excellent candidate material for gas sensors due to its advantages of easy preparation, stable crystal structure, high specific surface area, and abundant oxygen vacancies.
Easy Preparation
Table 1 shows some typical preparation methods of 2D h-WO3. Among them, the hydrothermal method is the most widely used. According to this method (Kitagawa et al., 2009; Szilágyi et al., 2010; Ji et al., 2019b), (NH4)10W12O41∙5H2O is firstly put into a high-pressure cauldron as the raw material. Then, under high temperature and high pressure, (NH4)10W12O41∙5H2O recrystallizes to obtain precipitates (h-WO3 crystals). Finally, the precipitates are removed and washed several times with deionized water to obtain the final product. Compared with vapor/liquid phase deposition methods, the hydrothermal method is simple and economical, and can prepare nanomaterials with high purity, good chemical uniformity and high dispersion. 2D h-WO3 is classified as the surface-controlled gas sensor by a gas sensing mechanism.
Stable Crystal Structure
The phases of WO3 can transform when it is annealed under different conditions. However, it does not simply form new nanostructures, but the original WO6 octahedron distorts and twists to a certain extent and thus can form different crystal phases. The phase transition with temperature of WO3 is shown in Figure 2 (Salje et al., 1997; Vogt et al., 1999; Roussel et al., 2000), which is monoclinic II (ε-WO3 < −43°C) → triclinic (−43°C < σ-WO3 < 17°C) → monoclinic I (17°C < m-WO3 < 330°C) → orthorhombic (330°C < β-O3 < 740°C) → tetragonal (740°C < α-WO3). Meanwhile, Gerand et al. (1979) found that stable hexagonal WO3 (h-WO3) can be prepared by dehydration method at 200°C–400°C.

FIGURE 2. Stability temperature domains of the different polymorphs of WO3 (Gerand et al., 1979; Roussel et al., 2000).
Tian et al. (2020) has calculated the gas (O2) sensing on hexagonal WO3 (001) surface by using the pseudopotentials method based on the density functional theory (DFT). The formation energy (Eform) of the h-WO3 (001) monolayer is −5.87 eV, indicating that the h-WO3 (001) monolayer is stable. The carrier mobility μ calculated from the energy band is 886 cm2V−1s−1 (as shown in Table 2) at T = 300 K. The value is higher than that of 2D GeP3 (Gerand et al., 1979) and MoS2 (Cai et al., 2014) and is about 88 times higher than that of bulk WO3 (Yamazoe et al., 2003), which implies that 2D h-WO3 may have excellent gas sensing performance.
High Specific Surface Area
Sun et al. (2015) investigated the high surface area tunnels in 3D h-WO3 by low-pressure CO2 adsorption isotherms with nonlocal density functional theory fitting (NLDET), transmission electron microscopy (TEM), and thermal gravimetric analysis. They found that h-WO3 has a large hexagonal tunnel structure (the diameter is 3.67 Å) and high specific surface area (45.585 m2/g). Meanwhile, the large lateral size and ultrathin thickness of 2D materials provide it with ultrahigh specific surface areas and high ratios of exposed surface atoms (Zhang, 2015), significantly improving the gas sensing performance of 2D h-WO3.
Abundant Oxygen Vacancies
The conduction band of 2D WO3 mainly consists of W-5d electrons, and the valence band mainly consists of O-2p electrons (Niklasson et al., 2004). Chatten et al. (2005) found that abundant oxygen vacancies are related to the energy gap between O-2p and W-5d orbitals in non-stoichiometric tungsten oxide. Makarov and Trontelj (1996) pointed out that the oxygen vacancies in 2D WO3 can affect the conductivity and carrier concentration, and further affect the gas sensing performance of WO3. For example, Tian et al. (2020) found that oxygen vacancies provide electrons to O2 gas molecules on the WO-terminated h-WO3 (001) surface, thus effectively improving the gas sensing performance of h-WO3 (001) surface to O2.
Influencing Factors of 2D h-WO3 on Gas Sensing Performance
When the gas sensors are exposed to the air, O2 molecules are physically or chemically adsorbed on the surface of 2D h-WO3. The oxygen will be dissociated and capture the electrons from the conduction bands of 2D h-WO3, generating ionized oxygen species (mainly O−). This leads to a decrease in the number of electrons on the surface and forming an electron depletion region (EDR), which causes the first change in resistance. When the sensors are exposed to the target gas, the gas molecules are adsorbed on the surface of 2D h-WO3. Then, the gas molecules react with pre-absorbed oxygen and change the number of the electrons of ionized oxygen species, increasing the density of carriers in the 2D h-WO3. It results in the second change in resistance (Deng et al., 2015; Li et al., 2015; Liu et al., 2016).
Effect of Microstructure on Gas Sensing Performance of 2D h-WO3
Figure 3 shows different microstructures of h-WO3. It can be seen that h-WO3 nanosheets and films can provide more gas molecular absorption sites because of their obvious orientation, small particle size, large specific surface area, and no agglomeration. However, h-WO3 nanoparticles, nanowires, and nanospheres have a negative effect on gas transportation and reaction due to serious agglomeration or large particle size. Moreover, we also find from Table 3 that h-WO3 nanosheets and films have the highest responsiveness (R) and wider detection scope (S) to H2, NH3, H2S, and NO2, compared with nanowires, nanorods, nanospheres, and nanoparticles. Different h-WO3 nanomaterials have exhibited different gas sensing performance due to their different microstructures. Among them, 2D h-WO3 nanomaterials show important application prospects in the gas sensing field due to their excellent gas sensing performance.
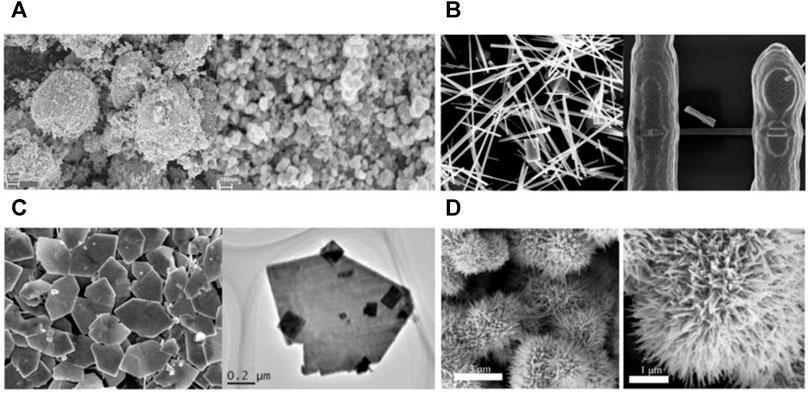
FIGURE 3. The microstructure of h-WO3 nanoparticle, nanowire, film, and nanosphere (A) 0D h-WO3 nanoparticle (Szilágyi et al., 2010). (B) 1D h-WO3 nanowire (Liu et al., 2014). (C) 2D h-WO3 film (Meng et al., 2015). (D) 3D h-WO3 nanosphere (Zhang et al., 2019).
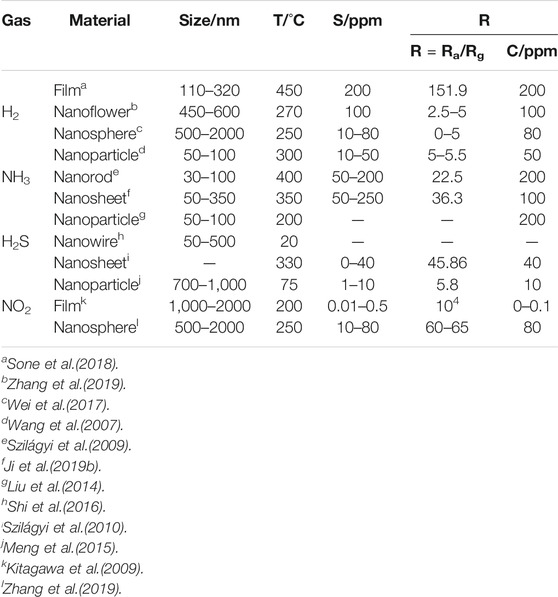
TABLE 3. Relationship between microstructure, particle size, and gas sensitivity of H2, NH3, H2S, and NO2 in h-WO3 (S is the detection scope, R is the responsiveness, and C is concentration).
Effect of Oxygen Vacancy on Gas Sensing Performance of 2D h-WO3
In 1964, Kevane (1964) found that oxygen vacancies are easy to form in the preparation of metal oxide semiconductors. Makarov and Trontelj (1996) found that the oxygen vacancies would affect the conductivity, thus further affecting the gas sensing performance of WO3. However, the expression of oxygen vacancy on metal oxide semiconductor surfaces is not in agreement (Gillet et al., 2003). Until 2018, Tian et al. (2018) established a theory based on surface oxygen density (do) and unitedly expressed the oxygen vacancies on the WO3 surface (Table 4). The O-terminated and WO-terminated h-WO3 (001) surfaces with and without oxygen vacancy are denoted as O- for O-terminated, Vac O- for defective O-terminated, WO- for WO-terminated, and Vac WO- for defective WO-terminated, respectively. The surface oxygen densities are defined as do = 1, 1 > do > 0, do = 0, 0 > do > −1. Based on this, oxygen vacancies of the 2D h-WO3 surface can be expressed by surface oxygen density.
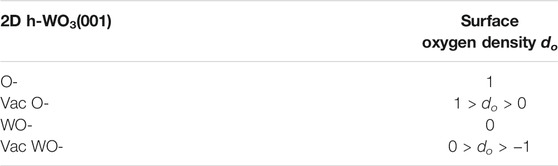
TABLE 4. The relationship between surface oxygen vacancy and oxygen density of 2D h-WO3 (Tian et al., 2018).
Recently, Tian et al. (2014) investigated the effect of oxygen vacancy on the gas sensing performance of CO on 2D h-WO3 (001) surface by using the first-principles calculations (Table 5). They found that the adsorption energy and charge transfer of CO of the defective O-terminated h-WO3 (001) surface decrease by 0.68 eV and 0.002e, respectively, compared with the O-terminated h-WO3 (001) surface. For defective WO-terminated, the values of decrease are 0.4 eV and 0.011e, respectively. The result shows that the adsorption and sensing ability of CO on the defective O- and WO-terminated h-WO3 (001) surface decreases. The oxygen vacancy inhibits the oxidation reaction of reducing gas CO on the 2D h-WO3 (001) surface, which reduces the gas sensing performance of the 2D h-WO3.
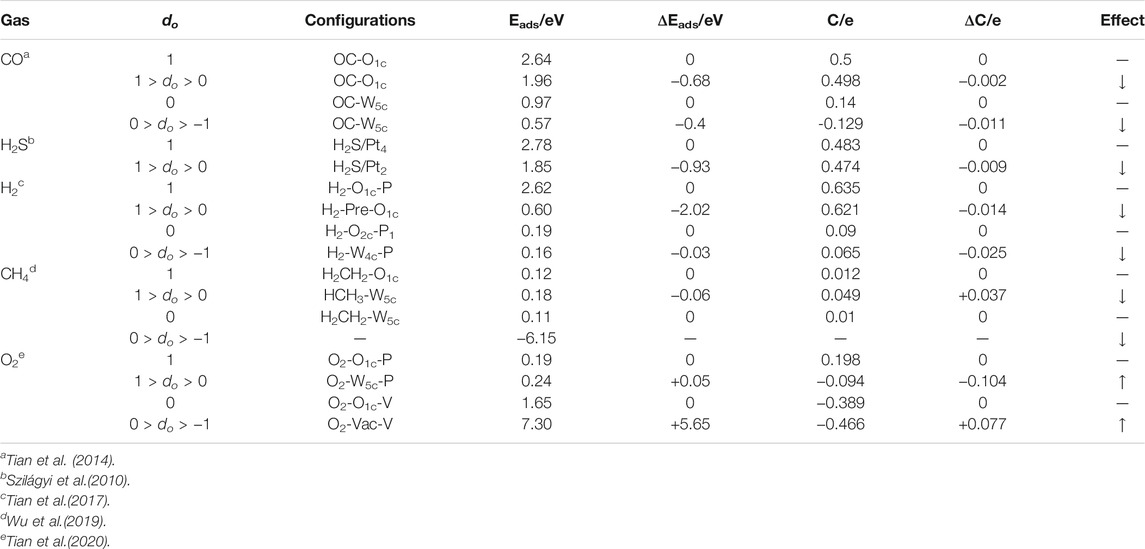
TABLE 5. The adsorption energy and charge transfer of O2, CO, H2, H2S, and CH4 on 2D h-WO3 (001) surface with oxygen vacancy (do is surface oxygen density, C is charge transfer, ΔC is the variation of charge transfer, ↑ is promotion, ↓ is reduction).
Oxygen vacancy also inhibits the gas sensing performance of other reducing gases (H2S, CH4, H2) on the 2D h-WO3 (001) surface (Szilágyi et al., 2010; Tian et al., 2017; Wu et al., 2019) (Table 5). However, the inhibitory effect of oxygen vacancy on H2S and CH4 is unapparent. Although the gas sensing performance of H2S is inhibited by oxygen vacancy, the value (1.85 eV) is still large enough for effective adsorption of H2S on the surface. The adsorption sensing ability of CH4 on the 2D h-WO3 (001) surface is weak and the inhibition of oxygen vacancy makes it difficult to spontaneously adsorb on defective WO-terminated h-WO3 (001) surface. Moreover, oxygen vacancy has the strongest inhibitory effect on the gas sensing performance of H2 on the 2D h-WO3 (001) surface. The adsorption energy decreases from 2.62 to 0.16 eV and the charge transfer decreases from 0.635e to 0.065e. The gas adsorption ability of H2 on the 2D h-WO3 (001) surface greatly reduces with the decrease of surface oxygen density.
More recently, Tian et al. (2020) investigated the effect of oxygen vacancy on the gas sensing performance of O2 on the 2D h-WO3 (001) surface (Table 5) by the first principles with pseudopotentials method based on the DFT. They found that the adsorption energy of O2 of the defective O-terminated h-WO3 (001) surface increases by 0.05 eV and the charge transfer decreases by 0.104e compared with the O-terminated h-WO3 (001) surface. For the defective WO-terminated surface, the values of increase are 5.65 eV and 0.077e, relatively. The result shows that the adsorption and sensing ability of O2 are improved on the defective O- and WO-terminated h-WO3 (001) surface. The oxygen vacancy activates the O-O bond of O2 and promotes the reduction reaction of oxidizing gas O2 on the 2D h-WO3 (001) surface, which improves the gas sensing performance of the 2D h-WO3.
These results indicate that the effect of oxygen vacancy on gases with different redox properties is different. For reducing gases, the oxygen vacancy inhibits their oxidation reactions on the 2D h-WO3 (001) surface and then reduces the gas sensing performance of the reducing gases. On the contrary, for oxidizing gases, the oxygen vacancy promotes the reduction reaction and then improves the gas sensing performance.
Effect of Doping Modification on Gas Sensing Performance of 2D h-WO3
Various methods have been performed to improve the gas sensing performance, to overcome the defects of pure metal oxides such as low sensitivity, low selectivity, and long response time for some gases (Liu et al., 2019). Among them, noble metal doping is one of the most common and effective methods. Due to the high electronic activity of noble metal elements, the activation energy of the reaction can be reduced during the contact reaction between the gas sensing material and the target gas, thus improving the gas sensing performance of the materials (Xu et al., 1990) when they react with target gases. Based on this, noble metals such as Au, Ag, Pd, and Pt are usually doped on WO3 films to improve their sensitivity and selectivity to NOx (Penza et al., 1998; Chen and Tsang, 2003), H2S (Stankova et al., 2004; Hurtado-Aular et al., 2021), CH3COCH3 (Feng et al., 2021), etc.
Recently, the gas sensing performance of CO adsorption on the 2D h-WO3 (001) surface doped with noble metals Cu, Ag, and Au were investigated by using DFT (as shown in Table 6) (Hurtado-Aular et al., 2021). They found that the incorporation of Au and Cu atoms improves the surface activity of the material and the absorptivity of CO on the 2D h-WO3 (001) surface. Meanwhile, the doped Au and Cu atoms provide a large number of electrons. The charge transfer increases, which effectively improves the sensing performance of CO on the 2D h-WO3 (001) surface.
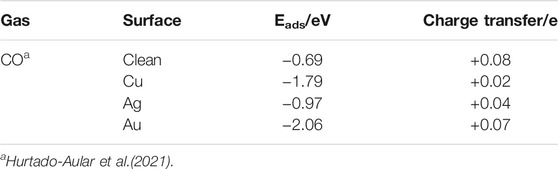
TABLE 6. Adsorption energy and charge transfer of CO and H2S on noble metal doped 2D h-WO3 (001) surface.
Theoretically, noble metal doping promotes the adsorption and sensing ability of the target gas on 2D h-WO3 surface, and then improves the gas sensing performance of 2D h-WO3. However, the experimental study on the mechanism of improving the gas sensing performance of noble metal doped h-WO3 films is still insufficient.
Summary and Prospect
The research progress of the gas sensing performance of 2D h-WO3 has been reviewed. Firstly, we briefly summarize the characteristics of 2D h-WO3 gas sensing materials. Then, the effects of microstructure, oxygen vacancy, and doped metal on the performance of 2D h-WO3 gas sensors are mainly discussed. We find that the 2D h-WO3 gas sensor has better gas sensing performance than other WO3 nanomaterials due to their small particle size and large specific surface area. Moreover, the effect of oxygen vacancy on the gas sensitivity of different oxidation-reducing gases on 2D h-WO3 is different. Besides, we also note that noble metal doping can improve the gas sensing performance of 2D h-WO3 due to the high electronic activity of noble metals and the reduction of reaction activation energy.
As we all know, 2D h-WO3 is an excellent candidate material for metal oxide semiconductor gas sensors, which has vital research significance and wide application prospects in gas sensors. However, there are still some unsolved problems in 2D h-WO3 that need to be completely solved, such as the low sensitivity and low selectivity to some gases. To solve the above problems, the possible solutions include the following: (1) Photoactivation method (i.e., activation of reactants by light), which can improve the sensitivity and selectivity effectively. Deng et al. (2012) activated mesoporous WO3 sensing material and improved the sensitivity of WO3 to HCHO by using visible light irradiation at room temperature. Moreover, Trawka et al. (2016) enhanced the sensitivity and selectivity of WO3-based gas sensors greatly by ultraviolet irradiation. (2) Noble metal doping method improves sensitivity and selectivity. Adding precious metal catalysts has become an important method to improve the gas sensing performance of metal oxide semiconductors, because the catalyst has a great influence on the resistance and sensitivity of semiconductor gas sensing materials (Krebs and Grisel, 1993).
Author Contributions
All authors listed have made a substantial, direct, and intellectual contribution to the work, and approved it for publication.
Funding
The work described in this paper is supported by Chongqing Natural Science Foundation of China (Grant No. cstc2019jcyj-msxmX0251), the Science and Technology Research Program of Chongqing Education Commission of China (Grant No. KJQN202000505), the Doctoral Fund Project of Chongqing Normal University (Grant No. 20XLB001), and the undergraduate innovation and entrepreneurship training program of Chongqing (Grant No. S202110637121).
Conflict of Interest
The authors declare that the research was conducted in the absence of any commercial or financial relationships that could be construed as a potential conflict of interest.
Publisher’s Note
All claims expressed in this article are solely those of the authors and do not necessarily represent those of their affiliated organizations, or those of the publisher, the editors, and the reviewers. Any product that may be evaluated in this article, or claim that may be made by its manufacturer, is not guaranteed or endorsed by the publisher.
References
Adhikari, S., and Sarkar, D. (2014). High Efficient Electrochromic WO3 Nanofibers. Electrochimica Acta 138, 115–123. doi:10.1016/j.electacta.2014.06.062
Ahsan, M., Tesfamichael, T., Ionescu, M., Bell, J., and Motta, N. (2012). Low Temperature CO Sensitive Nanostructured WO3 Thin Films Doped with Fe. Sensors Actuators B: Chem. 162 (1), 14–21. doi:10.1016/j.snb.2011.11.038
Alsaif, M. M. Y. A., Chrimes, A. F., Daeneke, T., Balendhran, S., Bellisario, D. O., Son, Y., et al. (2016). High-Performance Field Effect Transistors Using Electronic Inks of 2D Molybdenum Oxide Nanoflakes. Adv. Funct. Mater. 26 (1), 91–100. doi:10.1002/adfm.201503698
Balaji, S., Djaoued, Y., Albert, A.-S., Ferguson, R. Z., and Brüning, R. (2009). Hexagonal Tungsten Oxide Based Electrochromic Devices: Spectroscopic Evidence for the Li Ion Occupancy of Four-Coordinated Square Windows. Chem. Mater. 21 (7), 1381–1389. doi:10.1021/cm8034455
Balázsi, C., Wang, L., Zayim, E. O., Szilágyi, I. M., Sedlacková, K., Pfeifer, J., et al. (2008). Nanosize Hexagonal Tungsten Oxide for Gas Sensing Applications. J. Eur. Ceram. Soc. 28 (5), 913–917. doi:10.1016/j.jeurceramsoc.2007.09.001
Cai, Y., Zhang, G., and Zhang, Y.-W. (2014). Polarity-Reversed Robust Carrier Mobility in Monolayer MoS2 Nanoribbons. J. Am. Chem. Soc. 136 (17), 6269–6275. doi:10.1021/ja4109787
Chatten, R., Chadwick, A. V., Rougier, A., and Lindan, P. J. D. (2005). The Oxygen Vacancy in Crystal Phases of WO3. J. Phys. Chem. B 109 (8), 3146–3156. doi:10.1021/jp045655r
Chen, L., and Tsang, S. C. (2003). Ag Doped WO3-Based Powder Sensor for the Detection of NO Gas in Air. Sensors Actuators B: Chem. 89 (1), 68–75. doi:10.1016/S0925-4005(02)00430-6
D'Arienzo, M., Armelao, L., Mari, C. M., Polizzi, S., Ruffo, R., Scotti, R., et al. (2014). Surface Interaction of WO3 Nanocrystals with NH3. Role of the Exposed crystal Surfaces and Porous Structure in Enhancing the Electrical Response. RSC Adv. 4 (22), 11012–11022. doi:10.1039/C3RA46726K
Demarne, V., and Grisel, A. (1988). An Integrated Low-Power Thin-Film CO Gas Sensor on Silicon. Sensors and Actuators 13 (4), 301–313. doi:10.1016/0250-6874(88)80043-X
Deng, J., Zhang, R., Wang, L., Lou, Z., and Zhang, T. (2015). Enhanced Sensing Performance of the Co3O4 Hierarchical Nanorods to NH3 Gas. Sensors Actuators B: Chem. 209, 449–455. doi:10.1016/j.snb.2014.11.141
Deng, L., Ding, X., Zeng, D., Tian, S., Li, H., and Xie, C. (2012). Visible-light Activate Mesoporous WO3 Sensors with Enhanced Formaldehyde-Sensing Property at Room Temperature. Sensors Actuators B: Chem. 163 (1), 260–266. doi:10.1016/j.snb.2012.01.049
Dong, P., Hou, G., Xi, X., Shao, R., and Dong, F. (2017). WO3-based Photocatalysts: Morphology Control, Activity Enhancement and Multifunctional Applications. Environ. Sci. Nano 4 (3), 539–557. doi:10.1039/C6EN00478D
Feng, D.-L., Zhu, Z.-Y., Du, L.-L., Xing, X.-X., Wang, C., Chen, J., et al. (2021). Improved Sensing Performance of WO3 Nanoparticles Decorated with Ag and Pt Nanoparticles. Rare Met. 40 (6), 1642–1650. doi:10.1007/s12598-020-01666-0
Gerand, B., Nowogrocki, G., Guenot, J., and Figlarz, M. (1979). Structural Study of a New Hexagonal Form of Tungsten Trioxide. J. Solid State. Chem. 29 (3), 429–434. doi:10.1016/0022-4596(79)90199-3
Ghosh, B., Puri, S., Agarwal, A., and Bhowmick, S. (2018). SnP3: A Previously Unexplored Two-Dimensional Material. J. Phys. Chem. C 122 (31), 18185–18191. doi:10.1021/acs.jpcc.8b06668
Gillet, M., Lemire, C., Gillet, E., and Aguir, K. (2003). The Role of Surface Oxygen Vacancies upon WO3 Conductivity. Surf. Sci. 532-535, 519–525. doi:10.1016/S0039-6028(03)00477-1
Hübner, M., Simion, C. E., Haensch, A., Barsan, N., and Weimar, U. (2010). CO Sensing Mechanism with WO3 Based Gas Sensors. Sensors Actuators B: Chem. 151 (1), 103–106. doi:10.1016/j.snb.2010.09.040
Hurtado-Aular, O., Añez, R., and Sierraalta, A. (2021). DFT+U Study of the Electronic Structure Changes of WO3 Monoclinic and Hexagonal Surfaces upon Cu, Ag, and Au Adsorption. Applications for CO Adsorption. Surf. Sci. 714, 121907. doi:10.1016/j.susc.2021.121907
Ji, H., Zeng, W., and Li, Y. (2019a). Gas Sensing Mechanisms of Metal Oxide Semiconductors: a Focus Review. Nanoscale 11 (47), 22664–22684. doi:10.1039/C9NR07699A
Ji, H., Zeng, W., Xu, Y., and Li, Y. (2019b). Nanosheet-assembled Hierarchical WO3 Flower-like Nanostructures: Hydrothermal Synthesis and NH3-sensing Properties. Mater. Lett. 250, 155–158. doi:10.1016/j.matlet.2019.05.023
Kevane, C. J. (1964). Oxygen Vacancies and Electrical Conduction in Metal Oxides. Phys. Rev. 133 (5A), A1431–A1436. doi:10.1103/PhysRev.133.A1431
Kitagawa, C., Takahashi, A., Okochi, Y., and Tamaki, J. J. S. (2009). WO3 Crystals and Their NO2-Sensing Properties. Sensors Mater. 21 (5), 259–264. doi:10.18494/SAM.2009.611
Krebs, P., and Grisel, A. (1993). A Low Power Integrated Catalytic Gas Sensor. Sensors Actuators B: Chem. 13 (1), 155–158. doi:10.1016/0925-4005(93)85349-F
Lee, E., Yoon, Y. S., and Kim, D.-J. (2018). Two-Dimensional Transition Metal Dichalcogenides and Metal Oxide Hybrids for Gas Sensing. ACS Sens. 3 (10), 2045–2060. doi:10.1021/acssensors.8b01077
Li, L., Zhang, C., and Chen, W. (2015). Fabrication of SnO2-SnO Nanocomposites with P-N Heterojunctions for the Low-Temperature Sensing of NO2 Gas. Nanoscale 7 (28), 12133–12142. doi:10.1039/C5NR02334C
Liu, B., Tang, D., Zhou, Y., Yin, Y., Peng, Y., Zhou, W., et al. (2014). Electrical Characterization of H2S Adsorption on Hexagonal WO3 Nanowire at Room Temperature. J. Appl. Phys. 116 (16), 164310. doi:10.1063/1.4898127
Liu, T., Liu, J., Hao, Q., Liu, Q., Jing, X., Zhang, H., et al. (2016). Porous Tungsten Trioxide Nanolamellae with Uniform Structures for High-Performance Ethanol Sensing. CrystEngComm 18 (43), 8411–8418. doi:10.1039/C6CE01587E
Liu, W., Qu, Y., Li, H., Ji, F., Dong, H., Wu, M., et al. (2019). Nanostructure Bi2WO6: Surfactant-Assisted Hydrothermal Synthesis for High Sensitive and Selective Sensing of H2S. Sensors Actuators B: Chem. 294, 224–230. doi:10.1016/j.snb.2019.05.042
Liu, X., Ma, T., Pinna, N., and Zhang, J. (2017). Two-Dimensional Nanostructured Materials for Gas Sensing. Adv. Funct. Mater. 27 (37), 1702168. doi:10.1002/adfm.201702168
Makarov, V. O., and Trontelj, M. (1996). Sintering and Electrical Conductivity of Doped WO3. J. Eur. Ceram. Soc. 16 (7), 791–794. doi:10.1016/0955-2219(95)00204-9
Meng, Z., Fujii, A., Hashishin, T., Wada, N., Sanada, T., Tamaki, J., et al. (2015). Morphological and crystal Structural Control of Tungsten Trioxide for Highly Sensitive NO2 Gas Sensors. J. Mater. Chem. C 3 (5), 1134–1141. doi:10.1039/C4TC02762K
Miao, N., Xu, B., Bristowe, N. C., Zhou, J., and Sun, Z. (2017). Tunable Magnetism and Extraordinary Sunlight Absorbance in Indium Triphosphide Monolayer. J. Am. Chem. Soc. 139 (32), 11125–11131. doi:10.1021/jacs.7b05133
Morrison, S. R. (1987a). Mechanism of Semiconductor Gas Sensor Operation. Sensors and Actuators 11 (3), 283–287. doi:10.1016/0250-6874(87)80007-0
Morrison, S. R. (1987b). Selectivity in Semiconductor Gas Sensors. Sensors and Actuators 12 (4), 425–440. doi:10.1016/0250-6874(87)80061-6
Nazemi, H., Joseph, A., Park, J., and Emadi, A. (2019). Advanced Micro- and Nano-Gas Sensor Technology: A Review. Sensors 19 (6), 1285. doi:10.3390/s19061285
Niklasson, G. A., Berggren, L., and Larsson, A.-L. (2004). Electrochromic Tungsten Oxide: the Role of Defects. Solar Energ. Mater. Solar Cell 84 (1), 315–328. doi:10.1016/j.solmat.2004.01.045
Novoselov, K. S., Geim, A. K., Morozov, S. V., Jiang, D., Zhang, Y., Dubonos, S. V., et al. (2004). Electric Field Effect in Atomically Thin Carbon Films. Science 306 (5696), 666–669. doi:10.1126/science.1102896
Oison, V., Saadi, L., Lambert-Mauriat, C., and Hayn, R. (2011). Mechanism of CO and O3 Sensing on WO3 Surfaces: First Principle Study. Sensors Actuators B: Chem. 160 (1), 505–510. doi:10.1016/j.snb.2011.08.018
Penza, M., Martucci, C., and Cassano, G. (1998). NOx Gas Sensing Characteristics of WO3 Thin Films Activated by noble Metals (Pd, Pt, Au) Layers. Sensors Actuators B: Chem. 50 (1), 52–59. doi:10.1016/S0925-4005(98)00156-7
Qin, Y., Hu, M., and Zhang, J. (2010). Microstructure Characterization and NO2-Sensing Properties of Tungsten Oxide Nanostructures. Sensors Actuators B: Chem. 150 (1), 339–345. doi:10.1016/j.snb.2010.06.063
Roussel, P., Labbé, P., and Groult, D. (2000). Symmetry and Twins in the Monophosphate Tungsten Bronze Series (PO2)4(WO3)2m (2 ≤ M ≤ 14). Acta Crystallogr. Sect B 56 (3), 377–391. doi:10.1107/S0108768199016195
Salje, E. K. H., Rehmann, S., Pobell, F., Morris, D., Knight, K. S., Herrmannsdörfer, T., et al. (1997). Crystal Structure and Paramagnetic Behaviour of. J. Phys. Condens. Matter 9 (31), 6563–6577. doi:10.1088/0953-8984/9/31/010
Salje, E., and Viswanathan, K. (1975). Physical Properties and Phase Transitions in WO3. Acta Cryst. Sect A. 31 (3), 356–359. doi:10.1107/S0567739475000745
Seiyama, T., Kato, A., Fujiishi, K., and Nagatani, M. (1962). A New Detector for Gaseous Components Using Semiconductive Thin Films. Anal. Chem. 34 (11), 1502–1503. doi:10.1021/ac60191a001
Shi, J., Cheng, Z., Gao, L., Zhang, Y., Xu, J., and Zhao, H. (2016). Facile Synthesis of Reduced Graphene Oxide/hexagonal WO3 Nanosheets Composites with Enhanced H2S Sensing Properties. Sensors Actuators B: Chem. 230, 736–745. doi:10.1016/j.snb.2016.02.134
Sone, B. T., Nkosi, S. S., Nkosi, M. M., Coetsee-Hugo, E., Swart, H. C., and Maaza, M. (2018). Self-assembled Micro-/nanostructured WO3 Thin Films by Aqueous Chemical Growth and Their Applications in H2 and CO2 Sensing. AIP Conf. Proc. 1962 (1), 040003. doi:10.1063/1.5035541
Stankova, M., Vilanova, X., Calderer, J., Llobet, E., Ivanov, P., Gràcia, I., et al. (2004). Detection of SO2 and H2S in CO2 Stream by Means of WO3-Based Micro-hotplate Sensors. Sensors Actuators B: Chem. 102 (2), 219–225. doi:10.1016/j.snb.2004.04.030
Sun, W., Yeung, M. T., Lech, A. T., Lin, C.-W., Lee, C., Li, T., et al. (2015). High Surface Area Tunnels in Hexagonal WO3. Nano Lett. 15 (7), 4834–4838. doi:10.1021/acs.nanolett.5b02013
Szilágyi, I. M., Saukko, S., Mizsei, J., Tóth, A. L., Madarász, J., and Pokol, G. (2010). Gas Sensing Selectivity of Hexagonal and Monoclinic WO3 to H2S. Solid State. Sci. 12 (11), 1857–1860. doi:10.1016/j.solidstatesciences.2010.01.019
Szilágyi, I. M., Wang, L., Gouma, P.-I., Balázsi, C., Madarász, J., and Pokol, G. (2009). Preparation of Hexagonal WO3 from Hexagonal Ammonium Tungsten Bronze for Sensing NH3. Mater. Res. Bull. 44 (3), 505–508. doi:10.1016/j.materresbull.2008.08.003
Tian, F. H., Gong, C., Peng, Y., and Xue, X. (2017). H2 Sensing Mechanism under Different Oxygen Concentration on the Hexagonal WO3 (001) Surface: A Density Functional Theory Study. Sensors Actuators B: Chem. 244, 655–663. doi:10.1016/j.snb.2016.12.035
Tian, F. H., Gong, C., Wu, R., and Liu, Z. (2018). Oxygen Density Dominated Gas Sensing Mechanism Originated from CO Adsorption on the Hexagonal WO3 (001) Surface. Mater. Today Chem. 9, 28–33. doi:10.1016/j.mtchem.2018.04.004
Tian, F. H., Liu, Z., Tian, J., and Zhang, Y. (2020). Oxygen Vacancy O-Terminated Surface: The Most Exposed Surface of Hexagonal WO3 (001) Surface. Chin. Chem. Lett. 31 (8), 2095–2098. doi:10.1016/j.cclet.2020.01.015
Tian, F., Zhao, L., Xue, X.-Y., Shen, Y., Jia, X., Chen, S., et al. (2014). DFT Study of CO Sensing Mechanism on Hexagonal WO3 (001) Surface: The Role of Oxygen Vacancy. Appl. Surf. Sci. 311, 362–368. doi:10.1016/j.apsusc.2014.05.069
Tierney, M. J., and Kim, H. O. L. (1993). Electrochemical Gas Sensor with Extremely Fast Response Times. Anal. Chem. 65 (23), 3435–3440. doi:10.1021/ac00071a017
Trawka, M., Smulko, J., Hasse, L., Granqvist, C.-G., Annanouch, F. E., and Ionescu, R. (2016). Fluctuation Enhanced Gas Sensing with WO3-Based Nanoparticle Gas Sensors Modulated by UV Light at Selected Wavelengths. Sensors Actuators B: Chem. 234, 453–461. doi:10.1016/j.snb.2016.05.032
Vogt, T., Woodward, P. M., and Hunter, B. A. (1999). The High-Temperature Phases of WO3. J. Solid State. Chem. 144 (1), 209–215. doi:10.1006/jssc.1999.8173
Wang, L., Pfeifer, J., Balázsi, C., and Gouma, P. I. (2007). Synthesis and Sensing Properties to NH3of Hexagonal WO3 Metastable Nanopowders. Mater. Manufacturing Process. 22 (6), 773–776. doi:10.1080/10426910701385440
Wei, S., Zhao, J., Hu, B., Wu, K., Du, W., and Zhou, M. (2017). Hydrothermal Synthesis and Gas Sensing Properties of Hexagonal and Orthorhombic WO3 Nanostructures. Ceramics Int. 43 (2), 2579–2585. doi:10.1016/j.ceramint.2016.11.064
Wu, R., Tian, F., Liu, Z., Xue, X., Zhang, J., and Zu, J. (2019). CH4 Activation and Sensing on Hexagonal WO3 (001) and (110) Surfaces. Appl. Surf. Sci. 481, 1154–1159. doi:10.1016/j.apsusc.2019.03.094
Xu, Y., Tang, Z., Zhang, Z., Ji, Y., and Zhou, Z. (2008). Large-Scale Hydrothermal Synthesis of Tungsten Trioxide Nanowires and Their Gas Sensing Properties. Sen Lett. 6 (6), 938–941. doi:10.1166/sl.2008.534
Xu, Z., Vetelino, J. F., Lec, R., and Parker, D. C. (1990). Electrical Properties of Tungsten Trioxide Films. J. Vacuum Sci. Technol. A: Vacuum, Surf. Films 8 (4), 3634–3638. doi:10.1116/1.576517
Yamazoe, N., Sakai, G., and Shimanoe, K. (2003). Oxide Semiconductor Gas Sensors. Catal. Surv. Asia 7 (1), 63–75. doi:10.1023/A:1023436725457
Yang, S., Jiang, C., and Wei, S.-h. (2017). Gas Sensing in 2D Materials. Appl. Phys. Rev. 4 (2), 021304. doi:10.1063/1.4983310
Yang, W., Gan, L., Li, H., and Zhai, T. (2016). Two-dimensional Layered Nanomaterials for Gas-Sensing Applications. Inorg. Chem. Front. 3 (4), 433–451. doi:10.1039/C5QI00251F
Zee, F., and Judy, J. W. (2001). Micromachined Polymer-Based Chemical Gas Sensor Array. Sensors Actuators B: Chem. 72 (2), 120–128. doi:10.1016/S0925-4005(00)00638-9
Zhang, C., Debliquy, M., Boudiba, A., Liao, H., and Coddet, C. (2010). Sensing Properties of Atmospheric Plasma-Sprayed WO3 Coating for Sub-ppm NO2 Detection. Sensors Actuators B: Chem. 144 (1), 280–288. doi:10.1016/j.snb.2009.11.006
Zhang, H. (2015). Ultrathin Two-Dimensional Nanomaterials. ACS Nano 9 (10), 9451–9469. doi:10.1021/acsnano.5b05040
Zhang, W.-H., Ding, S.-J., Zhang, Q.-S., Yi, H., Liu, Z.-X., Shi, M.-L., et al. (2021). Rare Earth Element-Doped Porous In2O3 Nanosheets for Enhanced Gas-Sensing Performance. Rare Met. 40 (6), 1662–1668. doi:10.1007/s12598-020-01607-x
Zhang, Y., Zeng, W., and Li, Y. (2019). NO2 and H2 Sensing Properties for Urchin-like Hexagonal WO3 Based on Experimental and First-Principle Investigations. Ceramics Int. 45 (5), 6043–6050. doi:10.1016/j.ceramint.2018.12.075
Keywords: 2D, hexagonal WO3, gas sensing, oxygen vacancy, metal oxide semiconductor
Citation: Li Y, Zhou Q, Ding S and Wu Z (2021) Research Progress of Gas Sensing Performance of 2D Hexagonal WO3. Front. Chem. 9:786607. doi: 10.3389/fchem.2021.786607
Received: 01 October 2021; Accepted: 08 November 2021;
Published: 06 December 2021.
Edited by:
Zhaofu Zhang, University of Cambridge, United KingdomReviewed by:
Nan Yang, Xingtai University, ChinaHao Luo, Southwest University of Science and Technology, China
Xiaochuan Duan, Taiyuan University of Technology, China
Qingkai Qian, Chongqing University, China
Copyright © 2021 Li, Zhou, Ding and Wu. This is an open-access article distributed under the terms of the Creative Commons Attribution License (CC BY). The use, distribution or reproduction in other forums is permitted, provided the original author(s) and the copyright owner(s) are credited and that the original publication in this journal is cited, in accordance with accepted academic practice. No use, distribution or reproduction is permitted which does not comply with these terms.
*Correspondence: Shoubing Ding, c2hvdWJpbmdkaW5nQGNxbnUuZWR1LmNu; Zhimin Wu, em13dUBjcW51LmVkdS5jbg==