- School of Chemistry and Chemical Engineering, Guangxi University, Nanning, China
Abstract: The synthesis costs of macrolide musks are higher than those of other commercial musks. To make this process less expensive, eggshell waste was calcined at a low temperature to obtain a catalyst for the cyclopentadecanolide production via reactive distillation using a glycerol entrainer. X-ray diffraction, Fourier-transform infrared spectroscopy, scanning electron microscopy, transmission electron microscopy, and X-ray photoelectron spectroscopy analyses of the original and recovered catalysts revealed that the main catalytic ingredient was calcium glycerolate (CaG) and not calcium diglyceroxide (CaDG). The basic strengths of CaG and CaDG obtained by Hammett indicators were 7.2 < H_≤ 15.0 and 9.8 < H_≤15.0, while the corresponding base amounts were 1.9 and 7.3 mmol/ g, respectively. Because CaG was soluble in glycerine, the catalyst was efficiently reused. The reaction product containing over 95.0% cyclopentadecanolide with a yield of 49.8% was obtained at a temperature of 190°C and catalyst amount of 12 wt% after 7 h of reaction. Thus, eggshell waste may be directly placed into the reaction mixture after calcination at 600°C to synthesise a large amount of cyclopentadecanolide within a relatively short time. The results of this work indicate that eggshell waste can serve as a potential eco-friendly and affordable catalyst source for the production of macrolide musks.
Introduction
Eggs are consumed worldwide due to their high nutrition value. Eggshells constitute approximately one-tenth of the egg mass (Christmas and Harms, 1976) and are typically discarded as kitchen or solid waste without pretreatment (Kamkum et al., 2015). In China, several million tonnes of eggshells are generated annually, and this amount will continue to increase in the future. During the eggshell degradation process, hydrogen sulphide and other toxic gases toxic are generated to become a source of organic pollution (Oliveira et al., 2013). Hence, it is not desirable to landfill eggshell waste directly due to environmental concerns. Instead, eggshell waste can be potentially reused to eliminate the environmental threat and produce a high economic value (Xu et al., 2019; Mohazzab et al., 2020; Punj and Singh, 2020).
Eggshells comprise a network of protein fibres, in which calcium carbonate accounts for 96% of the shell weight, the contents of calcium phosphate and magnesium carbonate are 1% each, and the remaining part consists of organic matter and water. Because limestone is a non-renewable natural resource, using renewable eggshell waste as an alternative source of calcium carbonate can reduce the negative impact on the natural reserves of limestone. Calcium carbonate may be converted into calcium oxide by calcination, and the calcium oxide prepared from eggshell waste can potentially replace more expensive commercial calcium oxide reagents. The pores of eggshells may be used as catalyst carriers (Nasrollahzadeh et al., 2016), and the calcium oxide obtained after calcination represents a high-quality base catalyst (Verziu et al., 2011; Marinković et al., 2016). Therefore, developing eggshell catalysts provides a reliable direction for the effective use of eggshells waste. Eggshell-derived catalysts have been successfully applied in biodiesel production (Joshi et al., 2015; Yusuff et al., 2018; Kirubakaran and Arul Mozhi Selvan, 2018; Marwaha et al., 2018; Rahman et al., 2019). Wei et al. (Wei et al., 2009) obtained a highly active reusable solid catalyst by calcining eggshells and utilised it to convert soybean oil into biodiesel. When the calcination temperature of eggshells was below 600°C, the biodiesel yield was less than 30%, and its value was increased by raising the calcination temperature to 1,000°C. Similarly, eggshells and chicken fat constitute poultry wastes that can be valorised as cheap sources of the catalyst and oil for the production of biodiesel, respectively. Heterogeneous catalysts were successfully prepared by calcining eggshells, and the maximum biodiesel yield of 90.2% was achieved at a calcination temperature of 1,000°C (Odetoye et al., 2021).
In addition to catalysing the synthesis of biodiesel, eggshell-derived calcium oxide has also been employed in other catalytic reactions. (Gao and Xu, 2012) utilised eggshell waste as a raw material to prepare a catalyst for the synthesis of dimethyl carbonate (DMC). Eggshells were calcined in a muffle furnace at 1,000°C for 2 h under static air prior to the reaction. The obtained DMC yield was as high as 75%. Taufiq-Yap et al. (Taufiq-Yap et al., 2014) studied the possibility of hydrogen production via wood gasification facilitated by an eggshell-based catalyst. The biomass gasification of Azadirachta excelsa wood was performed using a CaO catalyst derived from the eggshells calcined at 900°C for 2 h. As a result, the hydrogen production was increased to a maximum value of 73%. Khazaei et al. (Khazaei A. et al., 2017; Khazaei et al., 2017b) employed a CaO catalyst derived from eggshells as a natural solid base for the Suzuki coupling reaction conducted in the presence of rice husk-supported Fe3O4 that stabilised palladium magnetic nanoparticles. The eggshell calcination temperature in that study was 900°C. A CaO nanocatalyst prepared from hen eggshells was used for the production of pyrano [4,3-b]pyrans. Eggshell powder was calcined at 900°C for 1 h, and the obtained product exhibited high catalytic activity (Mosaddegh and Hassankhani, 2014). In addition, eggshell waste can be reused multiple times in various catalytic processes, demonstrating very high stability. In the production of biodiesel and other industrial compounds, not only high yield and high value-added products were obtained, but the use value of eggshell waste was also improved, which allowed the successful reuse of this waste, decreased the production costs, and reduced environmental pollution.
Eggshell-derived calcium oxide is utilised as a catalyst, and its active ingredients depend on a particular catalytic system (Kouzu et al., 2008; Ebadi Pour et al., 2021). Specifically, when calcium oxide is extracted from eggshells as a catalyst to produce biodiesel, the obtained product contains not only calcium oxide, but other components as well. Furthermore, calcium oxide is easily converted into calcium glyceroxide (CaDG) through its reaction with glycerol. Similar to calcium oxide, CaDG effectively catalyses the transesterification of vegetable oil (Kouzu et al., 2010). Gupta’s group used eggshell waste to derive two catalysts: Eggshell–CaOC–H–D and Eggshell–CaDG, which were subsequently used for the transesterification of waste cooking oil The obtained biodiesel yields were 96.07 and 93.10%, respectively (Gupta and Rathod, 2018b). Therefore, further research is required for the catalytic utilisation of calcium oxide derived from eggshells. In general, eggshells are utilised in many fields, and their catalytic efficiency is very high. However, when calcium oxide derived from eggshells is used as a catalyst, the calcination temperature of eggshells in the above-mentioned reactions reached 900 °C and even exceeded 1,000°C in some cases, which resulted in very large energy consumption. Thus, our objective was to identify a synthetic reaction catalysed by a solid base which was obtained by the low-temperature calcination of eggshells. To the best of our knowledge, no studies on the catalytic synthesis of macrocyclic musks using eggshell derivatives have been performed previously.
The very special olfactory feeling exerted by musk or ‘muskiness’ is generally described as a unique blend of softness, gentle warmness, and sensuality with a skin-like impression (David, 2020). A well-known and best-selling class of fragrance compounds includes macrocyclic lactones and ketones known for centuries as musks (Sytniczuk et al., 2018). In the past, musks were obtained from fauna and flora leading to a severe decrease in their population and bringing many species to the verge of extinction. Therefore, people have turned their attention to synthetic musks, leading to significant achievements in this field (Nagarajan et al., 1999; Zviely, 2009; Ohloff and Pickenhagen, 2012; Parenty et al., 2013; Li et al., 2017; Zhang et al., 2018). These synthetic musks are widely used in many personal care products, including soaps, shampoos, detergents, and deodorants as well as in scented products such as air fresheners and scented candles (Belsito et al., 2011). Other musk applications include antibiotics (Jelić and Antolović, 2016), anti-cancer drugs (Luesch et al., 2002; Wright et al., 2007; Fuwa et al., 2011), food additives, and insecticides (Kirst, 2010; Bai et al., 2016). In 2017, the estimated market for flavours and fragrances was worth 24.8 billion dollars (International Flavors and Fragrances Inc., 2017). Macrocyclic musks have attracted increasing attention from the perfume industry (De Léséleuc et al., 2015) as they do not exhibit toxicity or bioaccumulation properties associated with the traditional nitro-aromatic and polycyclic musks (Zviely, 2009). In addition, they are environmentally friendly, have renewable alternatives, and possess good sustainability properties (Morin et al., 2019). The market demand for macrocyclic musks is rapidly growing (Matsuda et al., 2007; Boethling, 2011). Synthetic macrolactones, which represent an alternative to those found in natural sources (Blunt et al., 2009), have also attracted considerable attention from researchers (Morales-Serna et al., 2010). Cyclopentadecanolide is a semi-volatile cyclic organic chemical, which belongs to the class of macrolide musks. An important strategy for the synthesis of macrolide musks is the macrolactonisation of seco acid, which has been performed in a large number of synthetic processes. In 1998, the lactonisation of 15-hydroxypentadecanoic acid to cyclopentadecanolide was successfully conducted on dealuminated HY zeolite (Ookoshi and Onaka, 1998). The macrolactonization reaction utilises m-xylene as a solvent to obtain a 51% yield of cyclopentadecanol after 24 h reflux at a very low raw material concentration. Myléne de Léséleuc et al. (De Léséleuc and Collins, 2015) used Hf(OTf)4 to directly catalyse the macrolactonisation of seco acid via a ‘one-pot’ reaction. However, this method has several inherent limitations. For example, either the acid or hydroxyl groups must be activated by special reagents. In addition, to minimise intermolecular dimerisation, highly diluted conditions or slow addition protocols are required.
Zhang et al.(Zhang et al., 2018) used Rh(I)/Yb(III) to catalyse the macrolactonisation of alkynyl alcohol and provide a strategically distinct entry for macrolactones. In addition to the operational simplicity, this process can be performed at relatively high concentrations. Although many catalysed macrolactonisation reactions were conducted in the past (Gonthier and Breinbauer, 2005; Kopp et al., 2012; Parenty et al., 2013; Li et al., 2017; Xie et al., 2018), their practical implementation remains challenging due to the complex catalyst preparation process, large macrolactonisation time, high production costs, and the difficulty of obtaining intermediates. Furthermore, the reaction product must undergo complex separation and purification processes requiring large amounts of organic solvents, and the final macrolactone yield is very low. Hence, the synthesis of macrocyclic musk compounds is a difficult and, in many cases, multi-step procedure.
In this work, we studied the catalytic properties of an eggshell-derived catalyst for the macrolactonisation of methyl 15-hydroxypentadecanoate to cyclopentadecanolide using a reactive distillation method. Because both the reactant and the product are insoluble in glycerol, the latter is employed as an entrainer during the distillation of the raw materials for the synthesis of catalytically active compounds. The obtained catalyst was characterised by X-ray diffraction (XRD), thermogravimetric analysis (TGA), Fourier-transform infrared (FT–IR) spectroscopy, and scanning electron microscopy (SEM). The yields of the produced cyclopentadecanolide were determined by gas chromatography (GC) and optimised under various operating conditions, including calcination temperature, reaction temperature, reaction time, and catalyst amount. As a result, eggshell waste was found to be an economical and eco-friendly catalyst for the synthesis of macrocyclic musks.
Materials and Methods
Chemicals
Chicken eggshells were collected from a canteen at Guangxi University located in Nanning City, China. Diethyl ether, methanol, ethanol, sulfuric acid, n-hexane, bromothymol blue, phenolphthalein, 2,4,6-trinitroaniline, 4–nitroaniline, and glycerine was purchased from Guangdong Guanghua Sci-Tech Co., Ltd. (Guangdong, China). Cyclopentadecanolide (>98%) was obtained from Sigma-Aldrich Co., and 2-amino-2-methyl-1-propanol and calcium oxide were purchased from Shanghai Aladdin Biochemical Technology Co., Ltd. (Shanghai, China). All materials were of analytically pure grade. Malania Oleifera Chum oil was obtained from Yandong Township, Bama County, Guangxi Province, China.
Catalyst Preparation
The collected eggshells were rinsed with distilled water to remove impurities. The washed eggshells were dried overnight at 110°C and then crushed into powder using a mortar, which was subsequently calcined in a muffle furnace for 4 h. Before cooling to room temperature, the calcined sample was transferred into a sealed glass container.
CaDG was prepared as described elsewhere (Kouzu et al., 2010; León-Reina et al., 2013; Gupta et al., 2015; Esipovich et al., 2018). Briefly, 0.5 g of CaO was directly placed into a flask containing 35 ml of glycerol and 100 ml of methanol. The obtained mixture was stirred at a speed of 600 rpm and temperature of 80°C for 10 h. The obtained precipitate was separated by centrifugation and washed with methanol until the unreacted glycerol was completely removed and dried at 95°C for 2 h.
CaG synthesis was performed according to the method developed by the Taylor group (Whitehouse, 1992). In this process, 3 g of calcium hydroxide was added to 30 g of glycerol, and the obtained mixture was heated to 180°C for 4 h. After cooling to room temperature, the solids were washed with 40 ml of anhydrous ethanol solution and centrifuged at 3,000 rpm. After repeated washing, the collected solids were dried overnight in vacuum at 60°C.
Catalytic Test
Synthesis of Methyl 15-Hydroxypentadecanoate
15-Tetracosenoic acid was obtained from Malania Oleifera Chum oil after saponification, acidification, and solvent crystallisation. 15-Tetracosenoic acid (30.0 g) was dissolved in a n-hexane/methanol(4:1, 200 ml) mixture. The entire reactor assembly was immersed into a thermostatic water bath with a constant temperature of 0°C. Ozone gas generated by a laboratory-scale corona discharge generator was continuously bubbled through the reactor until reaching a turning point, at which potassium iodide starch paper turned blue. Potassium borohydride solution which was prepared by dissolving 0.2 g NaOH and 5.5 g KBH4 in 180 ml of deionised water was slowly dropped into the obtained ozonide intermediate at a temperature between 15 and 18°C and continuously stirred for 3 h. The pH value of the produced alkali liquor was adjusted to 2 by adding a 6 mol/L hydrochloric acid solution. The mixture was decompression filtered, and the 15-hydroxypentadecanoic acid filter cake was washed to the neutral pH by distilled water and dried at 70°C for 8 h under vacuum to remove water and other solvents.
The obtained 15-hydroxypentadecanoic acid was purified by forming an amine compound with 2-amino-2-methyl-1-propanol. After that, methyl 15-hydroxypentadecanoate was produced by methyl esterification. In this process, 15 g of 15-hydroxypentadecanoic acid was dissolved in 300 ml of methanol followed by the slow addition of 2 ml concentrated sulfuric acid and refluxing at 90°C for 4 h. After the reaction was complete, the product was extracted three times with diethyl ether and washed with water. The solvent was removed by rotary evaporation and concentrated to obtain methyl 15-hydroxypentadecanoate with a mass content of more than 70%.
Synthesis of Cyclopentadecanolide
Cyclopentadecanolide (2) was synthesised as follows. First, 0.5 g of the catalyst and 5 mmol of methyl 15-hydroxypentadecanoate (1) were mixed with 15 ml of glycerine solution and stirred at 120°C for 30 min until methyl 15-hydroxypentadecanoate was completely dissolved. Afterwards, the solvent and cyclopentadecanolide were distilled out from the reaction system at 190°C in vacuum (2 mbar), and the solvent was returned to the reaction medium. At the end of the reaction, the system was cooled to room temperature (25°C). The produced cyclopentadecanolide crystals were taken out and dissolved in 10 ml of ether and washed three times with 50 ml of deionised water. The upper solution layer was separated, and the solvent and water were removed under vacuum at 60°C. Finally, a white lumpish solid (cyclopentadecanolide) was obtained.
Catalyst Characterisation
XRD patterns of the produced samples were recorded on a Rigaku Ultima IV diffractometer with Cu Kalpha radiation in the 2θ range of 5–80°.
TGA and derivative thermogravimetry (DTG) studies were performed using a TA Instruments Q500 analyser at a heating rate of 10°C min−1 in the temperature region from 30 to 1,000°C under a nitrogen atmosphere.
XPS measurements were conducted on a Thermo Scientific K-Alpha spectrophotometer.
Morphologies and chemical compositions of the obtained catalysts were examined using a scanning electron microscope equipped with an energy dispersive X-ray analysis (EDX) module (Zeiss Sigma 300, SmartEDX).
FT–IR spectra were recorded on a Nicolet iS50 spectrometer in the wavenumber range of 4,000–800 cm−1 using KBr pellets.
Hammett indicator (dissolved in ethanol) was utilised to determine the basic strengths of the catalysts (Rahman et al., 2019). Briefly, 0.1 g of the analysed sample was shaken with 10 ml of moisture-free ethanolic solution of the Hammett indicator and left to equilibrate for 2 h. The indicators used were bromothymol blue (pKa = 7.2), phenolphthalein (pKa = 9.8), 2,4,6-trinitroaniline (pKa = 12.2), 2,4–dinitroaniline (pKa = 15.0), and 4–nitroaniline (pKa = 18.4). Afterwards, 2–3 drops of the Hammett indicator solution were added to an ethanolic solution of the sample with gentle shaking and then left to be equilibrated for colour variation. If the indicator exhibited a colour change, the basic strength of the catalyst was considered to be higher than that of the indicator; otherwise, the catalyst basic strength was lower than that of the indicator. Catalyst basicity was assessed by measuring the amount of the Hammett indicator–benzene carboxylic acid solution required for its neutralisation (Yan et al., 2009).
Product Analysis
GC analyses of the synthesised methyl 15-hydroxypentadecanoate and cyclopentadecanolide products were performed on a Shimadzu GC-2010 Plus gas chromatograph. Their yields were determined via the following formula:
Results and Discussion
Catalytic Performance
XRD Analysis of Eggshell Waste at Different Calcination Temperatures
The XRD patterns of the eggshells calcined at different temperatures are shown in Figure 1. The untreated eggshell spectrum contains a weak CaCO3 (PDF#47-1743) diffraction peak. In contrast, the XRD patterns of the eggshells calcined at 500 and 550 °C had very intense and sharp CaCO3 peaks centred at 23.06°, 29.40°, 35.97°, 39.41°, 43.16°, 47.50°, and 48.50° (PDF#72-1214), owing to the removal of organic impurities from the eggshell surface. When the calcination temperature exceeds 600 °C, the eggshells exhibit pronounced CaO diffraction peaks at 32.19°, 37.34°, 53.84°, 64.13°, and 67.36° (PDF#77-2010). As the calcination temperature further increases, the CaO diffraction peak gradually increases, and the CaCO3 diffraction peak gradually decreases until it completely disappears at 900°C because the CaCO3 phase in the eggshell has been fully converted into CaO. Therefore, the minimum temperature at which eggshell waste is converted into calcium oxide is 600°C.
TG/DTG Analysis of Eggshell Waste
The calcination process of waste eggshell powder was studied by TG/DTG, and the obtained results are shown in Figure 2. The eggshell powder was heated from 30 to 1,000 °C at a rate of 10°C min−1 under a nitrogen atmosphere. The quality reduction of eggshell powder observed at 30–500°C was mainly caused by the decomposition of organic matter and moisture removal from the eggshells. The quality of eggshell powder at 600–800°C was significantly reduced due to the removal of carbon dioxide. However, the eggshell quality changed very little after 850 °C, indicating that CaCO3 was completely decomposed into CaO at this temperature. From the results of TG/DTG analysis, it can be concluded that the temperature at which CaO starts to produce and CaCO3 begins to decompose is 600 °C consistent with the XRD data. Therefore, considering the preparation temperature and energy consumption, the CaO catalyst can be obtained from waste eggshells by roasting them at 600 °C. Similar results were obtained for waste eggshells by Joshi et al.(Joshi et al., 2015).
FT–IR and XRD Analyses of Different Catalysts
The FT–IR spectra recorded for fresh CaO derived from waste eggshells at 600 °C, Ca(OH)2, CaDG, CaG, CaG-E (used catalyst obtained from CaO derived from eggshells), and glycerol are presented in Figure 3. All samples are characterised by a broad band between 3,600 and 3,000 cm−1 which can be ascribed to O–H stretching vibrations. In addition, the FTIR spectra of fresh CaO exhibit O–H stretching vibrations, which were likely caused by the absorbed water from air. The bands at 2,925, 2,865, and 2,826 cm−1 in the CaDG, CaG, CaG-E, and glycerol spectra are related to C–H stretching vibrations. Similarly, various C–H bending modes (1,436, 1,228, and 921 cm−1), C–O–H bending modes (1,311 cm−1), and C–O stretching modes (1,104 and 1,050 cm−1) were observed. The band between 1,300 and 1700 cm−1 corresponds to the O–C–O stretching vibrations of CO32− species. The FT–IR spectra of CaDG, CaG, and glycerol are in good agreement with the results reported by Reyero et al.(Reyero et al., 2014) and León-Reina et al.(León-Reina et al., 2013). They show that CaDG and CaG have been successfully synthesised and used in the cyclopentadecanolide production. Note that the FT–IR spectrum of CaG-E is similar to those CaDG and CaG and exhibits a high O–C–O stretching vibration intensity, owing to the loss of CaG from the solid catalyst surface and exposure of CaCO3 species.
The XRD patterns of fresh CaO, Ca(OH)2 (2θ = 18.01°, 28.67°, 34.10°, 47.12°, 50.81°, PDF#44-1,481), CaDG (2θ = 8.18°, 10.09°, 21.19°, 24.30°, 26.59°, 34.37°, PDF#21-1,544), CaG (2θ = 10.13°, 22.08°, 29.56°, 32.62°, 33.92°, 37.84°, 45.06°), and CaG-E are presented in Figure 4. The CaG-E spectrum contains characteristic diffraction peaks of CaG (2θ = 10.13°, 22.08°, 32.62°, 33.92°, 37.84°, 45.06°, Taylor et al. (Whitehouse, 1992)) and Ca(OH)2 (2θ = 18.01°, 34.10°) with lower intensities, suggesting that CaG and Ca(OH)2 were formed in the reaction of CaO with glycerol at high temperatures, which was consistent with the results obtained by Pour et al. (Pour et al., 2021). Moreover, colloidal Ca(OH)2 was formed due to the presence of water in the reaction medium (Ruppert et al., 2008), and when CaO and Ca(OH)2 were converted in situ to solid CaG, an equilibrium was established between CaG and colloidal Ca(OH)2 species. The diffraction peak of CaCO3 (2θ = 29.18°) in the CaG-E spectrum is more pronounced, which is related to the loss of surface Ca(OH)2 species during the washing recovery process. Hence, CaG is the active component of the produced catalyst, which is in good agreement with the FTIR data.
SEM and EDX Analyses of Different Catalysts
The morphologies of the fresh CaO, CaDG, CaG, and CaG-E catalysts were analysed by SEM (Figure 5). The fresh CaO surface contains irregularly shaped particles with considerable gaps and relatively large pores, which are related to the eggshell hollow structure. The enlarged image indicates the presence of cracks on the particle surface caused by the overflow of CO2 gas during the calcination process. The SEM image of CaDG (Figure 5B) shows the particles with sizes ranging from 7 to 10 μm and angular rock shapes, which is consistent with the results of previous studies conducted by Gupta et al.(Gupta and Rathod, 2018a) and Sánchez-Cantú et al.(Sánchez-Cantú et al., 2014). Figure 5C displays the SEM image of the synthesised CaG surface, which exhibits a single layer of irregular sheet structures. In Figure 5D, a large number of irregular lamellar structures (similar to CaG particles) are stacked on the CaG-E surface. This increases its specific surface area and facilitates the dissolution of CaG in glycerol during reuse; hence, CaG can be utilised as a homogeneous catalyst in the macrolactonisation process. In addition, EDX analysis was performed to determine the CaG-E composition (Figure 5E). The obtained spectrum shows that the analysed area contains 13 wt% carbon, 32 wt% oxygen, and 55 wt% calcium.
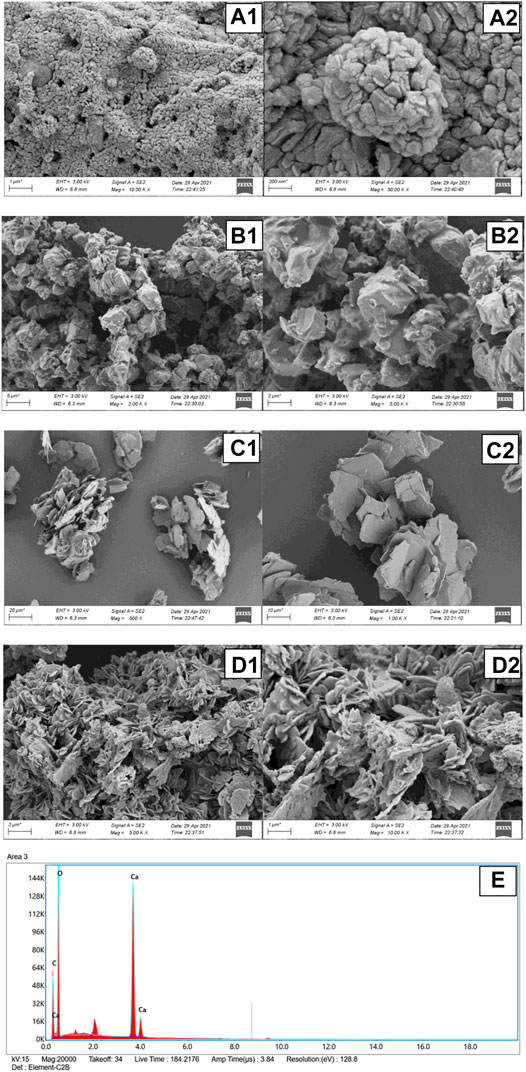
FIGURE 5. SEM images of the (A) fresh CaO, (B) CaDG, (C) CaG, and (D) CaG-E samples. (E) EDX profile of the CaG-E catalyst.
TEM and XPS Analyses of the CaG-E Catalyst
The TEM images of the CaG-E sample are presented in Figure 6. Figure 6A shows the transparent edge with several black spots in the middle. The edge can be attributed to the CaG phase, and the black spots likely consist of partially calcined CaCO3 species. After increasing the TEM magnification, CaG lattice fringes were observed (Figure 6B). Therefore, CaG-E is composed of CaG and CaG-wrapped CaCO3 species.
The chemical compositions of the CaG-E, CaG, and fresh CaO samples were also determined by XPS. The survey scan spectrum contains three main peaks with binding energies corresponding to C 1s, Ca 2p, and O 1s species (Figure 7A). The C 1s core level spectrum exhibits two broad peaks consisting of the C–C (284.4 eV), C–O (286.1 eV), and O–C=O (288.8 eV) components. Compared with fresh CaO, both the CaG-E and CaG samples exhibit C–O peaks, and the intensities of the C–C peaks of the CaG-E and CaG samples are significantly larger than those of the other catalysts (Table 1), indicating the formation of a new compound on the CaG-E surface. The Ca 2p region exhibits two symmetric bands at 346.5 and 350.1 eV corresponding to a typical doublet of the Ca 2p3/2 and Ca 2p1/2 components with a separation of 3.6 eV. The Ca 2p3/2 peak has lower binding energies than those of CaO/calcite (347.1–347.7 eV), which suggests weaker interactions between Ca2+ and glyceroxide ions. The XPS profile of the CaG-E surface (Figure 7, CaG-E) shows a broad O 1s peak that can be deconvoluted into two components centred at 531.8 and 532.6 eV with areas of 37.7 and 10.9% (Table 1), which are attributed to OH− and O− groups, respectively (León-Reina et al., 2013). In Figure 6, the CaG-E and CaG peals are matched very well, suggesting that CaG is composed of glyceroxide anions, Ca2+ and OH−, and its likely chemical formula is HOCH2CHOHCH2OCaOH, which is consistent with the XRD spectra presented in Figure 4.
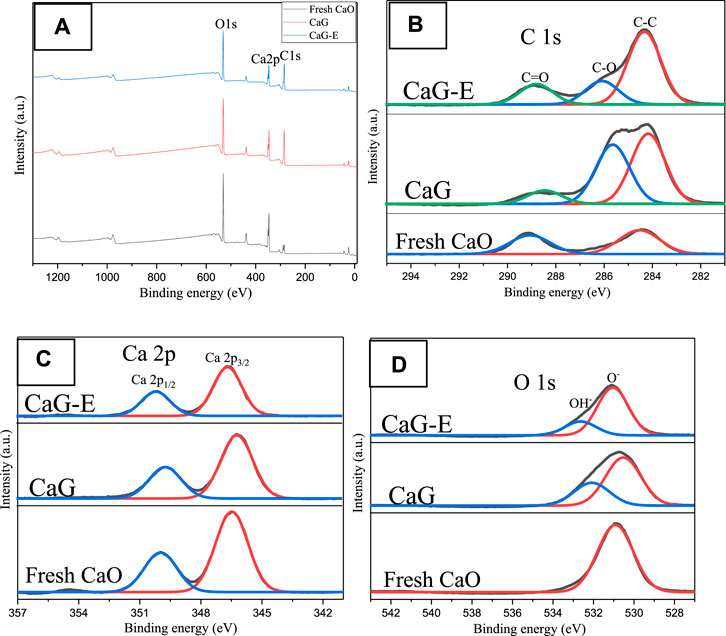
FIGURE 7. XPS (A) survey spectra of the CaG-E, CaG, and fresh CaO samples, and the corresponding core-level (B) C 1s, (C) Ca 2p, and (D) O 1s spectra.
Basicity and Basic Strength Measurements Conducted by the Hammett Method
The basicity and basic strength of a catalyst play a vital role in the macrolactonisation reaction. The basic strengths of the catalysts prepared in this work were determined by the Hammett indicator method (Table 2). The basic strengths of CaDG and CaG were 9.8 < H_ ≤ 15.0 and 7.2 < H_ ≤ 15.0, respectively. An aqueous solution of CaG was used to measure its pH value equal to 13.0. The basic amounts of CaDG and CaG were 7.3 and 1.9 mmol g−1, respectively. The basicity and basic strength of CaG were lower than those of CaDG; however, the former compound produced a stronger catalytic effect because the high basicity of CaDG promoted the saponification of raw materials. Furthermore, the relatively low basicity of CaG increased it resistance to poisoning by ambient CO2 and H2O species and storage stability.
Proposed Macrolactonisation Mechanism
The proposed macrolactonisation mechanism of methyl 15-hydroxypentadecanoate by CaG is presented in Scheme 1. In Here, CaG activates the carbon–oxygen bond of the terminal hydroxyl group and ester group of methyl 15-hydroxypentadecanoate. HOCH2CHOHCH2OCaδ+ and OHδ− are the two active catalytic sites participating in the reaction. In step (A), ester and alcohol are adsorbed on these two neighbouring free catalytic sites. The adsorbed ester had formed an intermediate, while OHδ− had extracted Hδ+ and HOCH2CHOHCH2OCaδ+ adsorbed–O–CH2– from alcohol. By decreasing their electron density through the coordination with Ca atoms, Cδ+ ions can undergo a nucleophilic attack by Oδ− anions. These two neighbouring adsorbed species react with each other in steps (B) and (C), leading to the formation of cyclopentadecanolide and methanol.
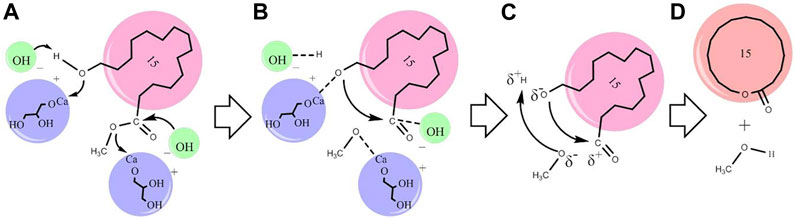
SCHEME 1. Proposed mechanism for the macrolactonisation of methyl 15-hydroxypentadecanoate using the CaG catalyst (A–D).
Catalytic Activity
Effect of Catalyst Type on the Product Yield
The catalytic effects of different catalysts on the macrolactonisation of methyl 15-Hydroxypentadecanoate are compared in Figure 8. The yield of cyclopentadecanolide is zero when no catalyst is added to the reaction mixture. Fresh CaO demonstrates a higher catalytic activity than those of eggshells, Ca(OH)2, and CaDG, while the catalytic activities of CaG and fresh CaO are very close. Finally, the catalytic effect of fresh CaO is slightly weaker than that of commercial CaO because eggshells contain small amounts of impurities such as magnesium carbonate in addition to CaCO3. After calcination, these impurities promote oxide formation and thus reduce the catalytic activity.
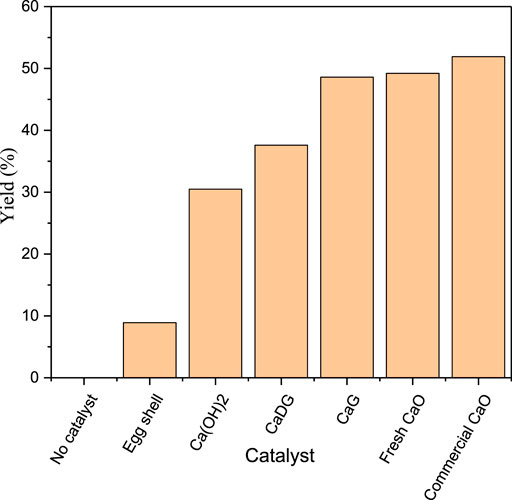
FIGURE 8. Effects of various catalysts for the macrolactonization of methyl 15-hydroxypentadecanoate on the product yield.
Effect of Reaction Conditions on the Product Yield
In this work, we optimised various process parameters, including the calcination temperatures of eggshells (500–900°C), catalyst amount (4–20 wt%), reaction time (3–8 h), and reaction temperature (170–210°C). The obtained results are shown in Figure 9. Note that to distil glycerol, the reaction must be performed under a vacuum of 2 mbar.
First, the effect of the calcination temperature of eggshells on the product yield was investigated. When the temperature is lower than 600°C, the cyclopentadecanolide yield cyclopentadecanolide is only 6.1%. Because the calcination temperature is too low, the CaCO3 phase in the eggshells is not decomposed into CaO. After the organic matter is carbonised, it forms a black solid layer on the catalyst surface, which ultimately deteriorates the catalytic effect. The yield rapidly increases to 49.8% at a roasting temperature of 600°C; however, the cyclopentadecanolide yield decreases with an increase in calcination temperature because the higher temperatures promote the CaCO3 conversion into CaO, increasing the CaO content. The glycerol-containing system produces a larger amount of colloidal Ca(OH)2, which inhibits the dissolution and diffusion of CaG species into glycerol and decreases the product yield. This trend is similar to that produced by the catalyst amount. When the amount of catalyst exceeds 12 wt%, the yield of cyclopentadecanolide decreases for the same reason.
Next, the effect of reaction time on the cyclopentadecanolide yield was investigated. Overall, the yield increases gradually with time. However, it rises very slowly when the reaction time exceeds 7 h, owing to the insufficiently high driving force for the reaction caused by the lower amounts of the raw materials. The best result was obtained at 7 h (49.8% yield) because only an additional 4.4% yield was achieved after the reaction time was increased by 2 h.
Finally, the impact of reaction temperature on the product yield was studied. As the reaction temperature increases, the yield first increases and then decreases after reaching 200°C. Because organic compounds are easily carbonised at high temperatures to increase the concentration of by-products, the saponification of the reactants in a strongly alkaline environment is likely to produce the corresponding salts. Based on these findings, 190°C was selected as the optimal reaction temperature. The related GC data indicated that the content of cyclopentadecanolide obtained in all reactions exceeded 95.0%.
Performance of Recycled Catalysts
After the reaction was complete, the catalyst was separated by filtering, washed with ethanol, and dried under vacuum at 60°C overnight for the subsequent reuse and analysis (Figure 10). Interestingly, after the first reuse of fresh CaO, the yield of cyclopentadecanolide increased due to the formation of colloidal Ca(OH)2 on the catalyst surface after the first reaction with a large amount of wrapped CaG species. During the washing step conducted after recovering the catalyst from the first reaction medium, the colloidal forms of Ca(OH)2 were washed out, and a large amount of CaG was exposed to the surface, which was consistent with the SEM and TEM data. In addition, a large CaG amount was dissolved in glycerol to serve as a homogeneous basic catalyst; as a result, the product yield during the first repeated use was higher than that obtained for CaO. After the first repeated use of the catalyst, the yield dropped significantly due to the large loss of CaG. In the XRD pattern of the second recovered catalyst, the diffraction peak of CaG is significantly smaller, and the quality of the recovered catalyst is much lower than those of the fresh catalyst. After three repeated experiments, the cyclopentadecanolide yield was reduced to 2.1%.
Proposed Mechanism of CaG Formation and Lixiviation
From the obtained XRD results, a plausible CaG formation and lixiviation mechanism was proposed (Scheme 2). The fresh CaO catalyst is mainly composed of CaO, Ca(OH)2, and CaCO3 species (A). After fresh CaO enters the reaction, Ca(OH)2 colloids are preferentially formed. These colloids react with glycerol to produce CaG in situ (B), and CaCO3 nuclei are retained in the centres of catalyst particles. After the first washing and recovery step, CaG is exposed to the catalyst surface (C). When the catalyst is recycled for the second time, CaG is dissolved into glycerol, leaving mostly CaCO3 cores (D).
Conclusion
In this study, the CaO catalyst prepared by calcining eggshell waste at a low temperature was used to catalyse the macrolactonisation of methyl 15-hydroxypentadecanoate. The characteristic changes in the catalyst morphology and composition after the reaction were characterised by XRD, SEM, TEM, and XPS, indicating that the main catalytically active component was CaG with the likely chemical formula HOCH2CHOHCH2OCaOH. Fresh CaO reacted with glycerine to form CaG, which acted as a homogeneous catalyst in the macrolactonisation reaction. The CaG-E catalyst was composed of CaG and CaCO3 species. The optimal macrolactonisation conditions determined by conducting single-factor experiments corresponded to the eggshell roasting temperature of 600°C, reaction temperature of 190°C, reaction time of 7 h, and catalyst amount of 12 wt%. Note that the utilised calcination temperature was only 600°C, which considerably decreased the energy consumption as compared with those of waste eggshell processes performed in other studies. Hence, the proposed catalyst can be obtained in large quantities by simply calcining eggshells in air at low temperatures. Its preparation method is simple, the reaction time is relatively short, and the product yield is high. The synthesised cyclopentadecanolactone is separated by reactive distillation. Because it is not soluble in glycerol, the obtained product does not require further purification, and its purity can exceed 95.0%. The method for the catalyst preparation from eggshell waste effectively recycles the waste, reduces the catalyst manufacturing cost, minimises the amount of contaminants, and makes the catalyst environmentally friendly. We used 15-tetracosenoic acid as a raw material, which may limit its industrial application. Therefore, finding other methods to synthesize methyl 15-hydroxypentadecanoate is our next work.
Data Availability Statement
The original contributions presented in the study are included in the article/supplementary material, further inquiries can be directed to the corresponding author.
Author Contributions
HC: performed the experiment, theoretical analysis, and writing—original draft. FL: theoretical analysis, Problem Identification. JW, ML, SD, and HW: assisted in the article polishment. XL and LM : review and editing.
Funding
This work was supported by the National Natural Science Foundation of China (Grant No. 21502027); Guangxi Natural Science Foundation (Grant No. 2015GXNSFAA139041); Scientific Research Foundation of Guangxi University (Grant No. XBZ130010); “Innovation and Entrepreneurship Training Program for College Students” of Guangxi University (Grant No. 201910593193).
Conflict of Interest
The authors declare that the research was conducted in the absence of any commercial or financial relationships that could be construed as a potential conflict of interest.
Publisher’s Note
All claims expressed in this article are solely those of the authors and do not necessarily represent those of their affiliated organizations, or those of the publisher, the editors and the reviewers. Any product that may be evaluated in this article, or claim that may be made by its manufacturer, is not guaranteed or endorsed by the publisher.
References
Bai, Y., Shen, X., Li, Y., and Dai, M. (2016). Total Synthesis of (−)-Spinosyn A via Carbonylative Macrolactonization. J. Am. Chem. Soc. 138, 10838–10841. doi:10.1021/jacs.6b07585
Belsito, D., Bickers, D., Bruze, M., Calow, P., Dagli, M. L., Fryer, A. D., et al. (2011). A Toxicological and Dermatological Assessment of Macrocyclic Lactone and Lactide Derivatives when Used as Fragrance Ingredients. Food Chem. Toxicol. 49, S219–S241. doi:10.1016/j.fct.2011.07.052
Blunt, J. W., Copp, B. R., Hu, W.-P., Munro, M. H. G., Northcote, P. T., and Prinsep, M. R. (2009). Marine Natural Products. Nat. Prod. Rep. 26, 170–244. doi:10.1039/b805113p
Boethling, R. S. (2011). Incorporating Environmental Attributes into Musk Design. Green. Chem. 13, 3386–3396. doi:10.1039/c1gc15782e
Christmas, R. B., and Harms, R. H. (1976). Utilization of Egg Shells and Phosphoric Acid as a Source of Phosphorus and Calcium in the Diet of White Leghorn Cockerels. Poult. Sci. 55, 264–267. doi:10.3382/ps.0550264
David, O. R. P. (2020). A Chemical History of Polycyclic Musks. Chem. Eur. J. 26, 7537–7555. doi:10.1002/chem.202000577
De Léséleuc, M., and Collins, S. K. (2015). Direct Macrolactonization of Seco Acids via Hafnium(IV) Catalysis. ACS Catal. 5, 1462–1467. doi:10.1021/acscatal.5b00082
Ebadi Pour, N., Dumeignil, F., Katryniok, B., Delevoye, L., Revel, B., and Paul, S. (2021). Investigating the Active Phase of Ca-Based Glycerol Polymerization Catalysts: On the Importance of Calcium Glycerolate. Mol. Catal. 507, 111571. doi:10.1016/j.mcat.2021.111571
Esipovich, A., Rogozhin, A., Danov, S., Belousov, A., and Kanakov, E. (2018). The Structure, Properties and Transesterification Catalytic Activities of the Calcium Glyceroxide. Chem. Eng. J. 339, 303–316. doi:10.1016/j.cej.2018.01.142
Fuwa, H., Suzuki, T., Kubo, H., Yamori, T., and Sasaki, M. (2011). Total Synthesis and Biological Assessment of (−)-Exiguolide and Analogues. Chem. Eur. J. 17, 2678–2688. doi:10.1002/chem.201003135
Gao, Y., and Xu, C. (2012). Synthesis of Dimethyl Carbonate over Waste Eggshell Catalyst. Catal. Today 190, 107–111. doi:10.1016/j.cattod.2011.12.004
Gonthier, E., and Breinbauer, R. (2005). Solid-supported Reagents and Catalysts for the Preparation of Large Ring Compounds. Mol. Divers. 9, 51–62. doi:10.1007/s11030-005-1308-8
Gupta, A. R., and Rathod, V. K. (2018a). Calcium Diglyceroxide Catalyzed Biodiesel Production from Waste Cooking Oil in the Presence of Microwave: Optimization and Kinetic Studies. Renew. Energ. 121, 757–767. doi:10.1016/j.renene.2017.11.027
Gupta, A. R., and Rathod, V. K. (2018b). Waste Cooking Oil and Waste Chicken Eggshells Derived Solid Base Catalyst for the Biodiesel Production: Optimization and Kinetics. Waste Manag. 79, 169–178. doi:10.1016/j.wasman.2018.07.022
Gupta, A. R., Yadav, S. V., and Rathod, V. K. (2015). Enhancement in Biodiesel Production Using Waste Cooking Oil and Calcium Diglyceroxide as a Heterogeneous Catalyst in Presence of Ultrasound. Fuel 158, 800–806. doi:10.1016/j.fuel.2015.05.064
International Flavors and Fragrances Inc (2017). Annual Report 2017. Available at: http://ir.iff.com/financialinformation/quarterly-results.
Jelić, D., and Antolović, R. (2016). From Erythromycin to Azithromycin and New Potential Ribosome-Binding Antimicrobials. Antibiotics 5, 29–13. doi:10.3390/antibiotics5030029
Joshi, G., Rawat, D. S., Lamba, B. Y., Bisht, K. K., Kumar, P., Kumar, N., et al. (2015). Transesterification of Jatropha and Karanja Oils by Using Waste Egg Shell Derived Calcium Based Mixed Metal Oxides. Energ. Convers. Manag. 96, 258–267. doi:10.1016/j.enconman.2015.02.061
Kamkum, P., Atiwongsangthong, N., Muanghlua, R., and Vittayakorn, N. (2015). Application of Chicken Eggshell Waste as a Starting Material for Synthesizing Calcium Niobate (Ca4Nb2O9) Powder. Ceramics Int. 41, S69–S75. doi:10.1016/j.ceramint.2015.03.189
Khazaei, A., Khazaei, M., and Nasrollahzadeh, M. (2017a). Nano-Fe 3 O 4 @SiO 2 Supported Pd(0) as a Magnetically Recoverable Nanocatalyst for Suzuki Coupling Reaction in the Presence of Waste Eggshell as Low-Cost Natural Base. Tetrahedron 73, 5624–5633. doi:10.1016/j.tet.2017.05.054
Khazaei, M., Khazaei, A., Nasrollahzadeh, M., and Tahsili, M. R. (2017b). Highly Efficient Reusable Pd Nanoparticles Based on Eggshell: Green Synthesis, Characterization and Their Application in Catalytic Reduction of Variety of Organic Dyes and Ligand-free Oxidative Hydroxylation of Phenylboronic Acid at Room Temperature. Tetrahedron 73, 5613–5623. doi:10.1016/j.tet.2017.04.016
Kirst, H. A. (2010). The Spinosyn Family of Insecticides: Realizing the Potential of Natural Products Research. J. Antibiot. 63, 101–111. doi:10.1038/ja.2010.5
Kopp, F., Stratton, C. F., Akella, L. B., and Tan, D. S. (2012). A Diversity-Oriented Synthesis Approach to Macrocycles via Oxidative Ring Expansion. Nat. Chem. Biol. 8, 358–365. doi:10.1038/nchembio.911
Kouzu, M., Hidaka, J.-s., Wakabayashi, K., and Tsunomori, M. (2010). Solid Base Catalysis of Calcium Glyceroxide for a Reaction to Convert Vegetable Oil into its Methyl Esters. Appl. Catal. A: Gen. 390, 11–18. doi:10.1016/j.apcata.2010.09.029
Kouzu, M., Kasuno, T., Tajika, M., Yamanaka, S., and Hidaka, J. (2008). Active Phase of Calcium Oxide Used as Solid Base Catalyst for Transesterification of Soybean Oil with Refluxing Methanol. Appl. Catal. A: Gen. 334, 357–365. doi:10.1016/j.apcata.2007.10.023
León-Reina, L., Cabeza, A., Rius, J., Maireles-Torres, P., Alba-Rubio, A. C., and López Granados, M. (2013). Structural and Surface Study of Calcium Glyceroxide, an Active Phase for Biodiesel Production under Heterogeneous Catalysis. J. Catal. 300, 30–36. doi:10.1016/j.jcat.2012.12.016
Li, Y., Yin, X., and Dai, M. (2017). Catalytic Macrolactonizations for Natural Product Synthesis. Nat. Prod. Rep. 34, 1185–1192. doi:10.1039/c7np00038c
Luesch, H., Yoshida, W. Y., Harrigan, G. G., Doom, J. P., Moore, R. E., and Paul, V. J. (2002). Lyngbyaloside B, a New Glycoside Macrolide from a Palauan marine Cyanobacterium, Lyngbya Sp. J. Nat. Prod. 65, 1945–1948. doi:10.1021/np0202879
M, K., and V, A. M. S. (2018). Eggshell as Heterogeneous Catalyst for Synthesis of Biodiesel from High Free Fatty Acid Chicken Fat and its Working Characteristics on a CI Engine. J. Environ. Chem. Eng. 6, 4490–4503. doi:10.1016/j.jece.2018.06.027
Marinković, D. M., Stanković, M. V., Veličković, A. V., Avramović, J. M., Miladinović, M. R., Stamenković, O. O., et al. (2016). Calcium Oxide as a Promising Heterogeneous Catalyst for Biodiesel Production: Current State and Perspectives. Renew. Sust. Energ. Rev. 56, 1387–1408. doi:10.1016/j.rser.2015.12.007
Marwaha, A., Rosha, P., Mohapatra, S. K., Mahla, S. K., and Dhir, A. (2018). Waste Materials as Potential Catalysts for Biodiesel Production: Current State and Future Scope. Fuel Process. Tech. 181, 175–186. doi:10.1016/j.fuproc.2018.09.011
Matsuda, H., Watanabe, S., and Yamamoto, K. (2007). New Macrocyclic Musk Compounds. Perspect. Flavor Fragr. Res. 1, 155–161. doi:10.1002/9783906390475.ch13
Mohazzab, B. F., Jaleh, B., Nasrollahzadeh, M., Khazalpour, S., Sajjadi, M., and Varma, R. S. (2020). Upgraded Valorization of Biowaste: Laser-Assisted Synthesis of Pd/Calcium Lignosulfonate Nanocomposite for Hydrogen Storage and Environmental Remediation. ACS Omega 5, 5888–5899. doi:10.1021/acsomega.9b04149
Morales-Serna, J. A., Sánchez, E., Velázquez, R., Bernal, J., García-Ríos, E., Gaviño, R., et al. (2010). Highly Efficient Macrolactonization of ω-hydroxy Acids Using Benzotriazole Esters: Synthesis of Sansalvamide A. Org. Biomol. Chem. 8, 4940–4948. doi:10.1039/c0ob00161a
Morin, É., Sosoe, J., Raymond, M., Amorelli, B., Boden, R. M., and Collins, S. K. (2019). Synthesis of a Renewable Macrocyclic Musk: Evaluation of Batch, Microwave, and Continuous Flow Strategies. Org. Process. Res. Dev. 23, 283–287. doi:10.1021/acs.oprd.8b00450
Mosaddegh, E., and Hassankhani, A. (2014). Preparation and Characterization of Nano-CaO Based on Eggshell Waste: Novel and green Catalytic Approach to Highly Efficient Synthesis of Pyrano[4,3-B]pyrans. Chin. J. Catal. 35, 351–356. doi:10.1016/s1872-2067(12)60755-4
Nagarajan, M., Kumar, V. S., and Rao, B. V. (1999). An Improved and Novel Approach to Macrolactonisation Using Di-tert-butyl Dicarbonate. Tetrahedron 55, 12349–12360. doi:10.1016/s0040-4020(99)00718-8
Nasrollahzadeh, M., Sajadi, S. M., and Hatamifard, A. (2016). Waste Chicken Eggshell as a Natural Valuable Resource and Environmentally Benign Support for Biosynthesis of Catalytically Active Cu/eggshell, Fe3O4/eggshell and Cu/Fe3O4/eggshell Nanocomposites. Appl. Catal. B: Environ. 191, 209–227. doi:10.1016/j.apcatb.2016.02.042
Odetoye, T. E., Agu, J. O., and Ajala, E. O. (202110565). Biodiesel Production from Poultry Wastes: Waste Chicken Fat and Eggshell. J. Environ. Chem. Eng. 9, 105654. doi:10.1016/j.jece.2021.105654
Ohloff, G., and Pickenhagen, W. (2012). Scent and Chemistry: the Molecular World of Odors. Choice Rev. Online 49, 49–6894. doi:10.5860/choice.49-6894
Oliveira, D. A., Benelli, P., and Amante, E. R. (2013). A Literature Review on Adding Value to Solid Residues: Egg Shells. J. Clean. Prod. 46, 42–47. doi:10.1016/j.jclepro.2012.09.045
Ookoshi, T., and Onaka, M. (1998). Zeolite-catalyzed Macrolactonization of ω-hydroxyalkanoic Acids in a Highly Concentrated Solution. Tetrahedron Lett. 39, 293–296. doi:10.1016/S0040-4039(97)10545-7
Parenty, A., Moreau, X., Niel, G., and Campagne, J.-M. (2013). Update 1 of: Macrolactonizations in the Total Synthesis of Natural Products. Chem. Rev. 113, PR1–PR40. doi:10.1021/cr300129n
Punj, S., and Singh, K. (2020). Bioactive Calcium Silicate Glass Synthesized from Sustainable Biomass Wastes. Biofuels, Bioprod. Bioref. 14, 1141–1151. doi:10.1002/bbb.2135
Rahman, W. U., Fatima, A., Anwer, A. H., Athar, M., Khan, M. Z., Khan, N. A., et al. (2019). Biodiesel Synthesis from eucalyptus Oil by Utilizing Waste Egg Shell Derived Calcium Based Metal Oxide Catalyst. Process Saf. Environ. Prot. 122, 313–319. doi:10.1016/j.psep.2018.12.015
Reyero, I., Arzamendi, G., and Gandía, L. M. (2014). Heterogenization of the Biodiesel Synthesis Catalysis: CaO and Novel Calcium Compounds as Transesterification Catalysts. Chem. Eng. Res. Des. 92, 1519–1530. doi:10.1016/j.cherd.2013.11.017
Ruppert, A. M., Meeldijk, J. D., Kuipers, B. W. M., Erné, B. H., and Weckhuysen, B. M. (2008). Glycerol Etherification over Highly Active CaO-Based Materials: New Mechanistic Aspects and Related Colloidal Particle Formation. Chem. Eur. J. 14, 2016–2024. doi:10.1002/chem.200701757
Sánchez-Cantú, M., Reyes-Cruz, F. M., Rubio-Rosas, E., Pérez-Díaz, L. M., Ramírez, E., and Valente, J. S. (2014). Direct Synthesis of Calcium Diglyceroxide from Hydrated Lime and Glycerol and its Evaluation in the Transesterification Reaction. Fuel 138, 126–133. doi:10.1016/j.fuel.2014.08.006
Sytniczuk, A., Forcher, G., Grotjahn, D. B., and Grela, K. (2018). Sequential Alkene Isomerization and Ring‐Closing Metathesis in Production of Macrocyclic Musks from Biomass. Chem. Eur. J. 24, 10403–10408. doi:10.1002/chem.201800728
Taufiq-Yap, Y. H., Wong, P., Marliza, T. S., Nurul Suziana, N. M., Tang, L. H., and Sivasangar, S. S. (2014). Hydrogen Production from wood Gasification Promoted by Waste Eggshell Catalyst. Int. J. Energ. Res. 37, 1866–1871. doi:10.1002/er.3003
Taylor, R., Slade, P., Aldous, G., Wilding, I., Siddiqui, O., and Whitehouse, M. (1992). Preparation and Properties of a Glycerolatocalcium Complex. Aust. J. Chem. 45, 1179–1185. doi:10.1071/CH9921179
Verziu, M., Coman, S. M., Richards, R., and Parvulescu, V. I. (2011). Transesterification of Vegetable Oils over CaO Catalysts. Catal. Today 167, 64–70. doi:10.1016/j.cattod.2010.12.031
Wei, Z., Xu, C., and Li, B. (2009). Application of Waste Eggshell as Low-Cost Solid Catalyst for Biodiesel Production. Bioresour. Tech. 100, 2883–2885. doi:10.1016/j.biortech.2008.12.039
Wright, A. E., Botelho, J. C., Guzmán, E., Harmody, D., Linley, P., McCarthy, P. J., et al. (2007). Neopeltolide, a Macrolide from a Lithistid Sponge of the Family Neopeltidae. J. Nat. Prod. 70, 412–416. doi:10.1021/np060597h
Xie, Z.-Y., Deng, J., and Fu, Y. (2018). W(OTf)6 -Catalyzed Synthesis of γ-Lactones by Ring Contraction of Macrolides or Ring Closing of Terminal Hydroxyfatty Acids in Ionic Liquid. ChemSusChem 11, 2332–2339. doi:10.1002/cssc.201800587
Xu, C., Nasrollahzadeh, M., Selva, M., Issaabadi, Z., and Luque, R. (2019). Waste-to-wealth: Biowaste Valorization into Valuable Bio(nano)materials. Chem. Soc. Rev. 48, 4791–4822. doi:10.1039/c8cs00543e
Yan, S., Kim, M., Salley, S. O., and Ng, K. Y. S. (2009). Oil Transesterification over Calcium Oxides Modified with Lanthanum. Appl. Catal. A. Gen. 360, 163–170. doi:10.1016/j.apcata.2009.03.015
Yusuff, A. S., Adeniyi, O. D., Olutoye, M. A., and Akpan, U. G. (2018). Development and Characterization of a Composite Anthill-Chicken Eggshell Catalyst for Biodiesel Production from Waste Frying Oil. IJTech 9, 110–119. doi:10.14716/ijtech.v9i1.1166
Zhang, W. W., Gao, T. T., Xu, L. J., and Li, B. J. (2018). Macrolactonization of Alkynyl Alcohol through Rh(I)/Yb(III) Catalysis. Org. Lett. 20, 6534–6538. doi:10.1021/acs.orglett.8b02858
Keywords: eggshell waste, low-temperature calcination, calcium glycerolate, macrolactonization, cyclopentadecanolide
Citation: Cheng H, Wei J, Liang M, Dai S, Liu X, Ma L, Wang H and Lai F (2021) Calcium Glycerolate Catalyst Derived from Eggshell Waste for Cyclopentadecanolide Synthesis. Front. Chem. 9:770247. doi: 10.3389/fchem.2021.770247
Received: 03 September 2021; Accepted: 18 November 2021;
Published: 09 December 2021.
Edited by:
Vasile I. Parvulescu, University of Bucharest, RomaniaReviewed by:
R. K. Sharma, University of Delhi, IndiaPaul-Henri Ducrot, INRA UMR1318 Institut Jean Pierre Bourgin, France
Mahmoud Nasrollahzadeh, University of Qom, Iran
Copyright © 2021 Cheng, Wei, Liang, Dai, Liu, Ma, Wang and Lai. This is an open-access article distributed under the terms of the Creative Commons Attribution License (CC BY). The use, distribution or reproduction in other forums is permitted, provided the original author(s) and the copyright owner(s) are credited and that the original publication in this journal is cited, in accordance with accepted academic practice. No use, distribution or reproduction is permitted which does not comply with these terms.
*Correspondence: Fang Lai, bGFpZmFuZzIwMDYwOUAxMjYuY29t