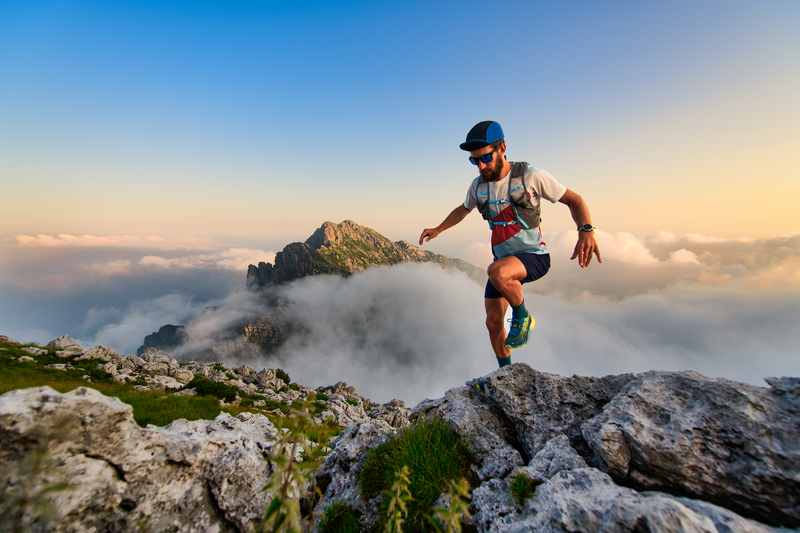
95% of researchers rate our articles as excellent or good
Learn more about the work of our research integrity team to safeguard the quality of each article we publish.
Find out more
ORIGINAL RESEARCH article
Front. Chem. , 04 November 2021
Sec. Chemical Physics and Physical Chemistry
Volume 9 - 2021 | https://doi.org/10.3389/fchem.2021.765374
This article is part of the Research Topic Metal-Free Room-Temperature Phosphorescence View all 11 articles
The development of molecular crystalline materials with efficient room-temperature phosphorescence has been obtained much attention due to their fascinating photophysical properties and potential applications in the fields of data storage, bioimaging and photodynamic therapy. Herein, a new co-crystal complex [(DCPA) (AD)2] (DCPA = 9,10-di (4-carboxyphenyl)anthracene; AD = acridine) has been synthesized by a facile solvothermal process. Crystal structure analysis reveals that the co-crystal possesses orderly and alternant arrangement of DCPA donors and AD acceptors at molecular level. Fixed by strong hydrogen bonds, the DCPA molecule displays seriously twisty spatial conformation. Density functional theory (DFT) calculations show well separation of HOMO and LUMO for this co-crystal system, suggesting the efficient triplet excitons generation. Photoluminescence measurements show intensive cyan fluorescence (58.20 ns) and direct white phosphorescence (325 µs) emission at room-temperature. The transient current density–time curve reveals a typical switching electric response under the irradiation of simulated light, reveal that the [(DCPA) (AD)2] co-crystal has a high photoelectric response performance.
The rational design of molecular crystalline materials with long-lived room-temperature phosphorescence (RTP) has atracted tremendous attentions owing to their extended potential to create new opportunities in the development of photocatalytic reactions, photodynamic therapy, optical storage, organic light emitting diodes, and bioimaging (Bhattacharjee and Hirata, 2020; Jiang et al., 2018; Gao and Ma, 2021; Hirata, 2019; Gu et al., 2019; Lei et al., 2019; Wang et al., 2020; Wang et al., 2021; Li and Li, 2020; Yang et al., 2019; Yang et al., 2020; Gao et al., 2021; Chen et al., 2021). Besides the traditional noble-metal (ruthenium, platinum, iridium) based complexes (Xiang et al., 2013), breakthroughs have been achieved during the past decade on pure organics, polymers, metal–organic frameworks (MOFs), organic−inorganic hybrid perovskite and host–guest doping (Mu et al., 2017; Lu et al., 2019; Zhou and Yan, 2019; Lei et al., 2020; Liu et al., 2020; Lei et al., 2021; Wu et al., 2021; Zhou et al., 2021). Promising strategies (such as crystallization, H-aggregation, halogen bonding) have also been vastly accepted to obtain efficient RTP (Bolton et al., 2011; Gong et al., 2015; Kenry and Liu, 2019; Wang et al., 2020), and the inherent principle is absolutely focused on promoting triplet excitons generation.
Considering the spin-forbidden intersystem crossing (ISC) from excited singlet state to excited triplet state, the rate of ISC can be enhanced by reducing the energy gap (ΔEST) between the lowest singlet excited state and a nearby triplet state. Small ΔEST can be achieved by designing the charge transfer of donor-acceptor system with large spatial separation between the HOMO and LUMO (Parke and Rivard, 2018). To date, many single component organic molecules with twisted donor-acceptor spatial conformation have been demonstrated as efficient RTP materials (Xiao et al., 2020; Xu et al., 2021). However, triplet state excitons of multi-component co-crystal donor-acceptor systems are still relatively limited (Zhou and Yan, 2019; Zhou et al., 2020), and the systematical investigation is needed for well understanding the relationship between their structures and photophysical behaviors.
In this paper, one new type of co-crystal [(DCPA) (AD)2] has been obtained under solvothermal conditions by the selection of 9,10-di (4-carboxyphenyl)anthracene (DCPA) electron donor and acridine (AD) electron acceptor. The obtained donor-acceptor co-crystal system shows alternant arrangement of DCPA and AD components at the molecular level. The crystal structure and density functional theory (DFT) calculations reveal that the DCPA molecule fixed by strong hydrogen bonds displays seriously twisty spatial conformation. This structure feature affords well separation of HOMO-LUMO, promoting for the generation of triplet excitons. As a result, the formation of [(DCPA) (AD)2] co-crystal exhibits cyan fluorescence and direct white long-lived RTP under ambient condition.
9,10-di (4-carboxyphenyl)anthracene (DCPA), acridine (AD) and anhydrous ethanol were purchased commercially. Single-crystal X-ray diffraction data were collected by Oxford Diffraction SuperNova area-detector diffractometer with the program of CrysAlisPro. The crystal structure was solved by SHELXS-2014 and SHELXL-2014 software (Sheldrick 2008). The crystallographic data for [(DCPA) (AD)2] were listed in Table 1. The CIF file (CCDC No. 2104581) presented in this study can be downloaded free of charge via http://www.ccdc.cam.ac.uk/conts/retrieving.html.
Phase purity of co-crystal powders were tested by Bruker D8-ADVANCE X-ray diffractometer with Cu Kα radiation. Elemental analysis was performed by Perkin–Elmer Elementarvario elemental analysis instrument. Fourier transform infrared (FT-IR) spectra were measured by SHIMADZU IR Spirit-T spectrometer from 4,000 to 400 cm−1 with KBr pellet. UV-vis absorption spectra were detected by Shimadzu UV-3600 plus UV-vis-NIR spectrophotometer. Thermo gravimetric analysis (TGA) experiments were measured by SII EXSTAR6000 TG/DTA6300 thermal analyzer from room temperature to 800°C. The fluorescent and phosphorescent spectra were conducted on Edinburgh FLS1000 fluorescence spectrometer excited by xenon arc lamp (Xe900) and microsecond flash lamp, respectively. The time-resolved phosphorescent decay curves were measured by a microsecond flash lamp with a frequency of 100 Hz. Optoelectronic properties were tested on CHI 660 E electrochemical analyzer in a standard three-electrode system. The working electrode, counter electrode, reference electrode, and electrolyte is [(DCPA) (AD)2] powders modified indium tin oxide (ITO) glass, platinum wire, Ag/AgCl, and 0.5 M sodium sulfate aqueous solution, respectively. The linear sweep voltammetry (LSV) was recorded by the voltage rang of 0.2 to −1 V with a scan rate of 50 mV/s. The transient photocurrent were measured by on–off cycle’s illumination of Xe lamp (300 W) with bias potential (vs Ag/AgCl) of 0 and −0.5 V.
A mixture of 9,10-di (4-carboxyphenyl)anthracene (0.1 mmol, 41.8 mg), acridine (0.2 mmol, 35.8 mg) and 8 ml EtOH was sealed into a Teflon reactor (23 ml), and heated at 120°C for 12 h. Then, the light yellow block crystals can be obtained after naturally cooled to room temperature. Anal. Calc (%) for C27H18NO2: C 83.48, H 4.67, N 3.61; found (%): C 83.12, H 4.33, N 3.46. IR (KBr pellet, cm−1): 3,415(w), 3,054(w), 1,947(w), 1,692(s), 1,607(m), 1,572(m), 1,524(m), 1,440(m), 1,401(m), 1,281(s), 1,100(m), 920(m), 853(w), 774(s), 735(s), 673(m), 505(m).
High-grade light yellow block single crystals of the two-component [(DCPA) (AD)2] co-crystal were synthesized under the solvothermal condition from the mixture of DCPA and AD (Figure 1A) with a 1:2 stoichiometry in ethanol solution. Single-crystal X-ray diffraction analysis reveals that [(DCPA) (AD)2] crystallizes in triclinic Pī space group, and the asymmetric unit consists of two AD and one DCPA molecules. In the co-crystal system, the DCPA molecules are linked together by C‒H···O hydrogen bonds (C6‒H6···O2: H6···O2 = 2.69 Å, ∠C6‒H6···O2 = 141.30°) to form a 1D chain (Figure 1B). Pairs of AD molecules arrange in a head-to-tail π-stacking mode with short interplanar distance of 3.66 Å, which extends the DCPA 1D chain into a 2D sheet with the alternant arrangement of DCPA and AD molecules (Figure 1C).
FIGURE 1. (A) Chemical structures of DCPA and AD molecules in this work. (B) 1D chain-like structure of DCPA extended by C‒H···O hydrogen bonds. (C) View of the 2D sheet constructed by the alternant arrangement of DCPA and AD molecules through C‒H···O and O‒H···N hydrogen bonds. (D) Torsion angles between benzene acid arm and the anthracene core.
Owing to above mentioned hydrogen bond interactions, the DCPA chromophores are highly fixed in an ordered arrangement at the molecular level, which exhibits a seriously twist conformation with torsion angles between benzene acid arm and the anthracene core up to 84.5° (Figure 1D). These supramolecular interactions also provide rigid environment to restrict the molecular motions/vibrations, minimizing the nonradiative loss of single/triplet excitons and facilitate for efficient emission (Yang et al., 2020).
Powder X-ray diffraction (PXRD) experiment was conducted to detect the phase purity of [(DCPA) (AD)2] co-crystal (Figure 2A). The experiment diffraction peaks match well with the simulated one, providing the high purity and good crystalline degree of the as-synthesized samples. Thermo gravimetric (Figure 2B) curve shows the first weight loss of about 44.50% in the range of 200–283°C, assigning to the loss of AD molecules (calculated: 46.14%). Additional heating results in the gradual decomposition of framework of co-crystal.
FIGURE 2. (A) PXRD patterns of simulated (black) and as synthesized DCPA-AD (red). (B) Thermo gravimetric analysis curve of DCPA-AD.
The steady-state, transient-state photoluminescence (PL) spectra and time-resolved PL decay curves of both [(DCPA) (AD)2] co-crystal, pure DCPA and AD in solid state were recorded at room temperture. Figure 3A illustrates the fluorescence spectra of DCPA in solid state, which shows strong dark-blue emission owing to the presence of the anthracene chromophore (λex = 329 nm). The fluorescence decay curve estimated at the maximal emission peak at 447 nm gives rise to a short lifetime of 1.01 ns(Figure 3B), whereas the single component AD has an emission peak at 396 nm and lifetime of 2.88 ns(Yang et al., 2020). By contrast, the formation of co-crystal presents a red-shift of the emission peak to long wavelength at 474 nm, attaching with a weak shoulder at about 443 nm when excited at 365 nm (Figure 3C), suggesting the charge transfer interaction between the DCPA donor and AD acceptor. Apart from the emission peak, the [(DCPA) (AD)2] co-crystal also shows much longer fluorescence lifetime up to 58.20 ns(Figure 3D), which is more than 50 times as long as that of free DCPA molecules in solid state.
FIGURE 3. Fluorescence spectra (A) decay curve (B) of DCPA in solid state (λex = 329 nm). Fluorescence spectra (C) decay curve (D) of [(DCPA) (AD)2] co-crystal in solid state (λex = 365 nm). Insert shows solid state samples under UV (365 nm) light radiation. Phosphorescence spectra (E) decay curve (F) of [(DCPA) (AD)2] co-crystal in solid state (λex = 365 nm). Insert shows CIE-1931 chromaticity diagram (0.33,0.34) of [(DCPA) (AD)2] co-crystal with white phosphorescence emission. All of these measurements were recorded under ambient condition.
The delayed PL spectrum shows a broad emission region spanning nearly the whole visible spectra with a maximum peak at 570 nm (Figure 3E). The time-resolved PL decay curve affords a long lifetime of 325 µs, indicating long-lived RTP emission of [(DCPA) (AD)2] co-crystal (Figure 3F). The inserts show the cyan emission of [(DCPA) (AD)2] crystalline powders irradiated under UV (365 nm) and the CIE-1931 chromaticity coordinate obtained from the phosphorescence spectra. The chromaticity coordinate of (0.33,0.34) is close to the optimum white-light with value of (0.33,0.33). The above results indicate that the fromation of co-crystal can largely tune the fluorescence emission of DCPA from dark-blue to cyan, and prolong the lifetime more than 50 times. In our opinion, the strong supramolecular interactions efficiently reduce the nonradiative loss of single/triplet excitons, and further enable prolonged PL lifetime.
Density functional theory (DFT) calculations were conducted by Dmol3 module in Material Studio software package (Delley 2000) based on the X-ray single crystal diffraction data of [(DCPA) (AD)2]. The results show the highest occupied molecular orbital (HOMO) is occupied by the anthracene core of DCPA molecules, whereas the lowest unoccupied molecular orbital (LUMO) is exclusively located on the benzene acid groups. The LUMO+1 mainly appears on AD molecules (Figure 4). Herein, the seriously twist conformation of DCPA molecule leads to large spatial separation of the HOMO and LUMO. The alternant arrangement of DCPA electron donor and AD electron acceptor further promotes the separation of molecular orbitals, boosting the spin-orbit coupling and intersystem crossing for efficient triplet state exciton generation.
It has been found that the long-lived triplet state excitons have more chance for the electron migration (Yang et al., 2019). Encouraged by the long-lived RTP of [(DCPA) (AD)2] co-crystal in this work, its photo-electronic properties have been further conducted by a three-electrode system in Na2SO4 aqueous solution. The UV-Vis absorption spectrum shows an optical band gap of 2.63 eV (471 nm), consisting with the fluorescence emission peak (Figure 5A). The linear sweep voltammetry (LSV) curve reveals that the [(DCPA) (AD)2] co-crystal material can generate large current with the addition of negative potential (Figure 5B). The absence of redox peak suggests that the [(DCPA) (AD)2] co-crystal is stable within the applied bias potential from 0.2 to −1 V.
FIGURE 5. (A) UV-VIS-NIR absorption of as synthesized [(DCPA) (AD)2]. (B) The linear sweep voltammetry curve of as synthesized [(DCPA) (AD)2] modified ITO electrode measured in 0.5 M Na2SO4 aqueous solution. Transient current density–time curve of [(DCPA) (AD)2] at bias potential of 0 V (C) and −0.5 V (D) with the periodic on-off cycles of light radiation.
The transient current density–time curve reveals a typical on/off switching response under the irradiation of simulated light. Without only bias potential, it generates high photocurrent up to −3.1 μA cm−2 with the momentary light radiation. Under the initiatory dark condition, extremely small dark current of about 0.002 μA cm−2 can be detected (Figure 5C). The rate of current between light radiation and dark conditions was calculated up to 1,550. By the addition of −0.5 V bias potential, it generates more large current of about −46.5 μA cm−2 under light radiation (Figure 5D). All these results reveal that the [(DCPA) (AD)2] co-crystal has superior photoelectric response performance, which can be applied in the future photoelectric detector device.
In summary, we report a rare example of direct white-light RTP co-crystal material [(DCPA) (AD)2], which can be synthesized under a facile solvothermal condition. The framework of [(DCPA) (AD)2] shows an orderly distribution of heterojuction at the molecular level: alternant arrangement of DCPA electronic donors and AD electron acceptors bonded together through strong C‒H···O and O‒H···N hydrogen bonds. Fixed by these supramolecular interactions, the molecular motions/vibrations can be restricted, which affords long-lasting singlet and triplet excitons through minimize the nonradiative loss. In addition, the seriously twist conformation of DCPA molecule is beneficial to the separation of molecule orbitals. Combined with the introduction of AD acceptor, it provides efficient platform for long distance exciton transfer and good electron-hole separation ability, possessing superior photoelectric response performance. Therefore, this work not only develops a new type of white-light RTP co-crystal, but also provides a perspective to deeply understand the relationship among molecular structure, stacking mode and photoelectric performance.
The data presented in the study are deposited in the (Cambridge Crystallographic Data Centre) repository, accession number (2104581).
XY, LF, and D conceived the idea and designed research. WJ, JR, XK, and X synthesized and characterized materials; all authors analyzed data and wrote the paper.
This work was supported by the National Natural Science Foundation of China (No. 21971100, 21771021, 21822501, and 22061130206), Project of Central Plains Science and Technology Innovation Leading Talents of Henan Province (No. 204200510001), Project for Science and Technology Innovation Talents in Universities of Henan Province (No. 21HASTIT006), and Key Scientific Research Projects of Higher Education of Henan Province (No. 20A150005).
The authors declare that the research was conducted in the absence of any commercial or financial relationships that could be construed as a potential conflict of interest.
All claims expressed in this article are solely those of the authors and do not necessarily represent those of their affiliated organizations, or those of the publisher, the editors, and the reviewers. Any product that may be evaluated in this article, or claim that may be made by its manufacturer, is not guaranteed or endorsed by the publisher.
Bhattacharjee, I., and Hirata, S. (2020). Highly Efficient Persistent Room‐Temperature Phosphorescence from Heavy Atom‐Free Molecules Triggered by Hidden Long Phosphorescent Antenna. Adv. Mater. 32, 2001348. doi:10.1002/adma.202001348
Bolton, O., Lee, K., Kim, H.-J., Lin, K. Y., and Kim, J. (2011). Activating Efficient Phosphorescence from Purely Organic Materials by Crystal Design. Nat. Chem 3, 205–210. doi:10.1038/nchem.984
Chen, C., Chi, Z., Chong, K. C., Batsanov, A. S., Yang, Z., Mao, Z., et al. (2021). Carbazole Isomers Induce Ultralong Organic Phosphorescence. Nat. Mater. 20, 175–180. doi:10.1038/s41563-020-0797-2
Delley, B. (2000). From Molecules to Solids with the DMol3 Approach. J. Chem. Phys. 113 (18), 7756–7764. doi:10.1063/1.1316015
Gao, H., and Ma, X. (2021). Recent Progress on Pure Organic Room Temperature Phosphorescent Polymers. Aggregate 2. doi:10.1002/agt2.38
Gao, R., Kodaimati, M. S., and Yan, D. (2021). Recent Advances in Persistent Luminescence Based on Molecular Hybrid Materials. Chem. Soc. Rev. 50, 5564–5589. doi:10.1039/d0cs01463j
Gong, Y., Zhao, L., Peng, Q., Fan, D., Yuan, W. Z., Zhang, Y., et al. (2015). Crystallization-induced Dual Emission from Metal- and Heavy Atom-free Aromatic Acids and Esters. Chem. Sci. 6, 4438–4444. doi:10.1039/c5sc00253b
Gu, L., Shi, H., Bian, L., Gu, M., Ling, K., Wang, X., et al. (2019). Colour-tunable Ultra-long Organic Phosphorescence of a Single-Component Molecular crystal. Nat. Photon. 13 (6), 406–411. doi:10.1038/s41566-019-0408-4
Hirata, S. (2017). Recent Advances in Materials with Room-Temperature Phosphorescence: Photophysics for Triplet Exciton Stabilization. Adv. Opt. Mater. 5 (17), 1700116. doi:10.1002/adom.201700116
Jiang, K., Wang, Y., Cai, C., and Lin, H. (2018). Conversion of Carbon Dots from Fluorescence to Ultralong Room-Temperature Phosphorescence by Heating for Security Applications. Adv. Mater. 30, 1800783. doi:10.1002/adma.201800783
Kenry, Chen. C., Chen, C., and Liu, B. (2019). Enhancing the Performance of Pure Organic Room-Temperature Phosphorescent Luminophores. Nat. Commun. 10, 2111. doi:10.1038/s41467-019-10033-2
Lei, Y., Dai, W., Guan, J., Guo, S., Ren, F., Zhou, Y., et al. (2020). Wide‐Range Color‐Tunable Organic Phosphorescence Materials for Printable and Writable Security Inks. Angew. Chem. Int. Ed. 59, 16054–16060. doi:10.1002/anie.202003585
Lei, Y., Dai, W., Tian, Y., Yang, J., Li, P., Shi, J., et al. (2019). Revealing Insight into Long-Lived Room-Temperature Phosphorescence of Host-Guest Systems. J. Phys. Chem. Lett. 10, 6019–6025. doi:10.1021/acs.jpclett.9b02411
Lei, Y., Yang, J., Dai, W., Lan, Y., Yang, J., Zheng, X., et al. (2021). Efficient and Organic Host-Guest Room-Temperature Phosphorescence: Tunable Triplet-Singlet Crossing and Theoretical Calculations for Molecular Packing. Chem. Sci. 12, 6518–6525. doi:10.1039/d1sc01175h
Li, Q., and Li, Z. (2020). Molecular Packing: Another Key point for the Performance of Organic and Polymeric Optoelectronic Materials. Acc. Chem. Res. 53, 962–973. doi:10.1021/acs.accounts.0c00060
Liu, S., Fang, X., Lu, B., and Yan, D. (2020). Wide Range zero-thermal-quenching Ultralong Phosphorescence from Zero-Dimensional Metal Halide Hybrids. Nat. Commun. 11, 4649. doi:10.1038/s41467-020-18482-w
Lu, X.-M., Zhai, Z.-M., Liu, X.-Y., Li, F.-F., Yang, X.-G., Li, J.-Y., et al. (2019). Near-infrared Phosphorescence Emission of Zn(II) Coordination Polymer Based on 3,5-Bis(1-Imidazoly)pyridine: Syntheses, Structure and Photoelectron Performance. J. Solid State. Chem. 279, 120958. doi:10.1016/j.jssc.2019.120958
Mu, Y., Shi, H., Wang, Y., Ding, H., and Li, J. (2017). CNDs@zeolite: New Room-Temperature Phosphorescent Materials Derived by Pyrolysis of Organo-Templated Zeolites. J. Mater. Chem. C 5, 10894–10899. doi:10.1039/C7TC03487C
Parke, S. M., and Rivard, E. (2018). Aggregation Induced Phosphorescence in the Main Group. Isr. J. Chem. 58, 915–926. doi:10.1002/ijch.201800039
Sheldrick, G. M. (2008). A Short History ofSHELX. Acta Cryst. Sect A. 64, 112–122. doi:10.1107/S0108767307043930
Wang, D., Xie, Y., Wu, X., Lei, Y., Zhou, Y., Cai, Z., et al. (2021). Excitation-dependent Triplet-Singlet Intensity from Organic Host-Guest Materials: Tunable Color, white-light Emission, and Room-Temperature Phosphorescence. J. Phys. Chem. Lett. 12 (12), 1814–1821. doi:10.1021/acs.jpclett.1c00188
Wang, H.-R., Yang, X.-G., Qin, J.-H., and Ma, L.-F. (2020). Long-lived Room Temperature Phosphorescence of Organic-Inorganic Hybrid Systems. Inorg. Chem. Front. 8, 1942–1950. doi:10.1039/d0qi01508c
Wang, W., Zhang, Y., and Jin, W. J. (2020). Halogen Bonding in Room-Temperature Phosphorescent Materials. Coord. Chem. Rev. 404, 213107. doi:10.1016/j.ccr.2019.213107
Wu, H.-X., Lu, X.-M., Chen, J.-Y., Yang, X.-G., Qin, W.-J., and Ma, L.-F. (2021). Long Afterglow of a Nonporous Coordination Polymer with Tunable Room-Temperature Phosphorescence by the Doping of Dye Molecules. Inorg. Chem. 60 (2), 846–851. doi:10.1021/acs.inorgchem.0c02888
Xiang, H., Cheng, J., Ma, X., Zhou, X., and Chruma, J. J. (2013). Near-infrared Phosphorescence: Materials and Applications. Chem. Soc. Rev. 42, 6128–6185. doi:10.1039/c3cs60029g
Xiao, F., Wang, M., Lei, Y., Dai, W., Zhou, Y., Liu, M., et al. (2020). Achieving crystal-induced Room Temperature Phosphorescence and Reversible Photochromic Properties by strong Intermolecular Interactions. J. Mater. Chem. C 8, 17410–17416. doi:10.1039/d0tc03980b
Xu, Z., Climent, C., Brown, C. M., Hean, D., Bardeen, C. J., Casanova, D., et al. (2021). Controlling Ultralong Room Temperature Phosphorescence in Organic Compounds with Sulfur Oxidation State. Chem. Sci. 12, 188–195. doi:10.1039/d0sc04715e
Yang, X.-G., Lu, X.-M., Zhai, Z.-M., Zhao, Y., Liu, X.-Y., Ma, L.-F., et al. (2019). Facile Synthesis of a Micro-scale MOF Host-Guest with Long-Lasting Phosphorescence and Enhanced Optoelectronic Performance. Chem. Commun. 55, 11099–11102. doi:10.1039/c9cc05708k
Yang, X.-G., Zhai, Z.-M., Lu, X.-M., Ma, L.-F., and Yan, D. (2020). Fast Crystallization-Deposition of Orderly Molecule Level Heterojunction Thin Films Showing Tunable Up-Conversion and Ultrahigh Photoelectric Response. ACS Cent. Sci. 6, 1169–1178. doi:10.1021/acscentsci.0c00447
Yang, X.-G., Zhai, Z.-M., Lu, X.-M., Qin, J.-H., Li, F.-F., and Ma, L.-F. (2020). Hexanuclear Zn(II)-Induced Dense π-Stacking in a Metal-Organic Framework Featuring Long-Lasting Room Temperature Phosphorescence. Inorg. Chem. 59, 10395–10399. doi:10.1021/acs.inorgchem.0c01415
Zhou, B., Xiao, G., and Yan, D. (2021). Boosting Wide‐Range Tunable Long‐Afterglow in 1D Metal-Organic Halide Micro/Nanocrystals for Space/Time‐Resolved Information Photonics. Adv. Mater. 33, 2007571. doi:10.1002/adma.202007571
Zhou, B., and Yan, D. (2019). Hydrogen-Bonded Two-Component Ionic Crystals Showing Enhanced Long-Lived Room-Temperature Phosphorescence via TADF-Assisted Förster Resonance Energy Transfer. Adv. Funct. Mater. 29, 1807599. doi:10.1002/adfm.201807599
Zhou, B., and Yan, D. (2019). Simultaneous Long‐Persistent Blue Luminescence and High Quantum Yield within 2D Organic-Metal Halide Perovskite Micro/Nanosheets. Angew. Chem. Int. Ed. 58, 15128–15135. doi:10.1002/ange.20190976010.1002/anie.201909760
Keywords: room temperature phosphorescence, co-crystal, white light, triplet excitons, photoelectric response
Citation: Yang X-G, Qin W-J, Zhang J-R, Tian X-K, Fan X, Ma L-F and Yan D (2021) Room-Temperature Phosphorescent Co-Crystal Showing Direct White Light and Photo-Electric Conversion. Front. Chem. 9:765374. doi: 10.3389/fchem.2021.765374
Received: 27 August 2021; Accepted: 23 September 2021;
Published: 04 November 2021.
Edited by:
Wang Zhang Yuan, Shanghai Jiao Tong University, ChinaReviewed by:
Xiaobo Huang, Wenzhou University, ChinaCopyright © 2021 Yang, Qin, Zhang, Tian, Fan, Ma and Yan. This is an open-access article distributed under the terms of the Creative Commons Attribution License (CC BY). The use, distribution or reproduction in other forums is permitted, provided the original author(s) and the copyright owner(s) are credited and that the original publication in this journal is cited, in accordance with accepted academic practice. No use, distribution or reproduction is permitted which does not comply with these terms.
*Correspondence: Dongpeng Yan, eWFuZHBAYm51LmVkdS5jbg==
Disclaimer: All claims expressed in this article are solely those of the authors and do not necessarily represent those of their affiliated organizations, or those of the publisher, the editors and the reviewers. Any product that may be evaluated in this article or claim that may be made by its manufacturer is not guaranteed or endorsed by the publisher.
Research integrity at Frontiers
Learn more about the work of our research integrity team to safeguard the quality of each article we publish.