- 1Molecular Electronics and Photonics Research Unit, Department of Mechanical Engineering and Materials Science and Engineering, Cyprus University of Technology, Limassol, Cyprus
- 2Department of Public and Community Health, School of Public Health, University of West Attica, Athens, Greece
- 3Department of Materials Science and Technology, University of Crete, Heraklion, Greece
This study outlines the synthesis and physicochemical characteristics of a solution-processable iron manganite (FeMnO3) nanoparticles via a chemical combustion method using tartaric acid as a fuel whilst demonstrating the performance of this material as a n-type photoactive layer in all-oxide solar cells. It is shown that the solution combustion synthesis (SCS) method enables the formation of pure crystal phase FeMnO3 with controllable particle size. XRD pattern and morphology images from TEM confirm the purity of FeMnO3 phase and the relatively small crystallite size (∼13 nm), firstly reported in the literature. Moreover, to assemble a network of connected FeMnO3 nanoparticles, β-alanine was used as a capping agent and dimethylformamide (DMF) as a polar aprotic solvent for the colloidal dispersion of FeMnO3 NPs. This procedure yields a ∼500 nm thick FeMnO3 n-type photoactive layer. The proposed method is crucial to obtain functional solution processed NiO/FeMnO3 heterojunction inorganic photovoltaics. Photovoltaic performance and solar cell device limitations of the NiO/FeMnO3-based heterojunction solar cells are presented.
Introduction
A major source of renewable energy is solar energy (Ellabban et al., 2014). Nevertheless, the production of fuels and electricity from solar power is still costly, mainly because of the materials used in building the cells (Ellabban et al., 2014; Hussein, 2015). Except for Si and copper indium gallium selenide solar cells (CIGSSe), which are principal targets for photovoltaic (PV) applications, CdTe and GaAs are also significant photoactive materials. However, the mass production of such photovoltaics is limited due to the high production costs, the indirect bandgap energy (for Si) and the dependence on elements which are expensive (In, Ga, Te) or even hazardous (As, Cd) (Rohatgi, 1996; Mitzi et al., 2011; Kosten et al., 2013; Wang, 2014). Contrarily, hybrid lead halide perovskite solar cells (PVSCs) have been in the epicenter of the current solar cell research because of their facile fabrication process and due to the fact that they appear a high PCE of over 23% (Jung et al., 2019). However, PVSCs have disadvantages as well, that is, ultraviolet light absorption, and humidity and atmospheric oxygen affecting decomposition (Li and Liu, 2017; Fu et al., 2018387). Along with the presence of toxic lead, these factors restrain the advancement of the PVSCs. Therefore, it is essential to find alternatives for inorganic PVs that use inexpensive, eco-friendly and Earth abundant photoactive materials with appropriate semiconductor structure, while at the same time seek improvements in solar cell efficiency.
Metal oxide (MeOx) based solar cells have the potential to resolve some of the issues which arise in conventional solar cells. The all-oxide perspective is advantageous due to its excellent chemical stability, minor toxicity and ample quantities of metal oxides that effectively permit the manufacturing of solar cells under ambient conditions (Pérez-Tomás et al., 2018). MeOx are typically used as functional layers in solar cells such as transparent conducting front electrodes (ITO, FTO), (Kim et al., 1999; Han et al., 2007) electron (TiO2, SnO2, ZnO, Fe2O3 etc.) (Seo et al., 2018; Xiong et al., 2018; Shin et al., 2019; Papadas et al., 2019) or hole (Cu:NiOx, CuGaO2, NiCo2O4, CuLi:NiCo2O4 etc.) transporting layers, (Galatopoulos et al., 2017; Papadas et al., 2018a; Papadas et al., 2018b; Ioakeimidis et al., 2019) whereas a very small number of MeOx have been used as photoactive layers, primarily Cu2O, CuO and Co2O3 (Pérez-Tomás et al., 2018).
Ferroic semiconductors are now being continually included in the list of materials which are employed to investigate and push the efficiency limits in all-oxide photovoltaics. Ferroic semiconductor materials are considered to be light absorbers, such as Pb(Zr,Ti)O3 (energy band gap (Eg) = 3.63 eV) (Pérez-Tomas et al., 2019), KNbO3 (Eg = 3.8 eV) (Grinberg et al., 2013) and BiFeO3 (Eg = 2.67 eV) (Papadas et al., 2015a). However, what seems to confine their implication to solar cells is their wide energy bandgap that results to low absorption of visible light and, thus, low conductivity. Other ferroelectric oxide semiconductors, like Bi2FeCrO6 (Nechache et al., 2014) and BiMnO3 (Sun et al., 2017), have a suitable bandgap between 1 and 2 eV and are considered as more efficient in absorbing solar light. It is worth mentioning that a power conversion efficiency (PCE) up to ∼8.1% has been recently achieved using a single ferroelectric Bi2FeCrO6 layer fabricated by pulse laser deposited technique with the following structure: SrTiO3/SrRuO3/Bi2CrFeO6/ITO (Nechache et al., 2014). However, without the mentioned method of the thin film deposition which is considered to be a very complicated and energy demanding procedure, it is very unlikely to gain high PCE values (Calnan, 2014). Furthermore, the usage of the low-bandgap KBiFe2O5 material (Eg ∼1.6 eV) in the photovoltaic cells appears to be restricted due to low PCE (∼3 10–3%) (Zhang et al., 2013). Therefore, the above mentioned examples demonstrate that further research is needed in finding more efficient narrow-bandgap, non-toxic and low-cost materials for solar cell devices.
The sol-gel synthesis has been the most frequently used method in the manufacture of MeOx. Nevertheless, to achieve crystallinity and to guarantee an efficient charge–carrier mobility, for metal oxide based active layers, high temperatures are necessary, which increases the cost of manufacturing and also restricts printable applications. These restrictions call for alternative techniques which could operate at lower temperatures. Compared to sol-gel synthesis of MeOx nanoparticles (NPs), the solution combustion synthesis (SCS) of NPs displays considerable advantages, such as use of a simple experimental setup, production of NPs with high crystallinity and pure phase, and exact control of the size and crystal structure of the particles by simple adjusting the fabrication conditions. (Suresh et al., 1991; Mimani and Patil, 2001; Patil et al., 2002a; Bansal, 2005; Deganello et al., 2009; Jadhav et al., 2011; Jiang et al., 2014) The SCS seems to be adaptable and effective for the growth of high crystalline MeOx layers at relative lower temperatures. As an exothermic procedure with a high rate of heat release, the necessity for high temperatures is circumvented and high purity MeOx NPs can be produced at moderate reaction conditions. In SCS process, the metal salts (e.g., nitrates) dissolved in saturated aqueous or alcoholic solutions act as oxidizing agents and react with organic fuels (such as urea, glycine, acetylacetonate, citric acid etc.) under relatively lower temperatures compared to other commonly used solution process methods to give rise to a combustion reaction and to produce the corresponding metal oxide NPs (Kim et al., 2011; Hsieh, 2014; Yu, 2015).
Iron manganite (FeMnO3, FMO) is a mixed perovskite material with the chemical formula ABO3, where the Fe atom is placed at the center of a cube formed by eight corner-sharing MnO6 octahedra (Habibi and Mosavi, 2017). FeMnO3 has been examined for applications such as lithium-ion batteries, catalysis, humidity sensors, energy storage and antibacterial devices (Doroftei et al., 2014; Cao et al., 2016; Cetin et al., 2019; Vasiljevic et al., 2020a; Nikolic et al., 2020). A large number of synthesis methods, such as co-precipitation, hydrothermal, ball milling, solid state reaction and sol-gel chemistry, have all been employed for the fabrication of FeMnO3 materials (Sundari et al., 2013; Doroftei et al., 2014; Cao et al., 2016; Soni and Pal, 2016; Bin et al., 2017; Mungse et al., 2017; Gowreesan and Ruban Kumar, 2017; Saravanakumar et al., 2018; Cetin et al., 2019; Fix, 2019; Lobo and Rubankumar, 2019; Vasiljevic et al., 2020a; Nikolic et al., 2020; Vasiljevic et al., 2020b). Despite this, not all these techniques are viable to synthesize FeMnO3 nanomaterials, as there are some drawbacks such as the expense of the source materials, chemical non-uniformity, high impurity, aggregated nanoparticles, and non-stoichiometry of some ferrite systems (Buonsanti et al., 2012; Alves et al., 2013; Bennet et al., 2016; Skliri, 2018). FeMnO3 is a semiconductor which consists of plentiful and environmentally friendly elements with an ideal direct optical bandgap (∼1.5 eV) to absorb solar photons, while it has a deep lying valence band (VB ∼ 5.3 eV) (Skliri, 2018) that corresponds well to the VB edges of several p-type materials (e.g., CuO, NiCo2O4 etc.) (Savva et al., 2017; Papadas et al., 2018b). Additionally, it has high photochemical stability which is necessary for long-term optoelectronic devices while furthermore its intrinsic electric polarization field can enable charge-carrier separation within the semiconducting structure. Such characteristics make FeMnO3 a promising light absorber for optoelectronic uses. On the other hand, between the numerous metal oxides that have been used as p-type active layers, nickel oxide (NiO) is a promising candidate for PVs due to its excellent electrochemical behavior (Kerli and Alver, 2016). NiO demonstrates a rock salt structure and exhibits adequate p-type conductivity with a wide bandgap in the range of 3.5 eV (Chrissanthopoulos et al., 2011; Mahmood et al., 2011; Zhang, 2015). NiO has been reported as p-type material in all-oxide solar cells in combination with the n-type TiO2 and ZnO materials, while also solution combustion synthesized NiOx is commonly used as HTL in perovskite solar cells (Warasawa et al., 2013; Kawade et al., 2015; Kerli and Alver, 2016; Karsthof et al., 2016; Galatopoulos et al., 2017; Patel, 2017; Ukoba et al., 2018).
In this work, SCS of FeMnO3 NPs is presented while indicating that tartaric acid can be used as a fuel and nitrate as an oxidizer agent. FeMnO3 NPs characterized by an average size of ∼13 nm and a narrow particle-size distribution, were prepared using a low cost SCS process (6 h calcination at 450°C in air). The as-synthesized FeMnO3 NPs were then functionalized with β-alanine and the ligand-capped NPs enabled the formation of compact and functional layers. These films were used, for the first time, as n-type photoactive materials and were incorporated in p-n heterojunction of all-oxide solar cells. For the purposes of this study, nanostructured NiO films were also synthesized by SCS method and applied as a p-type layer in the following structure ITO/NiO/FeMnO3/Cu. The corresponding MeOx PVs show a high Voc of 1.31 V with adequate FF of 54.3% and limited short current of 0.07 mA cm−2 resulting to a PCE of 0.05%. Electrical characterizations by impedance spectroscopy reveled a high charge recombination resistance inducing high Voc, whereas the limited current density is ascribed to the high charge transport resistance. Despite the low PCE values, these results provide a framework for further optoelectronic properties research on eco-friendly and cost-effective photoactive layers for fabrication of all solution processable inorganic photovoltaics.
Experimental
Materials: Pre-patterned glass-ITO substrates (sheet resistance 4 Ω/sq) were purchased from Psiotec Ltd. All the other chemicals used in this study were purchased from Sigma Aldrich.
Solution combustion synthesis (SCS) of FeMn O3NPs: For the synthesis of FeMnO3 NPs, 0.5 mmol Mn(NO3)2.4H2O, 0.5 mmol Fe(NO3)3.9H2O and tartaric acid were blended in 5 ml of 2-methoxy ethanol solution. Subsequently, 150 μl HNO3 (69% wt HNO3) were added slowly into the mixture, and the solution stirred up to almost complete homogeneity. The whole solution was left under stirring for at least 3 h at room temperature (RT). The molar ratio of the total metal nitrates and tartaric acid was 1. Thereafter, the precursor solution was heated at 100°C under consecutive stirring until complete evaporation of the solvent. The dry black powder was then used for the combustion synthesis of the FeMnO3 NPs in ambient atmosphere at 450°C in a preheated oven for 6 h, so that the combustion process be completed and then left to cool down at room temperature.
Perovskite FeMn O3films preparation: The prepared FeMnO3 (FMO) NP powder was used for the preparation of FMO dispersion for the deposition of corresponding film by spin coating technique. Firstly, the surface of NPs was modified with β-alanine. Briefly, as-made FMO NPs (50 mg) were added in 4 ml of deionized (DI) water containing β-alanine (10 mg), and the pH of the solution was adjusted to 4.2 with 1M HNO3. (Papadas et al., 2015b; Skliri, 2018) To secure that NPs will transfer to the liquid phase and form a stable suspension, typically within 1 day, the resulting mixture was then intensively stirred at RT. The dispersion was aided with probe sonicator for about 30 min. To a stable colloidal dispersion of 30 mg ml−1 be formed, the alanine-capped FMO NPs were isolated by centrifugation, rinsed several times with DI water, and finally dispersed in DMF. The obtained homogenous dispersion was then drop-casted and subsequently was spin coated on the top of NiO layer at 3,000 rpm for 40 s. The process of FeMnO3 film formation was repeated about ten times to obtain a desired thickness of about 500 nm. To assemble a network of tightly connected metal oxide NPs, the deposition of the FeMnO3 films was accomplished by spin coating technique of the colloidal NPs, followed by thermal annealing at 300°C for 30 min. In this way, β-alanine can enable direct NP–NP interactions upon ligand removal at growth temperature due to its small size, thus yielding high strength films consisted of firmly interconnected NP networks. This strategy is very important to obtain functional metal oxides photovoltaic devices with good charge transfer properties.
NiO NPs synthesis and films preparation by SCS: For the solution combustion synthesis of NiO, 1 mmol of Ni(NO3)2·6H2O were dissolved in 2.5 ml of 2-methoxyethanol. After the solution was stirred at 50°C for 1 h, 0.1 mmol of acetylacetone was added to the solution, and the whole solution was allowed under further stirring for 1 h at RT. Spin coating technique was applied for the fabrication of the precursor films on the various substrates. The precursor’s solution was spin coated at 3,000 rpm for 40 s. The resulting light green colored films were dried at 100°C for 5 min and used as a precursor for the combustion synthesis of NiO NPs. Subsequently the obtained films were heated at 300°C in ambient atmosphere for 1 h in a preheated hot plate to complete the combustion process and then left to cool down at room temperature, forming a ∼50 nm thin layer.
Device fabrication: The metal oxides solar cells under study were ITO/NiO-NPs/FeMnO3-NPs/Cu. ITO substrates were sonicated in acetone and subsequently in isopropanol for 10 min and then heated at 100°C on a hot plate for 10 min before use. The substrates were further treated with ozone for 10 min to achieve a better contact with the active layer by reducing the contact resistance. To fabricate the devices, a layer of NiO as p-type and FeMnO3 as n-type side of the p-n junction were formed in sequence. The deposition of corresponding metal oxides films was described in detail above. Finally, 200 nm Cu layers were thermally evaporated through a shadow mask to finalize the devices, giving an active area of 0.9 mm2.
Characterization: Thermogravimetric Analysis (TGA) were performed on a Shimadzu Simultaneous DTA-TG system (DTG-60H). Thermal analysis was conducted from 40 to 600°C in air atmosphere using air gas with a flow rate of 200 ml min−1 and a heating rate of 10°C min−1. X-ray diffraction (XRD) patterns were collected on a PANanalytical X´pert Pro MPD powder diffractometer (40 kV, 45 mA) using Cu Kα radiation (λ = 1.5418 Å). Transmission electron microscope (TEM) images and electron diffraction patterns were recorded on a JEOL JEM-2100 microscope with an acceleration voltage of 200 kV. The samples were first gently ground, suspended in ethanol, and then picked up on a carbon-coated Cu grid. Quantitative microprobe analyses were performed on a JEOL JSM-6390LV scanning electron microscope (SEM) equipped with an Oxford INCA PentaFET-x3 energy dispersive X-ray spectroscopy (EDS) detector. Data acquisition was performed with an accelerating voltage of 20 kV and 60 s accumulation time. Absorption measurements were performed with a Schimadzu UV-2700 UV-Vis spectrophotometer. For UV-VIS and PL measurements, thick films of FeMnO3 NPs have been fabricated on top of the quartz substrates employing the spin coating method. UV–vis/near-IR diffuse reflectance spectra were recorded with a Schimadzu UV-2700 UV-Vis spectrophotometer, using BaSO4 powder as a 100% reflectance standard. The energy bandgap (Eg) of the samples were estimated from Tauc plots of (Fhv)2 as a function of photon energy (hv), where F is the Kubelka–Munk function of the reflectance (R): F=(1−R)2/(2R) (Kubelka, 1948). The thickness of the films were measured with a Veeco Dektak 150 profilometer. The PL measurements were performed on FeMnO3 film on quartz substrate at an excitation wavelength of 400 nm. Photoluminescence (PL) spectrum was obtained at room temperature on a Jobin-Yvon Horiba FluoroMax-P (SPEX) spectrofluorimeter (Singapore) equipped with a 150 W Xenon lamp and operated from 300 to 900 nm. The current density-voltage (J-V) characteristics were characterized with a Botest LIV Functionality Test System. Forward bias scans were measured with 10 mV voltage steps and 40 msec of delay time. For illumination, a calibrated Newport Solar simulator equipped with a Xe lamp was used, providing an AM1.5G spectrum at 100 mW/cm2 as measured by a certified oriel 91150 V calibration cell. A shadow mask was attached to each device prior to measurements to accurately define the corresponding device area. EQE measurements were performed by Newport System, Model 70356_70316NS. Atomic force microscopy (AFM) images were obtained using a Nanosurf easy scan two controller under the tapping mode. Electrochemical Impedance Spectroscopy (EIS) and Mott-Schottky measurements were performed using a Metrohm Autolab PGSTAT 302N, where for the EIS a red light-emitting diode (LED) (at 625 nm) was used as the light source calibrated to 100 mW/cm2. For EIS a small AC perturbation of 20 mV was applied to the devices, and the different current output was measured throughout a frequency range of 1 MHz-1 Hz. The steady state DC bias was kept at 0 V throughout the EIS experiments. Mott-Schottky measurements on FeMnO3 films were performed in a 0.5 M Na2SO4 aqueous electrolyte (pH = 7) using a Metrohm Autolab PGSTAT 302N potentiostat. A three-electrode set-up, with a platinum plate (1.0 × 2.0 cm2) and a silver-silver chloride (Ag/AgCl, 3M KCl) as the counter and reference electrodes, respectively, was adopted to study the samples. The capacitance of the semiconductor/electrolyte interface was obtained at 1 kHz, with 10 mV AC voltage perturbation. All the experiments were conducted under dark conditions. The measured potential vs the Ag/AgCl reference electrode was converted to the normal hydrogen electrode (NHE) scale using the formula: ENHE = EAg/AgCl + 0.210 V. The working electrode for impedance-potential measurement was fabricated as follows, 10 mg of FeMnO3 NPs was dispersed in 1 ml DI water and the mixture was subjected to sonication in a water bath until a uniform suspension was formed. After that, 100 µl of the suspension was drop-casted onto the surface of fluorine-doped tin oxide (FTO, 9 Ω/sq) substrate, which was masked with an epoxy resin to expose an effective area of 1.0 × 1.0 cm2. The sample was dried in a 60°C oven for 30 min.
Results and Discussion
The solution processing technique provides an extensible low cost deposition procedure to fabricate high quality metal-oxide films and to replace costly and time consuming vacuum-deposition methods (Cochran et al., 2019). SCS has recently been employed in the low temperature manufacture of spinel nickel cobaltite (NiCo2O4) thin films as hole transport layers (HTLs) in inverted p-i-n perovskite solar cells (Papadas et al., 2018b). SCS possesses the benefit of speedily creating homogenous metal oxide materials of fine grain size. Most notably however, at a lower temperature than standard solid–state reaction, sol-gel and co-precipitation techniques (Chick et al., 1990; Rajeshwar and de Tacconi, 1998). Metal nitrates are distinguished for their capacity to synthesize metal oxides films of superior quality. Furthermore, it is important to choose the appropriate fuel agent for combustion, so as to circumvent the creation of sizeable clusters and particle agglomeration (Bansal, 2005; Verma et al., 2008). Tartaric acid was therefore used as the fuel agent in this work as it results in the formation of a single-crystalline phase of FeMnO3. In general, tartaric acid leads to the formation of stable heterometallic polynuclear complexes (Selbach et al., 2007) because of its carboxylate and hydroxyl groups which can bind different metal ions from the solution, such as Mn2+ and Fe3+ (Wang et al., 2011). Basically, the growth of FeMnO3 NPs is the result of combustion reaction of these polynuclear complexes while being heated in the presence of concentrated HNO3 (Patil et al., 2002b; Cochran et al., 2019).
Synthesis and Characterization FeMnO3 Nanoparticles
To achieve the solution combustion synthesis of FeMnO3 NPs, tartaric acid and metal nitrates precursors were dissolved in 2-methoxyethanol. The precursor’s solution was heated at 100°C under stirring until complete evaporation of the solvent. The obtained gel product was then analyzed by thermogravimetric analysis (TGA). The thermal behavior of the Mn/Fe-tartaric compound was observed by TGA, employing a heating rate of 10°C min−1 in ambient air. As illustrated in Figure 1A, the reaction shows an acute sudden mass loss at ∼230°C, noted in TGA curve, which is associated with a strong exothermic release of energy during the combustion process. In our study, the as-synthesized material was crystallized well to the perovskite phase in ambient atmosphere at 450°C in a preheated oven for 6 h.
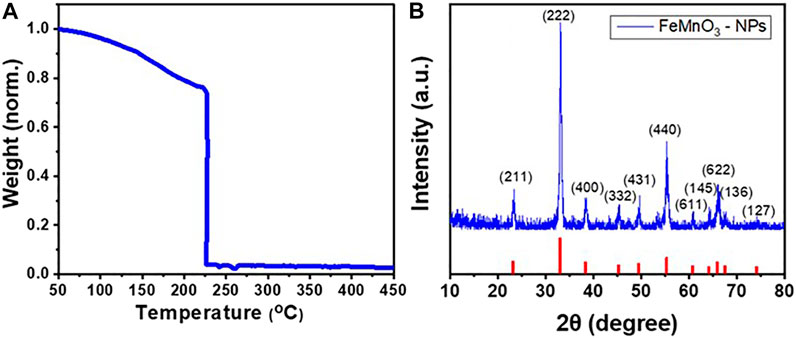
FIGURE 1. (A) TGA profile of the as-prepared FeMnO3 material synthesized via solution combustion process. The weight loss was normalized to the initial sample mass. (B) XRD pattern of FeMnO3 NPs obtained at 450°C annealing temperature.
The XRD measurement confirmed the crystallinity and phase purity of the FeMnO3 NPs produced through the SCS method. Figure 1B depicts the XRD pattern of nanocrystalline FeMnO3 obtained at 450°C soaking temperature. All the diffraction peaks compare well with the reported cubic iron manganite structure (JCPDS card #75–0,894) with a = b = c = 9.4 Å and α= β = γ = 90° unit cell parameters. No peaks from impurity phases, like MnO or Fe2O3, were observed in XRD pattern, showing the phase purity of the sample. The mean FeMnO3 crystallite size is estimated at ∼15 nm using the Scherrer`s equation and peak broadening of the (222) reflection.
TEM corroborated the phase purity of the obtained FeMnO3 NPs. Figure 2A illustrates a characteristic TEM image of the FeMnO3 sample fabricated at 450°C. It depicts that the obtained FeMnO3 consists of tightly connected NPs with an average diameter of 13 ± 2 nm in average (inset Figure 2A), which match well to the crystallite size calculated from XRD. The crystal structure of the FeMnO3 was then examined by selected-area electron diffraction (SAED). The SAED pattern recorded from a small area of the FeMnO3 sample (Figure 2B) indicates a series of broad concentric diffraction rings, which can be assigned to the cubic phase of FeMnO3 (Li et al., 2014). In line with XRD results, no other crystal phases were detected by means of electron diffraction. Furthermore, characterization of the composition of FeMnO3 with EDS analysis revealed a Fe:Mn atomic ratio close to 1:1, in agreement with the stoichiometry of FeMnO3 compound (Supplementary Figure S1).
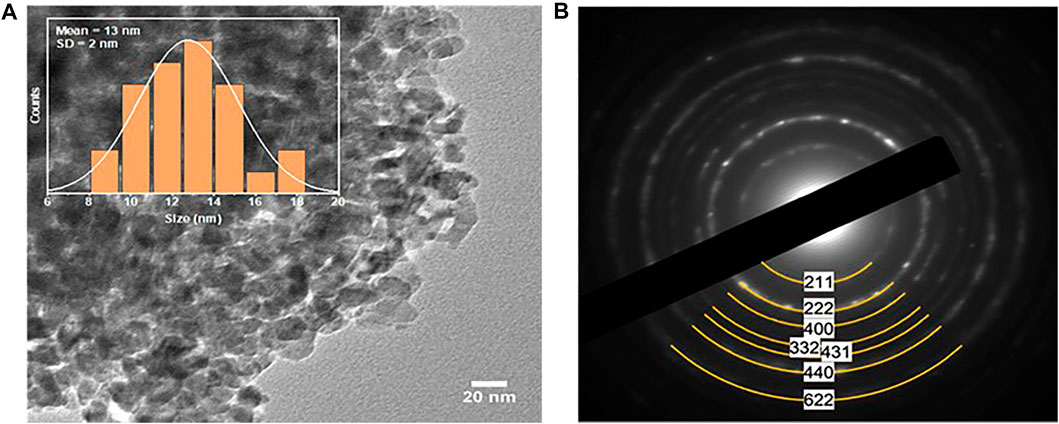
FIGURE 2. (A) Representative TEM image (inset size distribution histogram of the FeMnO3 NPs, showing an average diameter of 13 ± 2 nm), and (B) SAED pattern of the as-synthesized FeMnO3 NPs obtained at 450°C.
The electronic structure of as-prepared FeMnO3 was also examined by diffuse reflectance ultraviolet-visible/near-IR (UV-vis/NIR) spectroscopy. Figure 3A shows the UV-vis/NIR absorption spectrum for FeMnO3 NPs synthesized at 450°C by SCS. This sample shows an acute optical absorption onset in the near IR region (∼805 nm), which is associated with an energy gap at ∼1.54 eV, as determined by Tauc`s plots [(Fhv)1/2 versus photon energy (hv), where F, h, and v are the Kubelka-Munk function of the reflectance, Plank constant and light frequency, respectively] (Kubelka, 1948), see inset of Figure 3A.
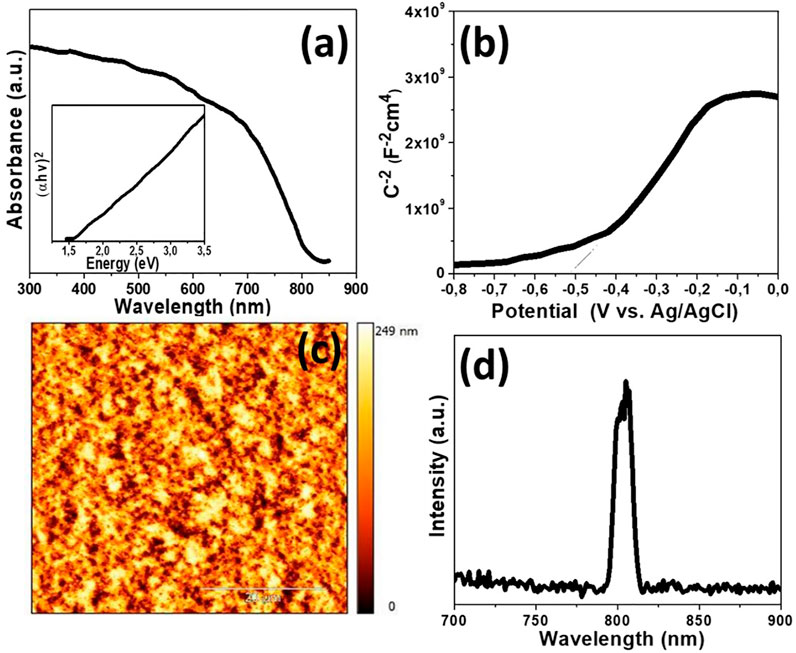
FIGURE 3. (A) UV-Vis/NIR absorption spectrum of FeMnO3 NPs [Inset: the (Fhv)2 versus hv plot derived from optical absorption spectra]. (B) Mott-Schottky plot of the inverse square space-charge capacitance (1/CSC2) as a function of applied voltage (E) relative to the redox potential of Ag/AgCl (3 M KCl) for the FeMnO3 NPs. (C) Typical AFM image of the ITO/FeMnO3 film after SCS synthesis at 450°C (The scale bar is 20 μm). (D) Room-temperature PL emission spectra of the FeMnO3 NPs.
Electrochemical impedance spectroscopy (EIS) thereafter was employed to examine the position of the conduction band (CB) and valence band (VB) edges of FeMnO3 material. Figure 3B shows the ensuing Mott-Schottky plot and the matching fit of the linear part of the inverse square space-charge capacitance (1/Csc2) as a function of potential (E). The FeMnO3 reveals a positive linear slope, showing n-type conductivity, where electrons are majority carriers. By using extrapolation to 1/CSC2 = 0, the flat-band potential (EFB) of FeMnO3 NPs was estimated to be –0.31 eV vs. NHE (pH = 7). Based on the EFB and optical bandgap (as obtained from UV-vis/NIR reflectance data, Figure 3A) values, the energy band edges for FeMnO3 NPs are CB: 3.78 eV and VB: 5.32 eV vs. vacuum (Skliri, 2018). This is further highlighted in the energy level diagram shown in Figure 4B, which is based on EIS measured values for FeMnO3 and literature data for ITO, NiO and Cu components (Haque et al., 2017). For heavily n-typed doped semiconductors, it can be supposed that the EFB level is very close to the CB edge. Generally, for several n-type semiconductors the CB edge is approximately 0.1–0.3 eV higher than the EFB potential. Therefore, the position of the VB edge was estimated from EFB–Eg. In iron manganite materials there are a number of reports which connect the electron hopping between Fe+3-Fe+2 and hole hopping between Mn+2-Mn+3 ions with n-type and p-type of conductivities (Veena Gopalan et al., 2008; Batoo et al., 2009). The findings of these studies suggest that both n-type and p-type charge carriers are anticipated to contribute to the conduction mechanism in FeMnO3 structure (Rezlescu and Rezlescu, 1974; Akhtar and Younas, 2012) In our study, the positive slope of the Mott-Schottky plots (Figure 3B) clearly shows that the perovskite iron manganite have n-type behavior (Skliri, 2018).
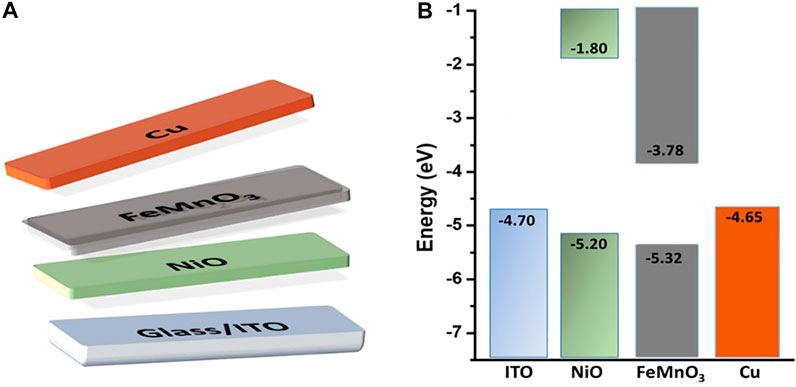
FIGURE 4. (A) Schematic representation, and (B) the corresponding energy level diagram of each component of the ITO/NiO/FeMnO3/Cu device.
The smoothness of deposited FeMnO3 film plays a crucial role for the formation of a deep depletion region, which is highly desirable for high performing devices. To achieve this β-alanine was used as surface capping ligand for FeMnO3 NPs. During this process, the carboxyl (–CO2H) groups of β-alanine adjust to the nanoparticle’s surface, whereas the amine (–NH2) functional groups inhibit the nanoparticles aggregation whilst stabilizing the colloidal solution. This resulted in the formation of a ∼500 nm thick compact film (Supplementary Figure S2) consisting of a continuous network of tightly interconnected NPs (Supplementary Figure S3) with a relative low roughness of ∼27 nm, as calculated by AFM topography measurements (Figure 3C).
For PL measurements of perovskite material, thick layers of FeMnO3 NPs were manufactured on top of the quartz substrates utilizing the spin coating method (for details, see the experimental section). PL spectroscopy is an essential tool for finding the purity and crystalline quality of semiconductors. The PL spectrum of FeMnO3 NPs, in Figure 3D, shows an intense near band edge emission at ∼805 nm. This emission peak corresponds to the CB-VB inter-band transition and no additional peaks due to the radiative relaxations from defect sites or impurities were observed in PL spectrum of FeMnO3 (Vasiljevic et al., 2020a).
Photovoltaic Device Characterization
As a proof of concept, the newly developed FeMnO3 NP aggregates were used as a n-type photoactive material in a p-n full metal oxides solar cell, with the structure ITO/NiO/FMO/Cu (Figure 4A). Both NiO and FeMnO3 materials were synthesized by the solution combustion method, as it is described in the experimental sections, rendering the fabrication process of such solar cells remarkably facile. Lastly, a 200-nm-thick Cu layer was thermally placed on the surface of FeMnO3 to complete the device (see Figure 4A).
Figure 5A shows the J–V curve of the ITO/NiO/FeMnO3/Cu device under 1 Sun simulated light (100 mW cm−2) where the curve shape evidence the Schottky barrier formation at the junctions. The extracted photovoltaic parameters, open-circuit voltage (Voc), short-circuit current (Isc), fill factor (FF), and power conversion efficiency (PCE) are listed in Table 1. The device yields a high Voc of 1.31 V with adequate FF of 54.3%, but the generated current density is low (Jsc = 0.07 mA cm−2) delivering a PCE of 0.05%.
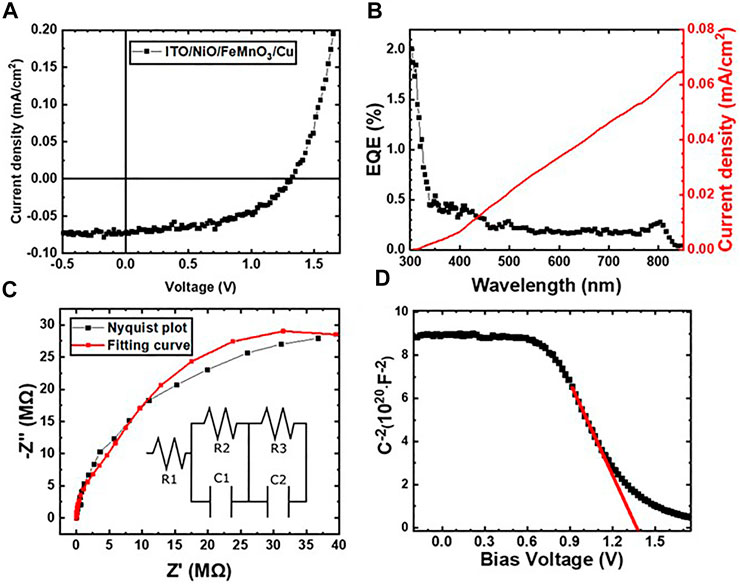
FIGURE 5. (A) J-V plot under illumination conditions for the representative p-n device under this study ITO/NiO/FeMnO3/Cu. (B) EQE spectrum of NiO−FeMnO3 (p–n) heterojunction sandwiched between ITO and Cu electrodes. The right axis represents the integrated photocurrent density of the corresponding device. (C) Nyquist, and (D) Mott–Schottky plots for the ITO/NiO/FeMnO3/Cu device.

TABLE 1. Extracted solar cell parameters from the J–V characterization of the ITO/NiO/FeMnO3/Cu device.
Among the studied all-oxide ferroic solar cells, that were fabricated by the same solution processes, the highest PCE was obtained by FeMnO3-based solar cell (∼0.05%), which is notably higher than those of the NiO/BiFeO3 (∼0.025%) (Chatterjee et al., 2014) and pure Pb(ZrTi)O3 (∼0.00008%) based solar cells (Paik et al., 2016). Furthermore, the obtained Voc of the FeMnO3-based solar cell (∼1.31 V) is also much higher with respect to the solar cells using BiFeO3 (∼0.41 V) (Chatterjee et al., 2014) and Pb(ZrTi)O3 (∼0.6 V) as a light absorber, respectively (Paik et al., 2016). Consequently, we suggest that FeMnO3 could be a potential candidate for solar cell applications.
To examine the spectral response of the device, external quantum efficiency (EQE) measurements were conducted, while the results being presented in Figure 5B along with the respective integrated photocurrent response. The spectral response of EQE reveals that the photo-generated current is produced in both NiO and FeMnO3 layers with the spectrum correspondingly match the optical absorption spectra of the respective NiO and FeMnO3 films. Specifically, we observe photocurrent generation onsets in the ultraviolent (300–350 nm) and near IR (800–850 nm) regions, which correspond to the acute optical absorption onset of NiO (∼355 nm) and FeMnO3 (∼805 nm), respectively (Tang et al., 2018). Thus, in the device structure under investigation the p-type NiO provides a small contribution to the external quantum efficiency as discussed above and for this reason the term NiO/FeMnO3-based heterojunction solar cells is used within the paper. The integrated photocurrent density (0.064 mA/cm2) is also in close accord with the value obtained from the J–V curve (0.07 mA/cm2) acquired from the solar simulator analysis.
EIS measurements were performed to obtain further insights into the depletion regions of the p−n device under study and to further understand the low generated photocurrent. Previous reported EIS measurements on lead free perovskite oxides have been performed at Voc conditions (Sariful Sheikh et al., 2017). Our trials to measure at Voc conditions resulted to low signal and high noise from moderate to high frequencies that do not enabled the analysis of EIS parameters. Thus, the presented measurements were performed under illumination and at Jsc conditions which provided adequate signal for the analysis of the EIS measurements. Figure 5C, shows characteristic Nyquist plots of the NiO/FeMnO3 heterojunction structure as well as the equivalent circuit model used to fit the experimental data; Even though the model does not perfectly much the experimental results is used to provide initial analysis of the EIS measurements presented. Specifically, the model is commonly used with the components R1, R3 and R3 being ascribed to the contacting, charge transport and recombination resistance, respectively (von Hauff, 2019). The obtained results shown in Table 2 indicate that R3 > R2 by an order of magnitude and so we can infer that the ITO/NiO/FeMnO3/Cu device exhibits a relative high recombination resistance which can explain the high Voc value, but on the other hand shows a high charge transport resistance which results in limited current density. We note that the value of recombination resistance is expected to be lower at Voc conditions due to the absence of the depletion’s layer driving force formed by the applied Jsc measuring conditions. In Figure 5D, the Mott-Schottky measurements of the title device which was swept from low to high external applied bias are illustrated. According to Mott-Schottky analysis the crossing of extrapolated linear section of the spectra with x-axis can be ascribed to the built-in potential of the device. Assuming that the proposed device is fully depleted during the measurement we exact a built-in potential of 1.38 V (von Hauff, 2019).
Overall, to achieve higher PCE from the presented solution processed NiO/FeMnO3 heterojunction inorganic photovoltaics, further research and material development methods are needed. The main limiting factor that must be addressed is the improvement of n-type FeMnO3 charge transport properties and thickness optimization of the active layer to decrease the charge carrier recombination. In parallel, incorporation of buffer layers within the ITO/NiO/FeMnO3/Cu device structure can be used to improved charge carrier selectivity as well as a more appropriate p-type material can be applied with higher light harvesting capabilities and better aligned VB level edge to n-type FeMnO3 to facilitate the holes transfer. The above proposed research and material development efforts can result to higher PCE for solution processed based heterojunction inorganic photovoltaics.
Conclusion
This study successfully proves that the synthesis of FeMnO3 NPs can be achieved by a solution combustion technique, using tartaric acid as a fuel. Furthermore, the ultimate control of the nanoparticle’s size can be readily attained due to the multiple binding ability of the tartaric acid that resulted in the formation of single-phase FeMnO3 with an average particle size of 13 ± 2 nm. X-ray diffraction and electron microscopy measurements verified the high phase purity and crystallinity of FeMnO3. Additionally, we used a method to construct a network of tightly connected FeMnO3 nanoparticles by spin coating of the colloidal solution. In this study, β-alanine was used as surface capping agent to produce a stable colloidal dispersion of FeMnO3 NPs (β-alanine-capped FeMnO3 NPs) in DMF. The short chain length of β-alanine allows direct interactions between the nanoparticles through ligand removal by thermal annealing (at 450°C in air), thus yielding a thick absorbing film (∼500 nm) consisting of continuous layers of interconnected nanoparticles and exhibiting a relative low roughness of ∼27 nm. The proposed strategy is crucial to obtain functional all-oxide photovoltaic devices that are developed using a process technique based on a simple solution. Furthermore, the inorganic perovskite FeMnO3 was tested as a light absorber for photovoltaic applications for the first time. The band gap (∼1.54 eV) of the synthesized FeMnO3 nanostructure was found to be very close to the hybrid lead perovskite CH3NH3PbI3 material (∼1.55 eV). To this end, all-inorganic NiO/FeMnO3 heterojunction photovoltaics were fabricated by solution combustion synthesis, using spin coating techniques. The corresponding all-inorganic solar cells reveal a high open circuit voltage (Voc) of 1.31 V with a fill factor (FF) of 54.3% but exhibit a PCE of 0.05% under 100 mW cm−2 illumination due to the limited short circuit current 0.07 mA cm−2. Electrical characterization by impedance spectroscopy showed that the ITO/NiO/FeMnO3/Cu device exhibits a high recombination resistance justifying the high Voc. The high charge transport resistance indicates charge transport limitations within the relative thick (∼500 nm) n-type FeMnO3 active layer. Optimizing the active layer thickness and improving the charge carrier transport properties are the main limiting processes of the n-type FeMnO3 which results in low Jsc values for the presented solution processed based heterojunction inorganic photovoltaics. Moreover, further PCE improvement could be achieved by the incorporation of suitable charge selective contacts within the solar cell device architecture and the replacement of NiO with a more appropriate p-type material. The obtained results encourage more intense research on solution processed and environmentally friendly inorganic solar cells with suitable opto-electronic properties and high photon to electron conversion efficiency.
Data Availability Statement
The raw data supporting the conclusion of this article will be made available by the authors, without undue reservation.
Author Contributions
IP, AI, and SC conceived the idea of the presented synthesis of controllable monodispersed FeMnO3 nanoparticles and examined it as n-type photoactive layer in all-inorganic NiO/FeMnO3 solution processed heterojunction photovoltaics. GA and IP design the nanoparticulate metal oxide materials. AI, PE, and SC designed the device experimental laboratory procedures. IP and IV performed the synthesis and material characterization studies. AI fabricated the devices, performed device characterization measurements and analyzed the device performance data under the supervision of SC and PE. All authors discussed the results and commented on the manuscript. SC supervised the project.
Funding
This research was funded by the European Research Council (ERC) under the European Union’s Horizon 2020 research and innovation program (Grant agreement No 647311) and further supported from the academic yearly research activity internal Cyprus University of Technology budget.
Conflict of Interest
The authors declare that the research was conducted in the absence of any commercial or financial relationships that could be construed as a potential conflict of interest.
Publisher’s Note
All claims expressed in this article are solely those of the authors and do not necessarily represent those of their affiliated organizations, or those of the publisher, the editors and the reviewers. Any product that may be evaluated in this article, or claim that may be made by its manufacturer, is not guaranteed or endorsed by the publisher.
Supplementary Material
The Supplementary Material for this article can be found online at: https://www.frontiersin.org/articles/10.3389/fchem.2021.754487/full#supplementary-material
References
Cetin, A., Önal, A. M., and Esenturk, E. N., “Nanowires assembled from iron manganite nanoparticles : Synthesis , characterization , and investigation of electrocatalytic properties for water oxidation reaction,” pp. 1–9, 2019, doi:10.1557/jmr.2019.215
Akhtar, M. J., and Younas, M. (2012). Structural and transport properties of nanocrystalline MnFe 2O 4 synthesized by co-precipitation method. Solid State. Sci. 14 (10), 1536–1542. doi:10.1016/j.solidstatesciences.2012.08.026
Alves, A., Bergmann, C. P., and Berutti, F. A. (2013). Novel Synthesis and Characterization of Nanostructured Materials. Berlin: Springer.
Bansal, N. P. (2005). “Combustion Synthesis of Sm0.5Sr0.5CoO3-x and La0.6Sr0.4CoO3-x Nanopowders for Solid Oxide Fuel Cell Cathodes,” in Conference Sixth Pacific Rim Conference on Ceramic and Glass Technology sponsored by the American Ceramic Society - 2005 Maui, Hawaii: NASA/TM—2005-213897 Available at: http://gltrs.grc.nasa.gov/.
Batoo, K. M., Kumar, S., Lee, C. G., and Alimuddin, A. (2009). Study of dielectric and ac impedance properties of Ti doped Mn ferrites. Curr. Appl. Phys. 9 (6), 1397–1406. doi:10.1016/j.cap.2009.03.012
Bennet, J., Tholkappiyan, R., Vishista, K., Jaya, N. V., and Hamed, F. (2016). Attestation in self-propagating combustion approach of spinel AFe 2 O 4 (A = Co, Mg and Mn) complexes bearing mixed oxidation states: Magnetostructural properties. Appl. Surf. Sci. 383, 113–125. doi:10.1016/j.apsusc.2016.04.177
Bin, H., Yao, Z., Zhu, S., Zhu, C., Pan, H., Chen, Z., et al. (2017). A high-performance anode material based on FeMnO3/graphene composite. J. Alloys Compd. 695, 1223–1230. doi:10.1016/j.jallcom.2016.10.249
Buonsanti, R., Pick, T. E., Krins, N., Richardson, T. J., Helms, B. A., and Milliron, D. J. (2012). Assembly of ligand-stripped nanocrystals into precisely controlled mesoporous architectures. Nano Lett. 12 (7), 3872–3877. doi:10.1021/nl302206s
Calnan, S. (2014). Applications of oxide coatings in photovoltaic devices. Coatings 4 (1), 162–202. doi:10.3390/coatings4010162
Cao, K., Liu, H., Xu, X., Wang, Y., and Jiao, L., (2016). FeMnO3, “A high-performance Li-ion battery anode material. Chem. Commun. 52 (76), 11414–11417. doi:10.1039/c6cc04891a
Chatterjee, S., Bera, A., and Pal, A. J. (2014). p-i-n heterojunctions with BiFeO3 perovskite nanoparticles and p-and n-type oxides: Photovoltaic properties. ACS Appl. Mater. Inter. 6 (22), 20479–20486. doi:10.1021/am506066m
Chick, L. A., Pederson, L. R., Maupin, G. D., Bates, J. L., Thomas, L. E., and Exarhos, G. J. (1990). Glycine-nitrate combustion synthesis of oxide ceramic powders. Mater. Lett. 10 (1–2), 6–12. doi:10.1016/0167-577X(90)90003-5
Chrissanthopoulos, A., Baskoutas, S., Bouropoulos, N., Dracopoulos, V., Poulopoulos, P., and Yannopoulos, S. N. (2011). Synthesis and characterization of ZnO/NiO p-n heterojunctions: ZnO nanorods grown on NiO thin film by thermal evaporation. Photon. Nanostructures - Fundam. Appl. 9 (2), 132–139. doi:10.1016/j.photonics.2010.11.002
Cochran, E. A., Woods, K. N., Johnson, D. W., Page, C. J., and Boettcher, S. W. (2019). Unique chemistries of metal-nitrate precursors to form metal-oxide thin films from solution: materials for electronic and energy applications. J. Mater. Chem. A. 7 (42), 24124–24149. doi:10.1039/C9TA07727H
Deganello, F., Marcì, G., and Deganello, G. (2009). Citrate-nitrate auto-combustion synthesis of perovskite-type nanopowders: A systematic approach. J. Eur. Ceram. Soc. 29 (3), 439–450. doi:10.1016/j.jeurceramsoc.2008.06.012
Doroftei, C., Dorin, P., Rezlescu, E., and Rezlescu, N. (2014). Composites: Part B Structural and catalytic characterization of nanostructured iron manganite. Compos. PART B 67, 179–182. doi:10.1016/j.compositesb.2014.07.005
Ellabban, O., Abu-Rub, H., and Blaabjerg, F. (2014). Renewable energy resources: Current status, future prospects and their enabling technology. Renew. Sust. Energ. Rev. 39, 748–764. Nov. 2014. doi:10.1016/j.rser.2014.07.113
Skliri, E., “Assembly and photochemical properties of mesoporous networks of spinel ferrite nanoparticles for environmental photocatalytic remediation,” Appl. Catal. B Environ., vol. 227, no. pp. 330–339. 2018, doi:10.1016/j.apcatb.2018.01.045
Fu, Q., Tang, X., Huang, B., Hu, T., Tan, L., Chen, L., et al. (2018387May 2018). Recent Progress on the Long-Term Stability of Perovskite Solar Cells. Adv. Sci. 5 (5), 1700387. doi:10.1002/advs.201700387
Galatopoulos, F., Savva, A., Papadas, I. T., and Choulis, S. A. (2017). The effect of hole transporting layer in charge accumulation properties of p-i-n perovskite solar cells. APL Mater. 5 (7), 076102. doi:10.1063/1.4991030
Gowreesan, S., and Ruban Kumar, A. (2017). Structural, magnetic, and electrical property of nanocrystalline perovskite structure of iron manganite (FeMnO3). Appl. Phys. A. Mater. Sci. Process. 123 (11), 1–8. doi:10.1007/s00339-017-1302-x
Grinberg, I., West, D. V., Torres, M., Gou, G., Stein, D. M., Wu, L., et al. (2013). Perovskite oxides for visible-light-absorbing ferroelectric and photovoltaic materials. Nature 503 (7477), 509–512. Nov. doi:10.1038/nature12622
Habibi, M. H., and Mosavi, V. (2017). Urea combustion synthesis of nano-structure bimetallic perovskite FeMnO3 and mixed monometallic iron manganese oxides: effects of preparation parameters on structural, opto-electronic and photocatalytic activity for photo-degradation of Basic Blue 12. J. Mater. Sci. Mater. Electron. 28 (12), 8473–8479. doi:10.1007/s10854-017-6568-4
Han, C.-H., Han, S.-D., Gwak, J., and Khatkar, S. P. (2007). Synthesis of indium tin oxide (ITO) and fluorine-doped tin oxide (FTO) nano-powder by sol-gel combustion hybrid method. Mater. Lett. 61 (8–9), 1701–1703. Apr. 2007. doi:10.1016/j.matlet.2006.07.114
Haque, M. A., Sheikh, A. D., Guan, X., and Wu, T. (2017). Metal Oxides as Efficient Charge Transporters in Perovskite Solar Cells. Adv. Energ. Mater. 7 (20), 1602803. doi:10.1002/aenm.201602803
Hsieh, H. H. (2014). Novel approaches for fabricating high-performance low-temperature solution-processed metal oxide transistors. Dig. Tech. Pap. - SID Int. Symp. 45 (1), 427–430. doi:10.1002/j.2168-0159.2014.tb00115.x
Hussein, A. K. (2015). Applications of nanotechnology in renewable energies-A comprehensive overview and understanding. Renew. Sust. Energ. Rev. 42, 460–476. doi:10.1016/j.rser.2014.10.027
Ioakeimidis, A., Papadas, I. T., Tsikritzis, D., Armatas, G. S., Kennou, S., and Choulis, S. A. (2019). Enhanced photovoltaic performance of perovskite solar cells by Co-doped spinel nickel cobaltite hole transporting layer. APL Mater. 7 (2), 021101. doi:10.1063/1.5079954
Jadhav, L. D., Patil, S. P., Chavan, A. U., Jamale, A. P., and Puri, V. R. (2011). Solution combustion synthesis of Cu nanoparticles: a role of oxidant-to-fuel ratio. Micro Nano Lett. 6 (9), 812–815. doi:10.1049/mnl.2011.0372
Jiang, Q., Lu, J., Cheng, J., Li, X., Sun, R., Feng, L., et al. (2014). Combustion-process derived comparable performances of Zn-(In:Sn)-O thin-film transistors with a complete miscibility. Appl. Phys. Lett. 105 (13), 132105. doi:10.1063/1.4896990
Jung, E. H., Jeon, N. J., Park, E. Y., Moon, C. S., Shin, T. J., Yang, T.-Y., et al. (2019). Efficient, stable and scalable perovskite solar cells using poly(3-hexylthiophene). NatureMar 567 (7749), 511–515. doi:10.1038/s41586-019-1036-3
Karsthof, R., Räcke, P., Von Wenckstern, H., and Grundmann, M. (2016). Semi-transparent NiO/ZnO UV photovoltaic cells. Phys. Status Solidi Appl. Mater. Sci. 213 (1), 30–37. doi:10.1002/pssa.201532625
Kawade, D., Moriyama, K., Nakamura, F., Chichibu, S. F., and Sugiyama, M. (2015). Fabrication of visible-light transparent solar cells composed of NiO/NixZn1-xO/ZnO heterostructures. Phys. Status Solidi Curr. Top. Solid State. Phys. 12 (6), 785–788. doi:10.1002/pssc.201400256
Kerli, S., and Alver, U. (2016). Preparation and characterisation of ZnO/NiO nanocomposite particles for solar cell applications. J. Nanotechnol. 2016. doi:10.1155/2016/4028062
Kim, H., Gilmore, C. M., Piqué, A., Horwitz, J. S., Mattoussi, H., Murata, H., et al. (1999). Electrical, optical, and structural properties of indium-tin-oxide thin films for organic light-emitting devices. J. Appl. Phys. 86 (11), 6451–6461. doi:10.1063/1.371708
Kim, M.-G., Kanatzidis, M. G., and Facchetti, A., (2011). Marks, “Low-temperature fabrication of high-performance metal oxide thin-film electronics via combustion processing SI. Nat. Mater. 10 (5), 382–388. doi:10.1038/nmat3011
Kosten, E. D., Atwater, J. H., Parsons, J., Polman, A., and Atwater, H. A. (2013). Highly efficient GaAs solar cells by limiting light emission angle. Light Sci. Appl. 2 (1), e45. doi:10.1038/lsa.2013.1
Kubelka, P. (1948). New Contributions to the Optics of Intensely Light-Scattering Materials Part I. J. Opt. Soc. Am. 38 (5), 448. doi:10.1364/JOSA.38.000448
Li, F., and Liu, M. (2017). Recent efficient strategies for improving the moisture stability of perovskite solar cells. J. Mater. Chem. A. 5 (30), 15447–15459. doi:10.1039/C7TA01325F
Li, M., Xu, W., Wang, W., Liu, Y., Cui, B., and Guo, X. (2014). Facile synthesis of specific FeMnO3 hollow sphere/graphene composites and their superior electrochemical energy storage performances for supercapacitor. J. Power Sourc. 248, 465–473. doi:10.1016/j.jpowsour.2013.09.075
Lobo, L. S., and Rubankumar, A. (2019). Investigation on structural and electrical properties of FeMnO 3 synthesized by sol-gel method. Ionics (Kiel). 25 (3), 1341–1350. doi:10.1007/s11581-018-2776-z
Mahmood, T., Saddique, M. T., Naeem, A., Westerhoff, P., Mustafa, S., and Alum, A. (2011). Comparison of Different Methods for the Point of Zero Charge Determination of NiO. Ind. Eng. Chem. Res. 50 (17), 10017–10023. doi:10.1021/ie200271d
Mimani, T., and Patil, K. C. (2001). Solution Combustion Synthesis of Nanoscale Oxides and Their Composites. Mater. Phys. Mech. 4, 134–137. doi:10.1016/j.materresbull.2008.09.034
Mitzi, D. B., Gunawan, O., Todorov, T. K., Wang, K., and Guha, S. (2011). The path towards a high-performance solution-processed kesterite solar cell. Solar Energ. Mater. Solar Cell 95 (6), 1421–1436. Jun. 2011. doi:10.1016/j.solmat.2010.11.028
Patel, M., “Excitonic metal oxide heterojunction (NiO/ZnO) solar cells for all-transparent module integration,” Sol. Energ. Mater. Sol. Cell, vol. 170, no. June, pp. 246–253. 2017. doi:10.1016/j.solmat.2017.06.006
Sariful Sheikh, M., Ghosh, D., Dutta, A., Bhattacharyya, S., and Sinha, T. P., “Lead free double perovskite oxides Ln2NiMnO6 (Ln = La, Eu, Dy, Lu), a new promising material for photovoltaic application,” Mater. Sci. Eng. B Solid-state Mater. Adv. Technol., vol. 226, no. pp. 10–17. 2017. doi:10.1016/j.mseb.2017.08.027
Mungse, P. B., Saravanan, G., Nishibori, M., and Subrt, J. (2017). “Solvent-Free, Improved Synthesis of Pure Bixbyite Phase of Iron and Manganese Mixed Oxides as Low-cost, Potential Oxygen Carrier for Chemical Looping with Oxygen Uncoupling,” in Conference 12th Conference on Solid State Chemistry - 2016, Prague, Czech Republic. doi:10.1515/pac-2016-1127
Nikolic, M. V., Krstic, J. B., Labus, N. J., Lukovic, M. D., Dojcinovic, M. P., Radovanovic, M., et al. “Structural, morphological and textural properties of iron manganite (FeMnO3) thick films applied for humidity sensing,” Mater. Sci. Eng. B Solid-state Mater. Adv. Technol., vol. 257, no, p. 114547, 2020, doi:10.1016/j.mseb.2020.114547
Nechache, R., Harnagea, C., Li, S., Cardenas, L., Huang, W., Chakrabartty, J., et al. (2014). Bandgap tuning of multiferroic oxide solar cells. Nat. Photon 9 (1), 61–67. doi:10.1038/nphoton.2014.255
Paik, Y. H., Kojori, H. S., Yun, J.-H., and Kim, S. J. (2016). Improved efficiency of ferroelectric Pb(Zr, Ti)O3 (PZT) based photovoltaic device with colloidal quantum dots. Mater. Lett. 185, 247–251. doi:10.1016/j.matlet.2016.08.082
Papadas, I., Christodoulides, J. A., Kioseoglou, G., and Armatas, G. S. (2015). A high surface area ordered mesoporous BiFeO3 semiconductor with efficient water oxidation activity. J. Mater. Chem. A. 3 (4), 1587–1593. doi:10.1039/C4TA05272B
Papadas, I. T., Galatopoulos, F., Armatas, G. S., Tessler, N., and Choulis, S. A. (2019). Nanoparticulate Metal Oxide Top Electrode Interface Modification Improves the Thermal Stability of Inverted Perovskite Photovoltaics. Nanomaterials 9 (11), 1616. Nov. doi:10.3390/nano9111616
Papadas, I. T., Ioakeimidis, A., Armatas, G. S., and Choulis, S. A. (2018). Low-Temperature Combustion Synthesis of a Spinel NiCo2 O4 Hole Transport Layer for Perovskite Photovoltaics. Adv. Sci. 5 (5), 1701029–9. doi:10.1002/advs.201701029
Papadas, I. T., Savva, A., Ioakeimidis, A., Eleftheriou, P., Armatas, G. S., and Choulis, S. A. (2018). Employing surfactant-assisted hydrothermal synthesis to control CuGaO2 nanoparticle formation and improved carrier selectivity of perovskite solar cells. Mater. Today Energ. 8, 57–64. doi:10.1016/j.mtener.2018.03.003
Papadas, I. T., Subrahmanyam, K. S., Kanatzidis, M. G., and Armatas, G. S. (2015). Templated assembly of BiFeO 3 nanocrystals into 3D mesoporous networks for catalytic applications. Nanoscale 7 (13), 5737–5743. doi:10.1039/C5NR00185D
Patil, K. C., Aruna, S. T., and Mimani, T. (2002). Combustion synthesis: An update. Curr. Opin. Solid State. Mater. Sci. 6 (6), 507–512. doi:10.1016/S1359-0286(02)00123-7
Patil, K. C., Aruna, S. T., and Mimani, T. (2002). Combustion synthesis: An update. Curr. Opin. Solid State. Mater. Sci. 6 (6), 507–512. doi:10.1016/S1359-0286(02)00123-7
Pérez-Tomás, A., Mingorance, A., Tanenbaum, D., and Lira-Cantú, M. (2018). Metal Oxides in Photovoltaics: All-Oxide, Ferroic, and Perovskite Solar Cells. Amsterdam: Elsevier.
Pérez-Tomas, A., Xie, H., Wang, Z., Kim, H.-S., Shirley, I., Turren-Cruz, S.-H., et al. (2019). PbZrTiO3 ferroelectric oxide as an electron extraction material for stable halide perovskite solar cells. Sust. Energ. Fuels 3 (2), 382–389. doi:10.1039/c8se00451j
Rajeshwar, K., and de Tacconi, N. R. (1998). Solution combustion synthesis of oxide semiconductors for solar energy conversion and environmental remediation. Chem. Soc. Rev. 38 (7), 1984. doi:10.1039/b811238j
Rezlescu, N., and Rezlescu, E. (1974). Dielectric properties of copper containing ferrites. Phys. Status Solidi 23 (2), 575–582. doi:10.1002/pssa.2210230229
Rohatgi, A. (1996). “Record high 18.6% efficient solar cell on HEM multicrystalline material,” in Conference Record of the Twenty Fifth IEEE Photovoltaic Specialists Conference - 1996 (New Jersey, 741–744. doi:10.1109/PVSC.1996.564236
Saravanakumar, B., Ramachandran, S. P., Ravi, G., Ganesh, V., Guduru, Ramesh. K., and Yuvakkumar, R. (2018). Electrochemical characterization of FeMnO3 microspheres as potential material for energy storage applications. Mater. Res. Express 5, 015504. doi:10.1088/2053-1591/aaa3b1
Savva, A., Papadas, I., Tsikritzis, D., Armatas, G. S., Kennou, S., and Choulis, S. (2017). Room Temperature Nanoparticulate Interfacial Layers for Perovskite Solar Cells via Solvothermal Synthesis. J. Mater. Chem. A. 5, 20381–20389. doi:10.1039/C7TA03802J
Selbach, S. M., Einarsrud, M.-A., Tybell, T., and Grande, T. (2007). Synthesis of BiFeO 3 by Wet Chemical Methods. J. Am. Ceram. Soc. 90 (11), 3430–3434. doi:10.1111/j.1551-2916.2007.01937.x
Seo, J.-Y., Uchida, R., Kim, H.-S., Saygili, Y., Luo, J., Moore, C., et al. (2018). Boosting the Efficiency of Perovskite Solar Cells with CsBr-Modified Mesoporous TiO2 Beads as Electron-Selective Contact. Adv. Funct. Mater. 28 (15), 1705763. doi:10.1002/adfm.201705763
Shin, S. S., Lee, S. J., and Seok, S. I. (2019). Exploring wide bandgap metal oxides for perovskite solar cells. APL Mater. 7 (2), 022401. doi:10.1063/1.5055607
Soni, D., and Pal, R. (2016). Nanocrystalline Manganese Iron Oxide as a C,harge Storage Electrode. Electroanalysis 28 (9), 1951–1956. doi:10.1002/elan.201600031
Sun, Y., Guo, F., Chen, J., and Zhao, S. (2017). Improved ferroelectric and photovoltaic properties of BiMnO3 modified lead-free K0.5Na0.5NbO3 solid-solution films. Appl. Phys. Lett. 111 (25), 253901. doi:10.1063/1.5006643
Sundari, R., Hua, T. I., and Rusli Yosfiah, M. (2013). Effect of calcinations on characterizations of fabricated nano manganese ferrite. Adv. Mater. Res. 634 (1), 2150–2154. doi:10.4028/www.scientific.net/AMR.634-638.2150
Suresh, K., Kumar, N. R. S., and Patil, K. C. (1991). A novel combustion synthesis of spinel ferrites, orthoferrites and garnets. Adv. Mater., 3, 148–150. doi:10.1002/adma.19910030306
Tang, L. J., Chen, X., Wen, T. Y., Yang, S., Zhao, J. J., Qiao, H. W., et al. (2018). A Solution-Processed Transparent NiO Hole-Extraction Layer for High-Performance Inverted Perovskite Solar Cells. Chem. - A. Eur. J. 24 (12), 2845–2849. doi:10.1002/chem.201705658
Ukoba, K. O., Inambao, F. L., and Eloka-Eboka, A. C. (2018). Fabrication of affordable and sustainable solar cells using NiO/TiO2 P-N heterojunction. Int. J. Photoenergy 2018. doi:10.1155/2018/6062390
Vasiljevic, Z. Z., Dojcinovic, M. P., Krstic, J. B., and Ribic, V. (2020). Synthesis and antibacterial activity of iron manganite (FeMnO3) particles against the environmental bacterium Bacillus subtilis. Bacillus subtilis, 13879. 13888. doi:10.1039/d0ra01809k
Vasiljevic, Z. Z., Dojcinovic, M. P., Krstic, J. B., Ribic, V., Tadic, N. B., Ognjanovic, M., et al. (2020). Synthesis and antibacterial activity of iron manganite (FeMnO3) particles against the environmental bacterium: Bacillus subtilis. RSC Adv. 10 (23), 13879–13888. doi:10.1039/d0ra01809k
Veena Gopalan, E., Malini, K. A., Saravanan, S., Sakthi Kumar, D., Yoshida, Y., and Anantharaman, M. R. (2008). Evidence for polaron conduction in nanostructured manganese ferrite. J. Phys. D. Appl. Phys. 41 (18), 185005. doi:10.1088/0022-3727/41/18/185005
Verma, S., Joshi, H. M., Jagadale, T., Chawla, A., Chandra, R., and Ogale, S. (2008). Nearly Monodispersed Multifunctional NiCo 2 O 4 Spinel Nanoparticles: Magnetism, Infrared Transparency, and Radiofrequency Absorption. J. Phys. Chem. C 112 (39), 15106–15112. doi:10.1021/jp804923t
von Hauff, E. (2019). Impedance Spectroscopy for Emerging Photovoltaics. J. Phys. Chem. C 123 (18), 11329–11346. doi:10.1021/acs.jpcc.9b00892
Wang, W. (2014). Device characteristics of CZTSSe thin-film solar cells with 12.6% efficiency. Adv. Energ. Mater. 4 (7), 1–5. doi:10.1002/aenm.201301465
Wang, Y., Hu, Y., Fei, L., Zhang, Y., Yuan, J., and Gu, H. (2011). Synthesis of bismuth ferrite nanoparticles via a wet chemical route at low temperature. J. Nanomater. 2011. doi:10.1155/2011/797639
Warasawa, M., Watanabe, Y., Ishida, J., Murata, Y., Chichibu, S. F., and Sugiyama, M. (2013). Fabrication of visible-light-transparent solar cells using p-type nio films by low oxygen fraction reactive rf sputtering deposition. Jpn. J. Appl. Phys. 52 (2). doi:10.7567/JJAP.52.021102
Xiong, L., Guo, Y., Wen, J., Liu, H., Yang, G., Qin, P., et al. (2018). Review on the Application of SnO2 in Perovskite Solar Cells. Adv. Funct. Mater. 28 (35), 1802757. doi:10.1002/adfm.201802757
Yu, X. (2015). Spray-combustion synthesis: efficient solution route to high-performance oxide transistors. Proc. Natl. Acad. Sci. U. S. A. 112 (11), 3217–3222. doi:10.1073/pnas.1501548112
Zhang, G., Wu, H., Li, G., Huang, Q., Yang, C., Huang, F., et al. (2013). New high Tc multiferroics KBiFe2O5 with narrow band gap and promising photovoltaic effect. Sci. Rep. 3 (1), 1265. doi:10.1038/srep01265
Keywords: inorganic perovskites, solution combustion synthesis, FeMnO3, NiO, p-n junction, functional metal oxides, inorganic solar cells, photoactive nanomaterials
Citation: Papadas IT, Ioakeimidis A, Vamvasakis I, Eleftheriou P, Armatas GS and Choulis SA (2021) All-Inorganic p−n Heterojunction Solar Cells by Solution Combustion Synthesis Using N-type FeMnO3 Perovskite Photoactive Layer. Front. Chem. 9:754487. doi: 10.3389/fchem.2021.754487
Received: 06 August 2021; Accepted: 13 September 2021;
Published: 29 September 2021.
Edited by:
Konstantinos Christoforidis, Democritus University of Thrace, GreeceReviewed by:
Dimitris Tsikritzis, Hellenic Mediterranean University, GreeceDimitris A. Chalkias, University of Peloponnese, Greece
Copyright © 2021 Papadas, Ioakeimidis, Vamvasakis, Eleftheriou, Armatas and Choulis. This is an open-access article distributed under the terms of the Creative Commons Attribution License (CC BY). The use, distribution or reproduction in other forums is permitted, provided the original author(s) and the copyright owner(s) are credited and that the original publication in this journal is cited, in accordance with accepted academic practice. No use, distribution or reproduction is permitted which does not comply with these terms.
*Correspondence: Ioannis T. Papadas, aW9hbm5pcy5wYXBhZGFzQGN1dC5hYy5jeQ==; Stelios A. Choulis, c3RlbGlvcy5jaG91bGlzQGN1dC5hYy5jeQ==