- State Key Laboratory of Bioactive Substances and Function of Natural Medicine, Institute of Materia Medica, Peking Union Medical College and Chinese Academy of Medical Sciences, Beijing, China
In this study, we evaluated the applicability of various superoxide anion sensors which were designed based on either redox or non-redox mechanisms. Firstly, both redox- and non–redox-based superoxide anion probes were designed and synthesized using either coumarin or chromone as the fluorophores, and the photophysical properties of these probes were measured. Subsequently, the sensing preference of both types of probes toward various reactive oxygen species (ROS) was evaluated. We found that non–redox-based O2•− probes exhibited broad sensing ability toward various ROS. By contrast, redox based O2•− probes showed a clear reactivity hierarchy which was well correlated to the oxidizing strength of the ROS. Lastly, the detection selectivity of redox-based O2•− recognizing probes was also observed when balancing various factors, such as reactant ROS concentrations, temperature, and changing reaction transformation rates. Herein, we concluded the selectivity advantage of redox-based O2•− probes.
Introduction
Reactive oxygen species (ROS) are a group of important oxidizing agents within biological systems, which play a key role in the regulation of homeostasis (Juan et al., 2021; Yang et al., 2019; D’Autréaux and Toledano, 2007). The concentrations of ROS generally remain balanced, and any interruption of this balance results in a cascade of unwanted biological events (Yang et al., 2019; Juan et al., 2021). Therefore, in the clinical setting, it is of utmost importance to accurately detect the concentrations of these ROS, as well as probe the underlying biological mechanism of this dysregulation.
The oxygen of ROS is in a highly oxidizing state, which results in all ROS being highly reactive toward a range of biological substances (Jiao et al., 2018). Thus, ROS are usually found in low concentrations in tissues under regular conditions, and therefore traditionally, it has been difficult to accurately quantify the concentration of ROS. The mitochondria and NADPH oxidase produce the major ROS, superoxide anion, and H2O2 in the cells (Dröge, 2002; Woolley et al., 2013). Usually, under normal conditions, the concentration of superoxide anion and H2O2 is estimated to be about 10−10 and 5 × 10−9 M, respectively (Dröge, 2002; Turrens, 2003; Woolley et al., 2013). However, the concentration of these at the cellular level can change in a wide range under stimulated conditions. Moreover, the oxidizing state of ROS ranges from 2 to 0, with degradation from high-oxidizing ROS forming additional low-oxidizing ROS (Jiao et al., 2018). Therefore, several ROS might coexist within a single system, and it will be important to distinguish each ROS during detection (Jiao et al., 2018). Among the ROS, the oxygen of the superoxide anion is in the highest oxidation state, and the superoxide anion is the precursor to several other ROS (Cadenas and Davies, 2000; Jiao et al., 2018). Thus, in regard to superoxide anion detection, selectivity would be a key parameter to be considered.
To this end, various methods have been developed for ROS detection, including the fluorescent dye method, nanoprobe technology, electrochemical biosensors, electron spin resonance method, genetic encoded ROS reporter, and others (Woolley et al., 2013; Mamone et al., 2016) Among them, fluorescent techniques have been widely used in sensing and detecting these biologically important species under certain biological conditions(Fuloria et al., 2021; Duanghathaipornsuk et al., 2021; Wu et al., 2019; Cheng et al., 2019). To date, a range of fluorescent sensors have been developed for various ROS (Chen et al., 2016; Yang et al., 2020). For O2•− sensors, based on design principles, these can be classified into two categories: redox and non-redox mechanisms–based O2•− fluorescent probes (Figure 1) (Jiao et al., 2018; Xiao et al., 2020). The redox-based fluorescent probes have been designed based on the oxidizing ability of O2•− (Tang et al., 2004; Zhang et al., 2013; Wang et al., 2020), and the non–redox-based fluorescent probes were designed on the nucleophilicity or other inherent reactivity of O2•− (Maeda et al., 2005; Xu et al., 2007; Zielonka et al., 2010). Although these descriptors exist, there has been no systematic study to understand their key differences in relation to their applicability, particularly, their sensing selectivity.
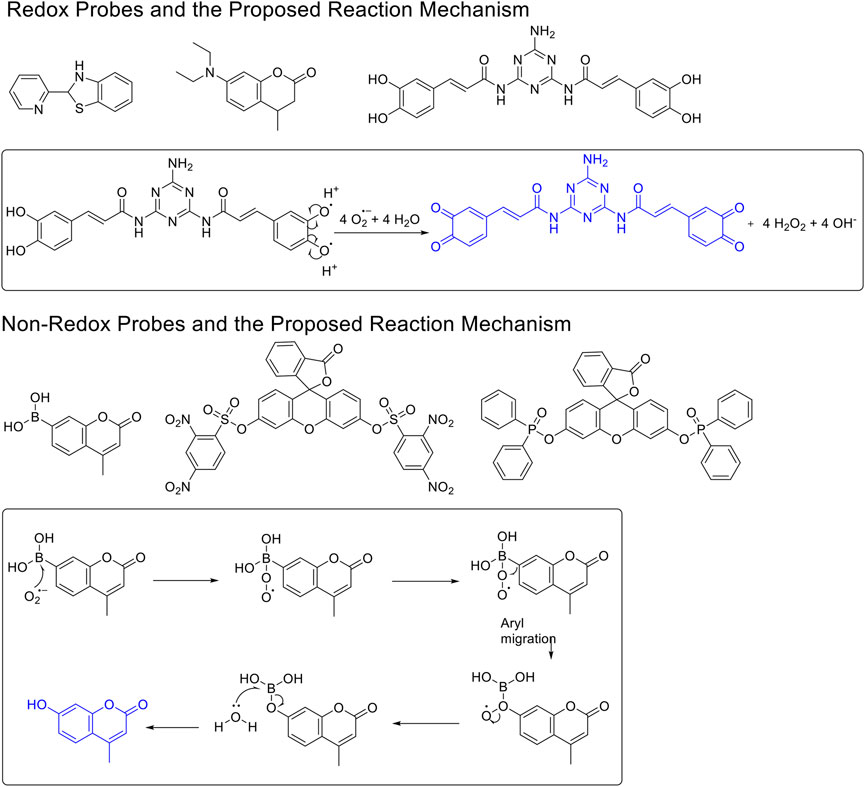
FIGURE 1. The redox- and non–redox-based superoxide anion probes. The reaction mechanisms of the selected probes were also proposed to help to understand this study.
Materials and Methods
Chemical Synthesis General
1H-NMR and 13C-NMR spectra were recorded with a Varian Mercury 400 or 500 spectrometer using tetramethylsilane as the internal standard in methanol-d4, DMSO-d6, or chloroform-d. High-resolution mass spectrometry (HRMS) data were measured on a Thermo Exactive Orbitrap Plus spectrometer. Liquid chromatography–mass spectrometry (LC-MS) was conducted on an Agilent 1100 series HPLC and an Agilent LC/MSD TOF. All of the solvents and chemicals were purchased from commercial sources: Sigma-Aldrich Chemical Co., Beijing Ou-he Reagents Co., Beijing Shiji-Aoke Biotechnology Co., and Shanghai Jingke Chemistry Technology Co. with a purity of more than 95% (LC-MS). All chemicals and solvents used were of reagent grade without further purification or drying before use. All the reactions were monitored by thin-layer chromatography (TLC) under a UV lamp at 254 nm. Column chromatography separations were performed using silica gel (200–300 mesh).
General Procedure for Preparation of Compounds R1–R3
To a solution of compound 1 or 2 or 3 (1.40 mmol) in absolute alcohol (10 ml), palladium on carbon (10% Pd/C, 10 wt% of the compound 1 or 2 or 3) was added and the suspension was hydrogenated (1 atm, balloon) at RT for 22 h. TLC indicated the completion. The suspension was filtered through a pad of Celite and the filtered solid was rinsed with ethyl acetate (3 × 10 ml). The combined filtrate and rinses were concentrated. The products R1–R3 were purified by silica gel column chromatography.
7-Hydroxy-4-methylchroman-2-one (R1). White solid, yield 23.5%. 1H-NMR (400 MHz, methanol-d4): δ 7.09 (d, J = 8.2 Hz, 1H), 6.58 (dd, J = 8.2, 2.2 Hz, 1H), 6.45 (d, J = 2.1 Hz, 1H), 3.10 (dd, J = 12.2, 6.0 Hz, 1H), 2.83 (dd, J = 15.6, 5.0 Hz, 1H), 2.53 (dd, J = 15.6, 6.7 Hz, 1H), 1.26 (d, J = 6.8 Hz, 3H). 13C-NMR (100 MHz, methanol-d4): δ 170.64, 158.72, 153.22, 128.37, 120.11, 112.75, 104.56, 37.94, 30.03, 20.53. HRMS (ESI): m/z calculated for C10H11O3 (M + H)+, 179.07027; found, 179.07010.
7-Amino-4-methylchroman-2-one (R2). White solid, yield 15.6%. 1H-NMR (400 MHz, methanol-d4): δ 6.99 (d, J = 8.2 Hz, 1H), 6.49 (dd, J = 8.1, 2.1 Hz, 1H), 6.38 (d, J = 2.1 Hz, 1H), 3.12–2.97 (m, 1H), 2.81 (dd, J = 15.7, 5.5 Hz, 1H), 2.51 (dd, J = 15.7, 7.0 Hz, 1H), 1.24 (d, J = 6.9 Hz, 3H). 13C-NMR (100 MHz, methanol-d4): δ 171.05, 153.25, 149.46, 128.14, 118.15, 112.78, 104.03, 38.19, 30.00, 20.61. HRMS (ESI): m/z calculated for C10H12NO2 (M + H)+, 178.08626; found, 178.08673.
7-(Diethylamino)-4-methylchroman-2-one (R3). Colorless oily liquid, yield 30.0%. 1H-NMR (400 MHz, DMSO-d6): δ 7.01 (d, J = 8.6 Hz, 1H), 6.39 (dd, J = 8.5, 2.6 Hz, 1H), 6.24 (d, J = 2.6 Hz, 1H), 3.26 (q, J = 7.0 Hz, 4H), 3.05–2.94 (m, 1H), 2.77 (dd, J = 15.7, 5.5 Hz, 1H), 2.45 (dd, J = 15.7, 7.5 Hz, 1H), 1.15–1.08 (m, 3H), 1.04–0.96 (m, 6H). 13C-NMR (100 MHz, DMSO-d6): δ 169.04, 152.48, 147.95, 127.62, 114.46, 108.15, 99.46, 44.21 (2C), 37.36, 28.20, 20.55, 12.80 (2C). HRMS (ESI): m/z calculated for C14H20NO2 (M + H]+, 234.14886; found, 234.14853.
General Procedure for the Preparation of Compounds Ra–Rc
The compound 4H-chromen-4-one derivative 4 or 5 or 6 (0.41 mmol) was added to dry THF (15 ml), and then the mixture was stirred and cooled to −20°C. A solution of LiAlH4 (0.45 ml, 1.0 M solution in THF) diluted with 5 ml dry THF was added dropwise to the above with stirring at −20°C. The mixture was stirred for 2 h at −20°C. The reaction was analyzed by TLC for completion. Then the reaction was quenched with 2 M NH4Cl aqueous solution (20 ml), and the solvent was removed in vacuo. The mixture was extracted with ethyl acetate (3 × 20 ml), and the combined organic layers were washed with saturated NaCl aqueous solution (2 × 20 ml). The organic layer was then dried (Na2SO4), filtered, and the solvent was removed in vacuo. The products Ra–Rc were purified by silica gel column chromatography.
7-Hydroxy-3-methylchroman-4-one (Ra). White solid, yield 46.2%. 1H-NMR (400 MHz, methanol-d4): δ 7.69 (d, J = 8.7 Hz, 1H), 6.47 (dd, J = 8.6, 2.0 Hz, 1H), 6.29 (d, J = 1.9 Hz, 1H), 4.47 (dd, J = 11.2, 5.0 Hz, 1H), 4.10 (t, J = 10.7 Hz, 1H), 2.84–2.65 (m, 1H), 1.15 (d, J = 7.0 Hz, 3H). 13C-NMR (100 MHz, methanol-d4): δ 196.05, 166.37, 165.58, 130.14, 114.52, 111.64, 103.46, 73.48, 41.50, 11.24. HRMS (ESI): m/z calculated for C10H11O3 (M + H)+, 179.07027; found, 179.07027.
7-Amino-3-methylchroman-4-one (Rb). Yellow solid, yield 29.3%. 1H-NMR (400 MHz, methanol-d4): δ 7.56 (d, J = 8.7 Hz, 1H), 6.29 (dd, J = 8.7, 2.1 Hz, 1H), 6.06 (d, J = 2.1 Hz, 1H), 4.41 (dd, J = 11.1, 4.8 Hz, 1H), 4.05 (dd, J = 11.1, 9.6 Hz, 1H), 2.67 (qd, J = 9.6, 7.1, 4.9 Hz, 1H), 1.15 (d, J = 7.1 Hz, 3H). 13C-NMR (100 MHz, methanol-d4): δ 195.64, 165.75, 158.12, 130.01, 111.47, 110.39, 99.84, 73.28, 41.32, 11.71. HRMS (ESI): m/z calculated for C10H12NO2 (M + H)+, 178.08626; found, 178.08649.
7-(Azetidin-1-yl)-3-propylchroman-4-one (Rc). White solid, yield 60.3%. 1H-NMR (400 MHz, DMSO-d6): δ 7.55 (d, J = 8.6 Hz, 1H), 6.07 (dd, J = 8.7, 2.1 Hz, 1H), 5.76 (d, J = 2.1 Hz, 1H), 4.43 (dd, J = 11.3, 4.4 Hz, 1H), 4.17 (dd, J = 11.3, 8.1 Hz, 1H), 3.92 (t, J = 7.4 Hz, 4H), 2.46 (h, J = 3.2 Hz, 1H), 2.33 (p, J = 7.3 Hz, 2H), 1.72–1.57 (m, 1H), 1.44–1.37 (m, 1H), 1.33 (ddd, J = 13.1, 9.2, 5.6 Hz, 2H), 0.88 (t, J = 7.1 Hz, 3H). 13C-NMR (100 MHz, DMSO-d6): δ 191.99, 163.07, 156.62, 128.73, 110.65, 105.66, 95.99, 70.51, 51.61, 44.84, 29.02, 20.13, 16.28, 14.49. HRMS (ESI): m/z calculated for C15H20O2N (M + H]+, 246.14886; found, 246.14819.
General Procedure for Preparation of Compounds N1, N2
To a solution of compound 10 or 11 (0.75 mmol) in 1,4-dioxane (3 ml), Et3N (304 mg, 3.00 mmol) and PdCl2 (dppf) (23 mg, 0.03 mmol) were added. Then 5,5,5′,5′-tetramethyl-2,2′-bi (1,3,2-dioxaborinane) (509 mg, 2.25 mmol) was added dropwise to the above with stirring. The mixture was stirred and heated to 120°C and refluxed. The reaction was analyzed by TLC for completion. The mixture was cooled to room temperature and 3 ml saturated NH4Cl aqueous solution added. The mixture was extracted with ethyl acetate (3 × 10 ml), and the combined organic layers were washed with saturated NaCl aqueous solution (2 × 10 ml). The organic layer was then dried (Na2SO4), filtered, and the solvent was removed in vacuo. The products N1, N2 were purified by silica gel column chromatography.
7-(5,5-Dimethyl-1,3,2-dioxaborinan-2-yl)-4H-chromen-4-one (N1). White solid, yield 75.6%. 1H-NMR (400 MHz, chloroform-d): δ 8.17 (d, J = 7.9 Hz, 1H), 7.89–7.85 (m, 2H), 7.80 (d, J = 7.8 Hz, 1H), 6.35 (d, J = 5.6 Hz, 1H), 3.81 (s, 4H), 1.04 (s, 6H). 13C-NMR (100 MHz, chloroform-d): δ 177.98, 156.10, 155.50, 129.99, 126.19, 124.58, 123.63, 116.59, 112.99, 72.46 (2C), 31.92, 21.85 (2C). HRMS (ESI): m/z calculated for C14H16O4B (M + H)+, 259.11362; found, 259.11300.
7-(5,5-Dimethyl-1,3,2-dioxaborinan-2-yl)-3-propyl-4H-chromen-4-one (N2). White solid, yield 82.2%. 1H-NMR (400 MHz, chloroform-d): δ 8.18 (d, J = 7.9 Hz, 1H), 7.85 (s, 1H), 7.76 (t, J = 3.9 Hz, 2H), 3.80 (s, 4H), 2.49–2.41 (m, 2H), 1.62 (dd, J = 14.9, 7.4 Hz, 2H), 1.04 (s, 6H), 0.97 (t, J = 7.3 Hz, 3H). 13C-NMR (100 MHz, chloroform-d): δ 178.14, 156.03, 152.22, 152.04, 129.46, 125.20, 124.65, 124.47, 123.54, 72.44 (2C), 31.91, 27.87, 21.86 (2C), 21.55, 13.83. HRMS (ESI): m/z calculated for C17H22O4B (M + H)+, 301.16057; found, 301.16030.
General Procedure for Preparation of Compounds N3, N4
To a solution of compound 1 or 9 (0.57 mmol) in dry DCM (10 ml), DIPEA (220 mg, 1.70 mmol) and 2,4-dinitrobenzenesulfonyl chloride (151 mg, 0.57 mmol) were added. The mixture was stirred at room temperature. The reaction was analyzed by TLC for completion, and the products N3 and N4 were purified by silica gel column chromatography.
4-Methyl-2-oxo-2H-chromen-7-yl 2,4-dinitrobenzenesulfonate (N3). White solid, yield 45.6%. 1H-NMR (400 MHz, DMSO-d6): δ 9.12 (d, J = 2.2 Hz, 1H), 8.61 (dd, J = 8.7, 2.3 Hz, 1H), 8.32 (d, J = 8.7 Hz, 1H), 7.85 (d, J = 8.8 Hz, 1H), 7.34 (d, J = 2.4 Hz, 1H), 7.23 (dd, J = 8.7, 2.4 Hz, 1H), 6.46 (s, 1H), 2.42 (s, 3H). 13C-NMR (100 MHz, DMSO-d6): δ 159.60, 154.00, 153.00, 152.10, 150.44, 148.61, 134.13, 131.04, 128.15, 127.97, 121.75, 120.05, 118.61, 115.43, 110.93, 18.64. HRMS (ESI): m/z calculated for C16H11N2O9S (M + H)+, 407.01798; found, 407.01721.
6-Methoxy-4-oxo-3-propyl-4H-chromen-7-yl 2,4-dinitrobenzenesulfonate (N4). White solid, yield 55.2%. 1H-NMR (400 MHz, chloroform-d): δ 8.90 (d, J = 2.7 Hz, 1H), 8.32 (dd, J = 9.2, 2.7 Hz, 1H), 7.77 (s, 2H), 7.31 (s, 1H), 6.94 (d, J = 9.2 Hz, 1H), 3.85 (s, 3H), 2.52–2.39 (m, 2H), 1.70–1.59 (m, 2H), 0.99 (t, J = 7.4 Hz, 3H). 13C-NMR (100 MHz, chloroform-d) δ 176.65, 155.10, 152.23, 151.09, 148.59, 146.01, 141.92, 139.31, 128.76, 124.31, 122.73, 122.21, 117.95, 111.88, 107.48, 56.55, 27.77, 21.50, 13.80. HRMS (ESI): m/z calculated for C19H17N2O10S (M + H)+, 465.05984; found, 465.05988.
Measurement of Photophysical Properties of the Probes
The photophysical properties of all compounds were measured. The measurement of the photophysical properties of various compounds was carried out as we described before (Miao et al., 2015; Wen et al., 2018; Yan et al., 2018). All compounds were dissolved in 0.1 M Tris-HCl buffer, pH 8.0 at the concentration of 10 μM. SHIMADU UV-2700, UV-visible spectrophotometer was used to measure UV-visible spectra. HITACHI F-7000 fluorescence spectrophotometer was used to measure excitation and emission spectra. For fluorescence quantum yield calculation, compounds were dissolved in 0.1 M Tris-HCl buffer (pH 8.0) at the concentration of 0.5 μg/ml or less using quinine sulfate (0.5 μg/L in 0.1 M H2SO4, Ф = 0.54) as a reference (Williams et al., 1983).
Detection of the pH Stability of the Probes
We also investigated the stability of these probes under various pHs. 0.2 M phosphate buffers with desired pHs (pH 3, pH 7, and pH13) were prepared. A 10 μM solution of each probe at different pHs (pH 3, pH 7, and pH13) was prepared, and their fluorescence was scanned (Ex, Em) to see the change.
Determination of the Reactivity Between Fluorescent Probes and Various ROS
Various ROS were also prepared as literature in 0.1 M phosphate buffer, 0.15 M NaCl, pH 7.4, or anhydrous DMSO (Wang et al., 2020; Chen et al., 2021). Each probe was dissolved in these ROS solutions at the final concentration of 10 μM. After being incubated at 37°C for 5 min, the mixture was scanned for the preferred Ex of the desired fluorophore to check if the desired fluorophore was formed. In order to detect the fluorescence change, the Ex was set as 340 nm, and the Em was measured between 380 and 600 nm. In addition, the final products of the reactions were also analyzed by LC-MS for confirmation (ESI S2).
Fluorescence Response of Various Probes Toward XO/HPX System
The enzymatic assay was performed in 0.1 M HEPES buffer, pH 7.4. Please refer to our previous publications (Wang et al., 2020). Initially, we began this study at a relatively low concentration of 0.25 U/ml XO enzyme and observed a real-time fluorescence change for non–redox-based probes N1, N2, N3, and N4. However, under these conditions, we did not observe a fluorescence change for redox-based probes R1, R2, and R3. We further increased the concentration of the XO enzymes (0.6 U/ml) to produce more O2•− in the system and observed a relatively low fluorescence increase for probes R2 and R3, but no fluorescence change was observed for probe R1.
Results and Discussion
Synthesis of Various 3,4-Dihydrocoumarin and Chromanone-Derived Probes
Series 1
The synthesis of probes R1–R3 is depicted in Scheme 1. Compounds 1, 2, and 3 are coumarin derivatives and were prepared as described previously (Wang et al., 2020; Chen et al., 2021). 1, 2, and 3 were reduced to probes R1–R3 via hydrogenation, employing 10% Pd/C.

SCHEME 1. The synthetic route of compounds R1, R2, R3. Reagent and conditions: 10% Pd/C, H2, EtOH, RT, Yield: 23.5% (R1), 15.6% (R2), 30% (R3).
Series 2
The synthetic routes of compounds Ra, Rb, and Rc are outlined in Scheme 2. Three chromones 4, 5, and 6 were prepared as previously described (Chen et al., 2021). For the reduction, we used LiAlH4 to reduce the chromones to yield the desired chromanones under a low temperature.

SCHEME 2. The synthetic routes of compounds Ra, Rb, Rc. Reagent and conditions: LiAlH4, dry THF, −20°C. Yield: 46.2% (Ra), 29.3% (Rb), 60.3% (Rc).
Series 3
The synthetic strategy towards N1 and N2 is shown in Scheme 3. Compounds 10 and 11 are commercially available. The compounds N1 and N2 were obtained following a previously reported Miyaura borylation protocol (Jana et al., 2014).
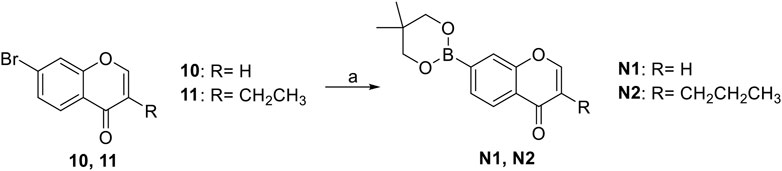
SCHEME 3. The synthetic route of compounds N1, N2. Reagent and conditions: (a) Et3N, (dppf)PdCl2, 1,4-dioxane, 2-(2,2-dimethyl-1,3,5-dioxaborinan-5-yl)-5,5-dimethyl-1,3,2-dioxaborinane, 120°C, reflux, Yields: 75.6% (N1) and 82.2% (N2).
Series 4
The synthesis of N3 and N4 is shown in Scheme 4 and was achieved via a simple one-step procedure from the corresponding phenol and sulfonyl chloride. Compound 1 (100 mg, 0.568 mmol) or compound 9 (100 mg, 0.427 mmol) reacted with 2,4-dinitrobenzene-1-sulfonyl chloride (151 mg, 0.568 mmol) in anhydrous DCM (10 ml) and DIPEA (1.704 mmol, 3 eq). The mixture was stirred at room temperature. The reaction was analyzed by TLC for completion. The yields of N3 and N4 were 45.6% or 55.2%.

SCHEME 4. The synthetic routes of N3 and N4. Reagent and conditions: (a) DIPEA, dry DCM, RT, Yield: 45.6% (N3) and 55.2% (N4).
We, therefore, set out to conduct this study. First, we worked to synthesize ten O2•- sensors of both redox- and non–redox-based probes (Figure 2 and Table 1), and 7-donor coumarin and 7-donor chromone were chosen as the fluorophoric portion of the molecule. Coumarin is a known fluorophore and has been frequently used in various studies to a high level of success (Cao et al., 2019). Additionally, chromone derivatives have also been found to exhibit a range of interesting fluorescent properties (Miao et al., 2015; Chen et al., 2021). Series 1 (R1, R2, R3) and series 2 (Ra, Rb, Rc) were designed as redox mechanism–based probes. For these two series, the broken aromatization of either coumarin or chromone quenched the fluorescence. For the detection, the oxidation of them by certain ROS was expected to recover the aromatization of the coumarin or chromone, and this would in accordance turn on the fluorescence (Doura et al., 2012; Wang et al., 2020). Series 3 (N1, N2) and series 4 (N3, N4) are the probes that were designed with a non-redox mechanism, and the 7-donor groups were modified with the boronate group (series 3) or sulfonyl ester group (series 4). The modification of the 7-donor group of the coumarin and chromone broke the electron transfer between donor and p-conjugated-acceptor, and this dramatically quenched the fluorescence of probes. For the detection, certain ROS will react with the probes to either replace or remove the modified group, and finally turn on the fluorescence (Maeda et al., 2005; Castro-Godoy et al., 2019).
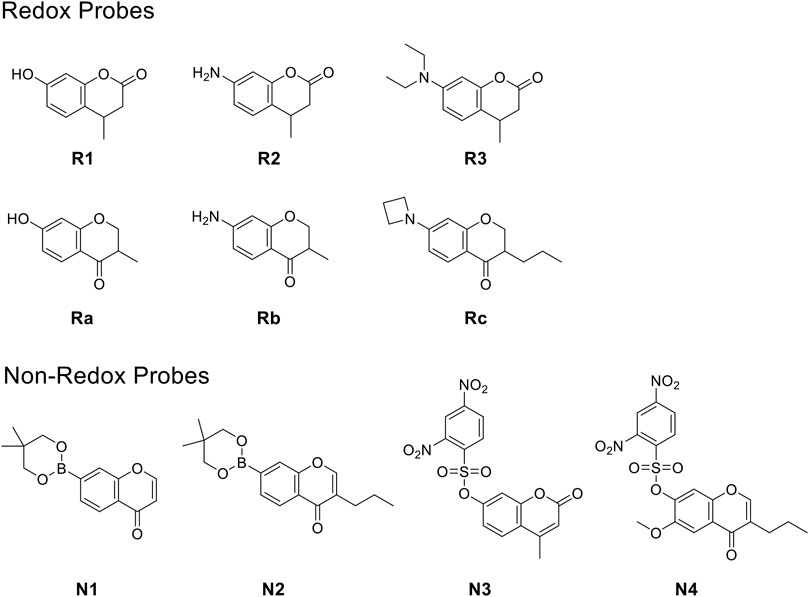
FIGURE 2. The redox- and non–redox-based superoxide anion probes designed and synthesized in this study. Coumarin and chromone were selected as the core fluorophores.
Measurement of Photophysical Properties and pH Stability of the Probes
Next, we measured the photophysical properties of all probes (Table 1). As expected, the majority of the fluorophores (1–9) exhibited moderate to high quantum yields, while the designed probes (R1–N4) had relatively low quantum yields (0.01–0.11). Moreover, given that the fluorescence intensity of a compound corresponds to the quantum yield and the molar extinction coefficient, we calculated the turn-on ratio for each matched pair of probe and fluorophore. As expected, the majority of the synthesized probes have a useful fluorescence turn-on ratio ranging from 10 to several hundred, and are therefore perfectly suited to being used as fluorescence turn-on probes. We further investigated the stability of these probes under various pHs (Figure 3). A 10 μM solution of each probe at different pHs (pH 3, pH 7 and pH13) was prepared and their fluorescence was measured. We can see that redox based O2•− probes (series 1, 2) have stable fluorescence intensity in various buffers at differing pH. For the non-redox based O2•− probes, we observed a stable but low fluorescence intensity of series 3 in various buffers (pH 3, pH 7, and pH13). However, we found that probes of series 4 (N3 and N4) exhibited strong fluorescence intensity in a basic buffer (pH13), which was 30–40 times stronger than that of other buffers (pH3 and pH7). This suggests that probes N3 and N4 degraded under basic conditions to turn on the fluorescence. Since N3 and N4 were designed to react with ROS to remove the sulfonyl ester group via nucleophilic substitution, the OH- group in a basic buffer can also react with them to eliminate the modification on 7-hydroxyl (Tampieri et al., 2019). In summary, redox based O2•− probes are rather stable at differing pHs. However, in basic conditions, some non-redox based O2•− probes might turn the fluorescence on by OH- group via nucleophilic substitution.
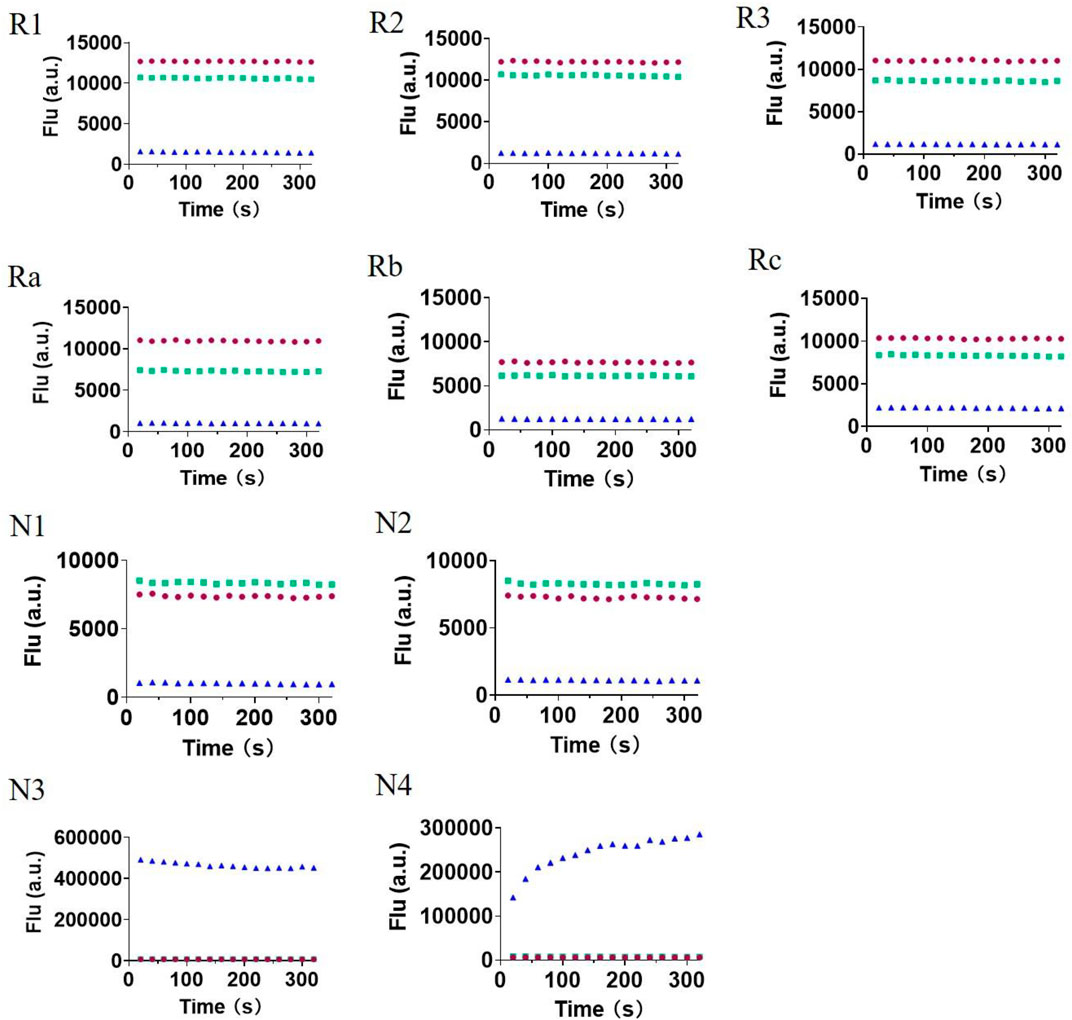
FIGURE 3. The fluorescence intensity of all 10 probes (R1–N4) in various phosphate buffers with different pHs (pH3, red; pH7, green; and pH13, blue). Once the compounds were dissolved, fluorescence intensity was immediately measured for several minutes.
Determination of the Reactivity Between Fluorescent Probes and Various ROS
Now that we have a primary understanding of the probes, we would like to explore the reactivity between the probes and various ROS [e.g., tert-butyl hydroperoxide(TBHP), H2O2, •OH, 1O2, ClO−, O2•−] (Table 2, ESI). Probes were incubated with various oxidizing agents (TBHP, H2O2, •OH, 1O2, ClO−) in 0.1 M phosphate buffer with 0.15 M NaCl (pH 7.4) at 37°C for 5 min (Doura et al., 2012; Xing et al., 2016; Zhan et al., 2017). Because O2•− cannot exist in an aqueous buffer, the reaction between the probes and O2•− was carried out in anhydrous DMSO at 37°C for 5 min (Wang et al., 2020). The concentrations of the probes were set as 10 μM, but the amounts of various ROS were excessive to promote the reaction (ESI). It was agreed that if the desired fluorophore was detected, regardless of the reaction transformation rate, the reactivity between the probe and ROS would be deemed successful in the study. The results (Table 2) showed that under our conditions: (1) R2 was the most reactive probe in series 1, which can react with 1O2, ClO−, and O2•−. The other two probes, R1 and R3, can only react with ClO− and O2•−. (2) Interestingly, chromone-derived probes (series 2) showed much lower reactivity when compared to series 1, and we unfortunately only saw the reactivity between Rb and O2•−. (3) However, we observed that the probes from series 3 and 4 were much more reactive toward various ROS than series 1 and 2. The boronate probes (N1 and N2) were successful in sensing TBHP, H2O2, 1O2, •OH, ClO−, and O2•−. The sulfonyl ester (N3 and N4) successfully reacted with TBHP, H2O2, and O2•−, but not with 1O2, •OH, and ClO−.
In this section, in order to rank the reactivity hierarchy toward various ROS among probes, we used excessive ROS to react with each probe. Generally speaking, redox-based O2•− probes exhibited a strong reactivity hierarchy which was well correlated to the oxidizing state of the ROS. The reactivity order of redox-based O2•− probes is R2 > R3 > R1 > Rb > Ra, Rc. Interestingly, although similar reaction mechanisms (the aromatization) were used for series 1 and series 2, series 1 (coumarin derivatives) was more active than series 2 (chromone derivatives) toward various ROS. This indicates that both the reactive group and the structure of the chosen fluorophore affected the reactivity of the probes. This provides the opportunity to further optimize the reactivity of these probes via structural modification. Unfortunately, the non–redox-based probes reacted with almost all ROS without any clear correlation to the oxidizing ability of the ROS. This broad ROS reactivity obviously limits the application of these types of probes.
Exploration of Detection Selectivity and Applicability of the Redox Based O2•− Probes
Next, we explored the selectivity profiles of redox-based O2•− probes (series 1 and 2). The selectivity of the probe was not only determined by the reactivity but also affected by the transformation rate. The transformation rate can be manipulated by adjusting ROS concentration, reaction temperature, and others. For example, in this study, we found that chromone-derived probe Rb can only react with O2•− but not with other ROS although we further increased the ROS concentration, reaction time, and temperature. Thus, probe Rb was highly specific to O2•−. On the other hand, when we balanced the conditions of ROS concentration, and reaction temperature, the transformation rate between the probe and certain ROS was subsequently changed. If we control the transformation rate to allow the number of reaction products to be below or above the detection line, we can achieve detection selectivity. In this study, we proved the reactivity between R3 and ClO−/O2•−. However, if we set the incubation time for less than 5 min at 37°C, probe R3 can only turn the fluorescence on by O2•− but not by ClO− (ESI S1.5). Thus, probe R3 can selectively detect O2•− under certain conditions.
Lastly, we explored the applicability of our redox-based O2•− probes in a biological system. The O2•− was produced via the more biologically relevant xanthine oxidase (XO)/hypoxanthine (HPX) system (Figure 4). We began this study at a relatively low concentration of XO enzyme (0.25 U/ml) and 1 mM HPX. We observed a real-time fluorescence change for non–redox-based probes N1, N2, N3, and N4. The sulfonyl ester series (N3, N4) was particularly active under the XO/HPX system, and the fluorescence quickly reached a peak level after several minutes. While the boronate series (N1, N2) was successful, it was much slower than the sulfonyl ester series (Figure 4A). However, under this enzyme condition, we did not observe a fluorescence change for all redox-based O2•− probes (series 1 and 2). We further increased the concentration of the XO enzymes (0.6 U/ml) to produce more concentrated O2•− in the system. Then, we observed a slow fluorescence increase for probes R2 and R3 (Figure 4B), but no fluorescence change was observed for probes R1, Ra, Rb, and Rc.
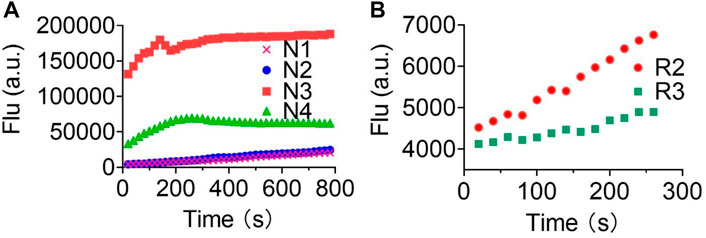
FIGURE 4. Fluorescence responses of various probes in the XO/HPX system. (A), non–redox-based O2•− probes: N1, N2, N3, and N4. (B), redox-based O2•− probes: R2 and R3. Time course for the change in fluorescence intensity observed with various probes. 50 μM various probes N1, N2, N3 and N4 were dissolved in 0.1 mM HEPES buffer in 0.25 U/ml XO and 1 mM HPX, pH 7.4. 50 μM various probes R2 and R3 were dissolved in 0.1 mM HEPES buffer in 0.6 U/ml XO and 1 mM HPX, pH 7.4. Fluorescence intensity was measured with the preference Ex and Em of the probes.
Previous studies have shown that O2•− reacts violently with H2O (Wang et al., 2020; Tampieri et al., 2019). Thus, in the XO/HPX system, the majority of the produced O2•− will react with excessive H2O before they can reach the probes. The reaction between O2•− and H2O produces H2O2 and OH- (Tampieri et al., 2019), both of which remain relatively stable in the aqueous solution. This will eventually cause the solution contain high concentrations of H2O2 and OH-, but rather low concentrations of freshly produced O2•−. Interestingly, we proved that non–redox-based O2•− probes can react with almost all oxidizing levels of ROS (Table 2). Thus, both newly produced O2•− and the degraded low oxidizing ROS can react with non-redox O2•− probes to turn on the fluorescence. Notably, we also showed that probes of series 4 (the sulfonyl ester) but not series 3 (the boronate) can react with OH- to turn on the fluorescence (Figure 3) (Tampieri et al., 2019), this was accordingly reflected as probes of series 4 were more reactive than those of series 3 in the XO/HPX system (Figure 4A). By contrast, in the XO/HPX system, only O2•− but not other low oxidizing ROS can turn on the fluorescence of redox-based O2•− probes (series 1 and 2). Thus, redox-based O2•− probes reacted more slowly toward O2•− in the XO/HPX system (Figure 4). When we further increased the concentration of the XO enzyme, a small portion of R2 and R3 slowly turned on the fluorescence. Taken together, we found that in the XO/HPX system, non–redox-based O2•− probes were more active than redox-based ones. Unfortunately, most of the non–redox-based O2•− probes unselectively turned on fluorescence by the low oxidizing level of ROS. By contrast, redox-based O2•− probes R2 and R3 can only be slowly oxidized by O2•− to turn on fluorescence, and the fluorescence change was directly caused by O2•− but not other low oxidizing ROS. In summary, we showed the advantage of redox-based O2•− probes in the detection of O2•− in a biological system.
Conclusion
In this study, we explored the difference between redox- and non–redox-based superoxide anion probes. We found that redox-based probes showed clear detection preference correlating with the oxidation ability of the ROS, with non–redox-based probes reacting unselectively with a range of ROS. This indicated that further efforts to develop O2•− sensors should pay attention to the redox-based mechanism. Interestingly, for the same type of redox-based probe, the detection selectivity toward superoxide anion can be optimized through the modification of the structure of the fluorophore, which will eventually provide the community with sensitive and highly selective sensors for O2•−.
Data Availability Statement
The fluorescence responses of all reactions, the LC-MS characterizations of the reactions, 1H and 13C NMR spectra for all final compounds can be found in the Supplementary Material.
Author Contributions
YW, SJ, and ZY carried out the experiments: detection, synthesis. HW contributed to the synthesis. HC designed the study. YW and HC wrote the manuscript.
Funding
This work was financially supported by CAMS Innovation Fund for Medical Sciences (2021-1-I2M-028).
Conflict of Interest
The authors declare that the research was conducted in the absence of any commercial or financial relationships that could be construed as a potential conflict of interest.
Publisher’s Note
All claims expressed in this article are solely those of the authors and do not necessarily represent those of their affiliated organizations, or those of the publisher, the editors, and the reviewers. Any product that may be evaluated in this article, or claim that may be made by its manufacturer, is not guaranteed or endorsed by the publisher.
Supplementary Material
The Supplementary Material for this article can be found online at: https://www.frontiersin.org/articles/10.3389/fchem.2021.753621/full#supplementary-material
References
Cadenas, E., and Davies, K. J. A. (2000). Mitochondrial Free Radical Generation, Oxidative Stress, and aging11 This Article Is Dedicated to the Memory of Our Dear Friend, Colleague, and mentor Lars Ernster (1920-1998), in Gratitude for All He Gave to Us. Free Radic. Biol. Med. 29, 222–230. doi:10.1016/s0891-5849(00)00317-8
Cao, D., Liu, Z., Verwilst, P., Koo, S., Jangjili, P., Kim, J. S., et al. (2019). Coumarin-Based Small-Molecule Fluorescent Chemosensors. Chem. Rev. 119, 10403–10519. doi:10.1021/acs.chemrev.9b00145
Castro-Godoy, W. D., Schmidt, L. C., and Argüello, J. E. (2019). A Green Alternative for the Conversion of Arylboronic Acids/Esters into Phenols Promoted by a Reducing Agent, Sodium Sulfite. Eur. J. Org. Chem. 2019, 3035–3039. doi:10.1002/ejoc.201900311
Chen, X., Wang, F., Hyun, J. Y., Wei, T., Qiang, J., Ren, X., et al. (2016). Recent Progress in the Development of Fluorescent, Luminescent and Colorimetric Probes for Detection of Reactive Oxygen and Nitrogen Species. Chem. Soc. Rev. 45, 2976–3016. doi:10.1039/c6cs00192k
Chen, Y., Gao, Y., He, Y., Zhang, G., Wen, H., Wang, Y., et al. (2021). Determining Essential Requirements for Fluorophore Selection in Various Fluorescence Applications Taking Advantage of Diverse Structure-Fluorescence Information of Chromone Derivatives. J. Med. Chem. 64, 1001–1017. doi:10.1021/acs.jmedchem.0c01508
Cheng, P., Miao, Q., Li, J., Huang, J., Xie, C., and Pu, K. (2019). Unimolecular Chemo-Fluoro-Luminescent Reporter for Crosstalk-free Duplex Imaging of Hepatotoxicity. J. Am. Chem. Soc. 141, 10581–10584. doi:10.1021/jacs.9b02580
D'Autréaux, B., and Toledano, M. B. (2007). ROS as Signalling Molecules: Mechanisms that Generate Specificity in ROS Homeostasis. Nat. Rev. Mol. Cell Biol 8, 813–824. doi:10.1038/nrm2256
Doura, T., Nonaka, H., and Sando, S. (2012). Atom Arrangement Strategy for Designing a Turn-on1H Magnetic Resonance Probe: a Dual Activatable Probe for Multimodal Detection of Hypochlorite. Chem. Commun. 48, 1565–1567. doi:10.1039/c1cc12044a
Dröge, W. (2002). Free Radicals in the Physiological Control of Cell Function. Physiol. Rev. 82, 47–95. doi:10.1152/physrev.00018.2001
Duanghathaipornsuk, S., Farrell, E. J., Alba-Rubio, A. C., Zelenay, P., and Kim, D. S. (2021). Detection Technologies for Reactive Oxygen Species: Fluorescence and Electrochemical Methods and Their Applications. Biosensors (Basel) 11. doi:10.3390/bios11020030
Fuloria, S., Subramaniyan, V., Karupiah, S., Kumari, U., Sathasivam, K., Meenakshi, D. U., et al. (2021). Comprehensive Review of Methodology to Detect Reactive Oxygen Species (ROS) in Mammalian Species and Establish its Relationship with Antioxidants and Cancer. Antioxidants (Basel) 10. doi:10.3390/antiox10010128
Jana, N., Nguyen, Q., and Driver, T. G. (2014). Development of a Suzuki Cross-Coupling Reaction between 2-Azidoarylboronic Pinacolate Esters and Vinyl Triflates to Enable the Synthesis of [2,3]-Fused Indole Heterocycles. J. Org. Chem. 79, 2781–2791. doi:10.1021/jo500252e
Jiao, X., Li, Y., Niu, J., Xie, X., Wang, X., and Tang, B. (2018). Small-Molecule Fluorescent Probes for Imaging and Detection of Reactive Oxygen, Nitrogen, and Sulfur Species in Biological Systems. Anal. Chem. 90, 533–555. doi:10.1021/acs.analchem.7b04234
Juan, C. A., Perez de la Lastra, J. M., Plou, F. J., and Perez-Lebena, E. (2021). The Chemistry of Reactive Oxygen Species (ROS) Revisited: Outlining Their Role in Biological Macromolecules (DNA, Lipids and Proteins) and Induced Pathologies. Int. J. Mol. Sci. 22. doi:10.3390/ijms22094642
Maeda, H., Yamamoto, K., Nomura, Y., Kohno, I., Hafsi, L., Ueda, N., et al. (2005). A Design of Fluorescent Probes for Superoxide Based on a Nonredox Mechanism. J. Am. Chem. Soc. 127, 68–69. doi:10.1021/ja047018k
Mamone, L., Di Venosa, G., Sáenz, D., Batlle, A., and Casas, A. (2016). Methods for the Detection of Reactive Oxygen Species Employed in the Identification of Plant Photosensitizers. Methods 109, 73–80. doi:10.1016/j.ymeth.2016.05.020
Miao, J., Cui, H., Jin, J., Lai, F., Wen, H., Zhang, X., et al. (2015). Development of 3-Alkyl-6-Methoxy-7-Hydroxy-Chromones (AMHCs) from Natural Isoflavones, a New Class of Fluorescent Scaffolds for Biological Imaging. Chem. Commun. 51, 881–884. doi:10.1039/c4cc06762b
Tampieri, F., Cabrellon, G., Rossa, A., Barbon, A., Marotta, E., and Paradisi, C. (2019). Comment on "Water-Soluble Fluorescent Probe with Dual Mitochondria/Lysosome Targetability for Selective Superoxide Detection in Live Cells and in Zebrafish Embryos". ACS Sens. 4, 3080–3083. doi:10.1021/acssensors.9b01358
Tang, B., Zhang, L., and Zhang, L.-l. (2004). Study and Application of Flow Injection Spectrofluorimetry with a Fluorescent Probe of 2-(2-Pyridil)-Benzothiazoline for Superoxide Anion Radicals. Anal. Biochem. 326, 176–182. doi:10.1016/j.ab.2003.11.023
Turrens, J. F. (2003). Mitochondrial Formation of Reactive Oxygen Species. J. Physiol. 552, 335–344. doi:10.1113/jphysiol.2003.049478
Wang, Y., Han, J., Xu, Y., Gao, Y., Wen, H., and Cui, H. (2020). Taking Advantage of the Aromatisation of 7-Diethylamino-4-Methyl-3,4-Dihydrocoumarin in the Fluorescence Sensing of Superoxide Anion. Chem. Commun. 56, 9827–9829. doi:10.1039/d0cc02282a
Wen, H., Xue, N., Wu, F., He, Y., Zhang, G., Hu, Z., et al. (2018). Exploration of the Fluorescent Properties and the Modulated Activities against Sirtuin Fluorogenic Assays of Chromenone-Derived Natural Products. Molecules 23. doi:10.3390/molecules23051063
Williams, A. T., Winfield, S. A., and Miller, J. N. (1983). Relative Fluorescence Quantum Yields Using a Computer-Controlled Luminescence Spectrometer. Analyst 108, 5. doi:10.1039/an9830801067
Woolley, J. F., Stanicka, J., and Cotter, T. G. (2013). Recent Advances in Reactive Oxygen Species Measurement in Biological Systems. Trends Biochem. Sci. 38, 556–565. doi:10.1016/j.tibs.2013.08.009
Wu, D., Chen, L., Xu, Q., Chen, X., and Yoon, J. (2019). Design Principles, Sensing Mechanisms, and Applications of Highly Specific Fluorescent Probes for HOCl/OCl-. Acc. Chem. Res. 52, 2158–2168. doi:10.1021/acs.accounts.9b00307
Xiao, H., Zhang, W., Li, P., Zhang, W., Wang, X., and Tang, B. (2020). Versatile Fluorescent Probes for Imaging the Superoxide Anion in Living Cells and In Vivo. Angew. Chem. Int. Ed. 59, 4216–4230. doi:10.1002/anie.201906793
Xing, P., Gao, K., Wang, B., Gao, J., Yan, H., Wen, J., et al. (2016). HEPES Is Not Suitable for Fluorescence Detection of HClO: a Novel Probe for HClO in Absolute PBS. Chem. Commun. 52, 5064–5066. doi:10.1039/c6cc00880a
Xu, K., Liu, X., Tang, B., Yang, G., Yang, Y., and An, L. (2007). Design of a Phosphinate-Based Fluorescent Probe for Superoxide Detection in Mouse Peritoneal Macrophages. Chem. Eur. J. 13, 1411–1416. doi:10.1002/chem.200600497
Yan, N., He, Y., Wen, H., Lai, F., Yin, D., and Cui, H. (2018). A Suzuki-Miyaura Method for Labelling Proliferating Cells Containing Incorporated BrdU. Analyst 143, 1224–1233. doi:10.1039/c7an01934c
Yang, B., Chen, Y., and Shi, J. (2019). Reactive Oxygen Species (ROS)-Based Nanomedicine. Chem. Rev. 119, 4881–4985. doi:10.1021/acs.chemrev.8b00626
Yang, M., Fan, J., Du, J., and Peng, X. (2020). Small-molecule Fluorescent Probes for Imaging Gaseous Signaling Molecules: Current Progress and Future Implications. Chem. Sci. 11, 5127–5141. doi:10.1039/d0sc01482f
Zhan, Z., Liu, R., Chai, L., Li, Q., Zhang, K., and Lv, Y. (2017). Turn-on Fluorescent Probe for Exogenous and Endogenous Imaging of Hypochlorous Acid in Living Cells and Quantitative Application in Flow Cytometry. Anal. Chem. 89, 9544–9551. doi:10.1021/acs.analchem.7b02613
Zhang, W., Li, P., Yang, F., Hu, X., Sun, C., Zhang, W., et al. (2013). Dynamic and Reversible Fluorescence Imaging of Superoxide Anion Fluctuations in Live Cells and In Vivo. J. Am. Chem. Soc. 135, 14956–14959. doi:10.1021/ja408524j
Keywords: coumarin, ROS, superoxide anion, redox-based probes, non–redox-based probes, selectivity
Citation: Wang Y, Jia S, Yu Z, Wen H and Cui H (2021) Insights Into the Detection Selectivity of Redox and Non-redox Based Probes for the Superoxide Anion Using Coumarin and Chromone as the Fluorophores. Front. Chem. 9:753621. doi: 10.3389/fchem.2021.753621
Received: 05 August 2021; Accepted: 18 October 2021;
Published: 25 November 2021.
Edited by:
Angela Stefanachi, Angela Stefanachi, ItalyReviewed by:
Naresh Kumar, SRM University (Delhi-NCR), IndiaAhmed A. Al-Amiery, National University of Malaysia, Malaysia
Copyright © 2021 Wang, Jia, Yu, Wen and Cui. This is an open-access article distributed under the terms of the Creative Commons Attribution License (CC BY). The use, distribution or reproduction in other forums is permitted, provided the original author(s) and the copyright owner(s) are credited and that the original publication in this journal is cited, in accordance with accepted academic practice. No use, distribution or reproduction is permitted which does not comply with these terms.
*Correspondence: Huaqing Cui, aGN1aUBpbW0uYWMuY24=
†These authors have contributed equally to this work