- 1Department of Biotechnology, Delft University of Technology, Delft, Netherlands
- 2School of Chemistry, University of Glasgow, Glasgow, United Kingdom
Fast and reliable industrial production of ammonia (NH3) is fundamentally sustaining modern society. Since the early 20th Century, NH3 has been synthesized via the Haber–Bosch process, running at conditions of around 350–500°C and 100–200 times atmospheric pressure (15–20 MPa). Industrial ammonia production is currently the most energy-demanding chemical process worldwide and contributes up to 3% to the global carbon dioxide emissions. Therefore, the development of more energy-efficient pathways for ammonia production is an attractive proposition. Over the past 20 years, scientists have imagined the possibility of developing a milder synthesis of ammonia by mimicking the nitrogenase enzyme, which fixes nitrogen from the air at ambient temperatures and pressures to feed leguminous plants. To do this, we propose the use of highly reconfigurable molecular metal oxides or polyoxometalates (POMs). Our proposal is an informed design of the polyoxometalate after exploring the catabolic pathways that cyanobacteria use to fix N2 in nature, which are a different route than the one followed by the Haber–Bosch process. Meanwhile, the industrial process is a “brute force” system towards breaking the triple bond N-N, needing high pressure and high temperature to increase the rate of reaction, nature first links the protons to the N2 to later easier breaking of the triple bond at environmental temperature and pressure. Computational chemistry data on the stability of different polyoxometalates will guide us to decide the best design for a catalyst. Testing different functionalized molecular metal oxides as ammonia catalysts laboratory conditions will allow for a sustainable reactor design of small-scale production.
Introduction
Multicellular organisms are unable to metabolize atmospheric N2 because of its high bond enthalpy and zero dipole moment. Instead, they source nitrogen from fixed resources such as nitrate and ammonia (Sadeghi et al., 2015). The process known as biological nitrogen fixation in which N2 is converted into assimilable forms is carried out by a specialized group of microorganisms that possess nitrogenases which are enzymes able to reduce atmospheric nitrogen into ammonia (NH3). At the start of the last century the only solid natural forms of nitrogen to enrich the soil were Peruvian guano and Chilean nitrate but in 1913, the Haber–Bosch process changed the course of the 20th Century allowing mass production of ammonia. In fact, ammonia production is the base of agriculture supporting between a third and a half of human food intake. Despite technical improvements for industrial NH3 production, it still requires both high temperature (350–500°C) and high pressure (15–20 MPa) consuming more than 1% of world-wide energy production and being one of the main world-wide producers of carbon dioxide and nitrous oxide emissions, both tagged as green-house gases (Foster et al., 2018). We can reduce travelling to mitigate climate change, but definitely, we cannot stop eating (Erisman et al., 2008), and massive industrial ammonia production of NH3 is fundamental in sustaining the human population (50% of the nitrogen found in human tissues originates from the Haber–Bosch process). However, the abuse of ammonia fertilizers, of which only about 50% are efficiently absorbed in soils, has led to an accumulation of nitrogen in natural waterbodies with negative consequences (such as limitation of natural diversity and proliferation of toxic algae) (Fields, 2004). Therefore, sustainable nitrogen fixation has remained as a critical area of research at the frontiers of inorganic, organometallic, coordination chemistry, and biochemistry for decades. Finding efficient alternatives to the Haber–Bosch process is a challenge because of the extraordinarily complicated characteristics of the reaction. In fact, ammonia synthesis is currently the most well-characterized heterogeneous catalytic reaction.
The overall reaction of ammonia synthesis from N2 is accessible thermodynamically at standard conditions (ΔG° = −16.4 kJ mol−1) (Lide, 2005), which indicates that this reaction could occur without external energy input at low temperatures. However, it does not take place spontaneously (Jia and Quadrelli, 2014). Kinetics, and endergonic production of intermediates, dictate operation at ca 350–500°C and elevated pressures are needed to achieve acceptable process yields at an industrial level (Hargreaves et al., 2020). The detailed thermodynamic analysis presented in (Jia and Quadrelli, 2014) also shows that although the overall reaction of fixing N2 is exergonic, the kinetic routes that lead to them demand high amounts of energy. Indeed, diazene and hydrazine are intermediates of the overall reaction with very high enthalpies of formation (Van Der Ham et al., 2014).
Given its global impact, the fundamentals of the Haber–Bosch process have hardly changed at all over the past 100 years. It still relies on an iron catalyst with potassium oxide and alumina acting as electronic and structural promoters, respectively (Galloway et al., 2013). In the early 1900s, Alwin Mittasch conducted a large-scale screening experiment to find a substitute for Haber’s osmium- and uranium-based catalysts (Hargreaves, 2014). Approximately 3,000 catalyst compositions were evaluated in over 20,000 small-scale tests. He developed a Fe-based catalyst, which is still used today, but in the 1970s ruthenium (Ru) was acknowledged as the best elemental metal catalyst for industrial ammonia production.
In recent years, there has been a large amount of research on reducing the temperature and pressure of the Haber-Bosch process using a variety of advanced catalysts such as promoted-iron, supported-ruthenium, and metal nitrides (Humphreys et al., 2021). Today we know that Ru has much higher activity than Fe, at least near thermodynamic equilibrium. However, due to the higher cost of Ru and its shorter catalytic lifetime, promoted Ru catalysts have only recently begun to challenge iron-based catalysts (Ross, 2019). Also, it has been long accepted that d-block metals can bind the abundant dinitrogen molecule, however, only a few are able to catalyze the conversion of dinitrogen to ammonia. Indeed, the main impediment to N2 fixation is primarily of kinetic nature (Jia and Quadrelli, 2014). After carefully analyzing existing thermodynamic experimental data, Borden provided an insightful explanation to the energetics of bonding H2 to N2 (Borden, 2017). The study showed how the difficulty associated to N2 fixation, is only partly due to the strength of one of the three N−N π bond that is broken in this reaction. In fact, the relative weakness of the intermediate sp2 N−H σ bonds in E-HN = NH obtained in this reaction plays a slightly larger role which allows us to conclude that reactivity of the intermediates rely on a delicate balance between the bonds that are formed and broken towards the yielding of the final product (Nicolaides and Borden, 1991).
Under the current global scenario of environmental emergency, it is urgent to find sustainable solutions to fulfil the ammonia demands of the human population. Novel design of catalysts is required to efficiently produce NH3 at low temperatures and with less energy requirements. Ideally, these catalyzers could drive N2 fixation at small scale, tailoring the operation for specific demands and contributing to the reduction of synthetic NH3 accumulation in the environment. But in developing these alternative solutions, it is necessary to design new catalysts which can follow alternative pathways that substitute the endergonic dissociative mechanism used in the Haber–Bosch process, and reduce the industrial energy spilt accounted for NH3 synthetic production.
Nitrogenases
Nature, contrary to chemists, has found a way to use the abundant N2 gas effectively at room temperature and neutral pH by using natural catalysts, enzymes, called nitrogenases. The nitrogenase can channel electrons and energy from different sources in anaerobic and aerobic conditions to form bioavailable NH3 breaking the triple bond of the (almost inert) molecules of N2 gas. Three homologous nitrogenases have been reported, distinguished by their metal-centred catalytic cofactors: molybdenum (MoFe), iron (FeFe) and vanadium (VFe) (Hu and Ribbe, 2015). Although the three homologous enzymes have been associated with specific activities, our understanding of the nitrogenase metal cofactors and their role is still incomplete (Rutledge and Tezcan, 2020).
The more ancient, abundant, efficient, and studied nitrogenase is the molybdenum containing system (Curatti et al., 2006). This nitrogenase is composed of two proteins, an homodimeric iron (FeP) protein (∼66 kDa) and the α2β2 heterotetrameric molybdenum-iron (MoFe) protein (∼240 kDa, with two complex metalloclusters). The FeP protein contains an ATP-binding site within each subunit interface of the protein, and it oversees the shuttle of eight electrons towards the reduction of 1 mole of N2. Concomitantly, 1 mole of H2 is produced per mole of N2 fixed. The explanation for this H2 reduction and apparent waste of equivalent power remains elusive but considering that H2 reduction by nitrogenase occurs only in the presence of N2, it has been proposed that production of H2 activates the FeMo-protein. Together, the oxidation of the low-potential [4Fe-4S]1+ cluster requires activation, and this happens when the hydrolysis of ATP takes place (Barsukova-Stuckart et al., 2012). Commonly, the ATP requirement of nitrogenase is evaluated as 2 moles of ATP are hydrolyzed into ADP and inorganic phosphate (Pi) per mole of electrons transferred, although more efficient ratios (down to 1 mole of ATP consumed per mole of electron) have been reported (Tan et al., 2016; Poudel et al., 2018). With this, the overall stoichiometry of natural N2 fixation remains as presented in Eq. 1.
The detailed explanation for the necessary loss of a cell’s energy currency (ATP) associated with nitrogenase activity remains elusive (Rabo and Schoonover, 2001; Milton et al., 2017) but it is assumed to be essential to reduce the activation barriers associated to the catalysis of the intermediates that lead to the overall reaction (Van Der Ham et al., 2014) and to activate the transfer of electrons (Rutledge and Tezcan, 2020). Also, the electron transfer to the substrate in nitrogenase seems to follow the description drawn in 1978 by Thorneley and colleagues (Thorneley et al., 1978), but the delicate and precise donation of electrons, protons and energy is not fully deciphered yet. Meanwhile, this optimized coordinated mechanism plays a fundamental role in maintaining the high efficiency of the non-selective nitrogenase enzyme (Kang et al., 2021).
After the donation of electrons, the [4Fe-4S]1+ cluster must be reduced again. This can happen by subsequent reduction by flavodoxin in aerobic or facultative anaerobic organisms, or by ferredoxin (more sensitive to O2 presence) in anaerobic ones. Phylogenetic analyses suggested the use of flavodoxin as strategy for diversification of nitrogenases in aerobic environments (Boyd et al., 2015). The electrons that feed flavodoxin and/or ferredoxin come directly from pyruvate or H2 oxidation (mostly in anaerobic organisms) or NAD(P)H electron carriers (aerobic, facultative anaerobes, and anoxygenic phototrophs) (Poudel et al., 2018). Indeed, the reduction of flavodoxin or ferredoxin starts the cycle towards N2 fixation again.
Although some research efforts have been trying to take advantage of the high efficiency of nitrogenase using the two-protein mechanism to directly catalyse N2 fixation (Harris et al., 2018), the high efficiency of electrons donated per mole of N2 fixated by nitrogen-fixing bacteria (8 electrons per mole of NH3 produced), has not been achieved by any in vitro system using the MoFe protein, the nitrogenase enzyme or any inorganic catalysts (Table 1). Engineering of nitrogenase in eukaryotic cells is another promising avenue but still requires overcoming fundamental challenges (Yang et al., 2014; Vicente and Dean, 2017). Therefore, other efforts have been directed towards the generation of enzymatic fuel cells, which has been approached using methyl viologen as solely electron mediator between a cathodic surface and a nitrogenase (Milton et al., 2017). This is a rather difficult catalysis as it requires an ATP regenerating system to activate the FeP protein, and anaerobic conditions, with remarkably low efficiencies reported. To remove the necessity of an ATP regeneration, bioelectrocatalysis of N2 fixation has been explored using only the MoFe protein of the nitrogenase and cobaltocene as electron mediator (Milton et al., 2016). However, production of NH3 was only reported with the reduction of N3− or NO2−.
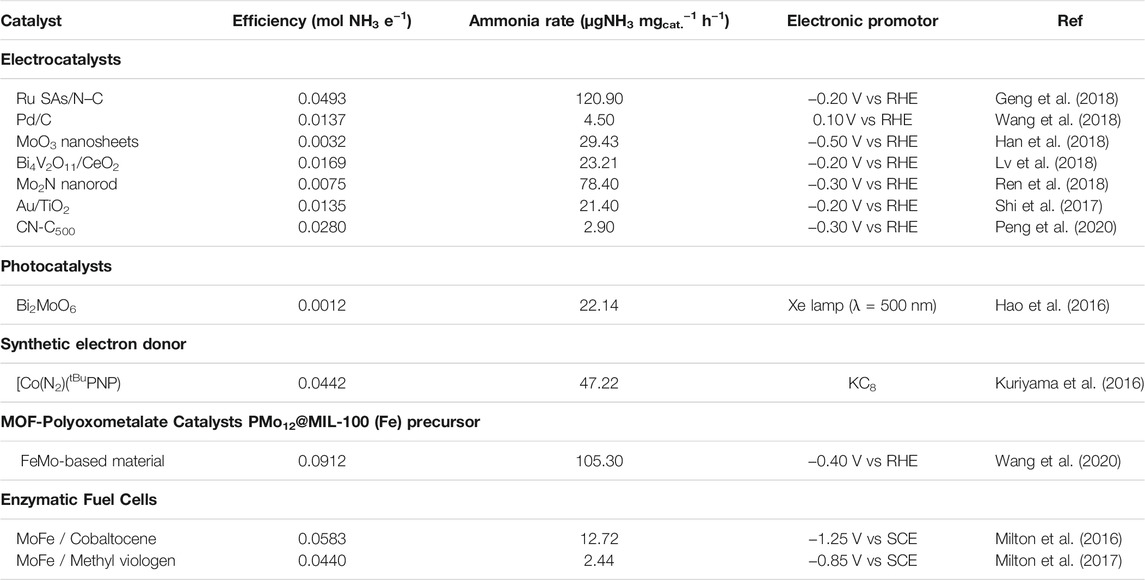
TABLE 1. Efficiencies of selected novel catalysts for N2 fixation under ambient temperatures and pressures. RHE = Reversible Hydrogen Electrode / SCE = Standard Calomel Electrode.
Few electrochemical systems that produce convincing amounts of NH3 have been reported with the most successful so far being the molybdenum based ones (see, Table 1). However, the poor Faradaic efficiency of these systems due to their low selectivity competing with H2 production, makes them, in many cases more energy demanding than Haber–Bosh process (Van Der Ham et al., 2014). These inefficiencies can only be surpassed by the design of other catalysts able to follow a more feasible reaction pathway at room temperature. The reliability of experimental electrochemical nitrogen reduction reaction (ENRR) experiments was questioned in a recent publication by Choi et al. detailing the complexity that arises from the potential intrusion of airborne contaminants. The reduction of nitrogen oxides (NO, NO2, etc.,) are more thermodynamically favorable than direct ENRR (Choi et al., 2020). Failure to control this has led to contentious Faradaic efficiencies and ammonia yields.
The design of novel bio-inspired catalysts, containing multiple active sites, has the potential to bypass the obvious limitations associated with exploitation of the complex nitrogenase enzyme, although competitive CO2 and H2 selectivity must be overcome with concomitant effectiveness in N2 adsorption and mechanistic delivery of electrons and protons (Bagger et al., 2021). Other authors, have reported that the use of a bio-inspired catalysts operating via an associative mechanism, like the one described for nitrogenases, are able to fix N2, CO2 and CH4 simultaneously at room temperature (Revilla-López et al., 2020). This can open the avenue for the development of new industrial processes able to combine N2 fixation with carbon homologation.
Molecular Metal Oxides or Polyoxometalates
Molecular metal oxides, or polyoxometalates (POMs) offer a route to design efficient ENRR using Earth abundant transition metals. POMs are primarily comprised of early-transition-metal (d-block) elements in their highest oxidation states. A great majority of these structures are anionic and consequently salts with charge balancing cations. In fact, POMs are an archetypal family of self-assembled molecular clusters that display a vast range of physical properties, structural features and sizes (Vilà-Nadal and Cronin, 2017). POMs are mainly formed by Mo6+ and W6+ combined with a main group oxyanion (phosphate, silicate, etc.,). Simply speaking, the synthesis of POM clusters in a “one-pot” solution involves dissolving the [MO4]n− (M = W, Mo) salt in aqueous solution followed by acidification, addition of electrophiles, buffer, additional cations and in some cases a reducing agent (Proust et al., 2012). The solution can then be processed by normal, microwave or hydrothermal heating followed by controlled precipitation to yield the cluster in crystalline form so that the structure of the cluster can be elucidated by single crystal X-ray diffraction (Long et al., 2004).This route has been used in 99% of all cases in POM chemistry and is very convenient to yield complex structures from “one-pot” but suffers a great deal from dependence on initial reaction conditions, reproducibility, and the ability to systematically investigate parameter space to design new cluster architectures. In this respect, during the last decade the field of POMs has been transformed by trapping reactive building blocks and generating an accessible building block library as a function of pH, template, linker heteroatoms, and cation type (Miras et al., 2020). The key aspect here is that the heteroatom mediated assembly of the anionic metal-oxo units to building blocks which then link to clusters, can be used to form new types of materials with novel and unprecedented architectures (Zheng et al., 2018). In fact, POM structures and functionalities make them ideal candidates as model systems for metal-oxide-anchored single atom catalysts (POM-SAC) (Liu and Streb, 2021). POMs are polynuclear metal oxide anions that are molecular analogues of solid-state metal oxides. Diverse fields such as, water oxidation catalysts (Blasco-Ahicart et al., 2018), photocatalysis (Costa-Coquelard et al., 2010), molecular electronics (Busche et al., 2014), quantum computation (Gaita-Ariño et al., 2019), biology (Gumerova and Rompel, 2021) and medicinal science (Lu et al., 2021) have all been impacted by POM chemistry. Current findings demonstrate the feasibility of hydrogen-production using silicotungstic acid, H6[SiW12O4], by coupling low-pressure oxygen production via water oxidation linked to non-electrolyzer catalytic hydrogen production (Rausch et al., 2014). Given their structural diversity and versatility of POM cluster applications, they are ideal candidates to provide further insight into the heterogeneous Haber–Bosch catalyst or the low-energy nitrogenase enzymes that directly make ammonia.
Discussion
Ammonia is a viable hydrogen energy vector, and its pre-existing industry, which produces, stores, and trades millions of tons of ammonia annually, means that the infrastructure necessary to jump-start the hydrogen economy already exists. The United Kingdom has developed detailed plans for the next decade to use “green” ammonia as an energy storage material for renewable electricity (The Royal Society, 2020).
The global cycling of nitrogen through the biosphere depends upon a heavy element: molybdenum and requires bacteria in the fixation of nitrogen (Hille, 2002). However, when extensively starved nitrogen-fixating bacteria A. Viinelandi were grown in a medium that lacked molybdate but that contained tungstate, A. vinelandii synthesized the regular storage protein but with tungstate. This is perhaps not surprising since tungsten, lies below molybdenum in the d-block, and is consequently expected to feature chemical properties related to those of molybdenum. Recent work indicated that molybdenum and tungsten-based enzymes are incredibly ancient and their enzymatic role and functionality has been preserved (Vitousek et al., 2002). It is thought that in the reducing environment of the primordial world tungsten-enzymes were favoured. In those days, oxygen atom transfer reactions were more challenging than in our oxic modern world, with its preference for molybdenum-enzymes (Schemberg et al., 2007). By deepening our understanding of the microbial populations that cycle nitrogen, we can find opportunities to deliver more efficient bioengineering solutions. To date, no one has systematically explored the new biotechnologies for nitrogen removal that can emerge from this new knowledge because a purely empirical exploration would require significant investigation. To achieve low-temperature, cost-effective and efficient electrochemical ammonia synthesis requires a multidisciplinary approach able to characterise natural biocatalysts (nitrogenases) that efficiently catalyse N2 reduction, as well as develop heterogeneous (molecular) catalytic systems informed by current computational theory developments in the area that can direct efficiently experimental investigation (Foster et al., 2018).
We will start by looking into transition metal substituted lacunary Keggin anions, as shown in Figure 1. Such structures are derivatives from the parent anion [XM12O40]n−, where X is the heteroatom (most commonly are P5+, Si4+, or B3+), and M = W, Mo, inspired by recent work in the area, (Lin et al., 2020) which investigated the Gibbs free energy change for the reductive adsorption of *N2 and *H on four Keggin-POM-supported Ru single atom electrocatalysts. The phosphorus-templated tungstate- and molybdate-Keggin clusters presented high nitrogen-binding selectivity, whereas the silicon-templated analogues prefer hydrogen binding. Our aim is to explore the functionalization of molecular dinitrogen and its catalytic conversion in POMs by combining our expertise in inorganic chemistry with exploring the catalytic conversion d-block metals. This will be our theoretical model structure, bearing in mind that the pH increases the Mo- and W-based Keggin ions gradually disintegrate (Kondinski and Parac-Vogt, 2018). Computational chemistry will help us to describe the intermediates of bioinspired reaction pathways. These results will complement in-depth metabolic analyses of highly efficient nitrogenases at ambient temperature and pressure. We will work closely with experimentalists in the area that will help us to translate our theoretical results into effective experimental N2 reduction catalysis.
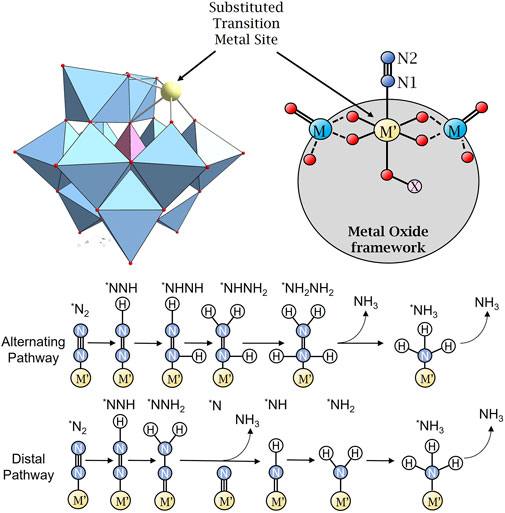
FIGURE 1. Polyhedral (top-left) representation of mono-substituted heteropolyanion. The nitrogen molecule adsorbs onto the transition metal site through the η1 (end-on) binding mode. The schematic depiction (top-right) shows the mono-substituted heteropolyanion in the nitrogen-bound state. Below this, a schematic depiction of the associative mechanisms for nitrogen reduction in which the N-N bond is cleaved simultaneously with the release of ammonia. The associative mechanism can proceed via two separate pathways—“alternating” and “distal” which invoke distinctly different intermediates. Colours corresponding to addenda metal = Cyan; substituted metal = yellow; heteroatom = Pink; O = red; N = dark blue; and H = white.
Data Availability Statement
The original contributions presented in the study are included in the article/supplementary material, further inquiries can be directed to the corresponding authors.
Author Contributions
LV-N conceived the idea, designed the project and together with RG-C coordinated the efforts of the research team. LV-N and RG-C co-wrote the paper with input from JAT.
Funding
Financial support for this work was provided by University of Glasgow and the Engineering and Physical Sciences Research Council Grants (EP/S030603/1; EP/R513222/1; EP/T517896/1), Royal Society of Chemistry RSC Hardship Grant (Covid-19). We also thank the University of Glasgow Early Career Development Programme (ECDP) for support.
Conflict of Interest
The authors declare that the research was conducted in the absence of any commercial or financial relationships that could be construed as a potential conflict of interest.
Publisher’s Note
All claims expressed in this article are solely those of the authors and do not necessarily represent those of their affiliated organizations, or those of the publisher, the editors and the reviewers. Any product that may be evaluated in this article, or claim that may be made by its manufacturer, is not guaranteed or endorsed by the publisher.
Acknowledgments
The authors acknowledge Justin Hargreaves from the School of Chemistry at the University of Glasgow for useful ongoing discussions in this project and proof reading the manuscript. We acknowledge Cindy Smith from the School of Engineering at the University of Glasgow for ongoing discussions on environmental controls of the microorganisms driving the nitrogen cycle.
References
Bagger, A., Wan, H., Stephens, I. E. L., and Rossmeisl, J. (2021). Role of Catalyst in Controlling N2 Reduction Selectivity: A Unified View of Nitrogenase and Solid Electrodes. ACS Catal. 11, 6596–6601. doi:10.1021/acscatal.1c01128
Barsukova-Stuckart, M., Izarova, N. V., Barrett, R. A., Wang, Z., van Tol, J., Kroto, H. W., et al. (2012). Polyoxopalladates Encapsulating 8-Coordinated Metal Ions, [MO8PdII12L8]n− (M = Sc3+, Mn2+, Fe3+, Co2+, Ni2+, Cu2+, Zn2+, Lu3+; L = PhAsO32-, PhPO32-, SeO32-). Inorg. Chem. 51, 13214–13228. doi:10.1021/ic301537n
Blasco-Ahicart, M., Soriano-López, J., Carbó, J. J., Poblet, J. M., and Galan-Mascaros, J. R. (2018). Polyoxometalate Electrocatalysts Based on Earth-Abundant Metals for Efficient Water Oxidation in Acidic media. Nat. Chem 10, 24–30. doi:10.1038/NCHEM.2874
Borden, W. T. (2017). Why Are Addition Reactions to N2 Thermodynamically Unfavorable? J. Phys. Chem. A. 121, 1140–1144. doi:10.1021/acs.jpca.6b11728
Boyd, E. S., Costas, A. M. G., Hamilton, T. L., Mus, F., and Peters, J. W. (2015). Evolution of Molybdenum Nitrogenase during the Transition from Anaerobic to Aerobic Metabolism. J. Bacteriol. 197, 1690–1699. doi:10.1128/JB.02611-14
Busche, C., Vilà-Nadal, L., Yan, J., Miras, H. N., Long, D.-L., Georgiev, V. P., et al. (2014). Design and Fabrication of Memory Devices Based on Nanoscale Polyoxometalate Clusters. Nature 515, 545–549. doi:10.1038/nature13951
Choi, J., Suryanto, B. H. R., Wang, D., Du, H.-L., Hodgetts, R. Y., Ferrero Vallana, F. M., et al. (2020). Identification and Elimination of False Positives in Electrochemical Nitrogen Reduction Studies. Nat. Commun. 11, 1–10. doi:10.1038/s41467-020-19130-z
Costa-Coquelard, C., Sorgues, S., and Ruhlmann, L. (2010). Photocatalysis with Polyoxometalates Associated to Porphyrins under Visible Light: An Application of Charge Transfer in Electrostatic Complexes. J. Phys. Chem. A. 114, 6394–6400. doi:10.1021/jp101261n
Curatti, L., Ludden, P. W., and Rubio, L. M. (2006). NifB-dependent In Vitro Synthesis of the Iron-Molybdenum Cofactor of Nitrogenase. Proc. Natl. Acad. Sci. 103, 5297–5301. doi:10.1073/pnas.0601115103
Erisman, J. W., Sutton, M. A., Galloway, J., Klimont, Z., and Winiwarter, W. (2008). How a century of Ammonia Synthesis Changed the World. Nat. Geosci 1, 636–639. doi:10.1038/ngeo325
Fields, S. (2004). Global Nitrogen: Cycling Out of Control. Environ. Health Perspect. 112, 556–563. doi:10.1289/ehp.112-a556
Foster, S. L., Bakovic, S. I. P., Duda, R. D., Maheshwari, S., Milton, R. D., Minteer, S. D., et al. (2018). Catalysts for Nitrogen Reduction to Ammonia. Nat. Catal. 1, 490–500. doi:10.1038/s41929-018-0092-7
Gaita-Ariño, A., Luis, F., Hill, S., and Coronado, E. (2019). Molecular Spins for Quantum Computation. Nat. Chem. 11, 301–309. doi:10.1038/s41557-019-0232-y
Galloway, J. N., Leach, A. M., Bleeker, A., and Erisman, J. W. (2013). A Chronology of Human Understanding of the Nitrogen Cycle. Phil. Trans. R. Soc. B 368, 20130120. doi:10.1098/rstb.2013.0120
Geng, Z., Liu, Y., Kong, X., Li, P., Li, K., Liu, Z., et al. (2018). Achieving a Record-High Yield Rate of 120.9 μgNH3 mgcat.−1 H−1 for N2 Electrochemical Reduction over Ru Single-Atom Catalysts. Adv. Mater. 30, 1803498–1803507. doi:10.1002/adma.201803498
Gumerova, N. I., and Rompel, A. (2021). Interweaving Disciplines to Advance Chemistry: Applying Polyoxometalates in Biology. Inorg. Chem. 60, 6109–6114. doi:10.1021/acs.inorgchem.1c00125
Han, J., Ji, X., Ren, X., Cui, G., Li, L., Xie, F., et al. (2018). MoO3 Nanosheets for Efficient Electrocatalytic N2 Fixation to NH3. J. Mater. Chem. A. 6, 12974–12977. doi:10.1039/c8ta03974g
Hao, Y., Dong, X., Zhai, S., Ma, H., Wang, X., and Zhang, X. (2016). Hydrogenated Bismuth Molybdate Nanoframe for Efficient Sunlight-Driven Nitrogen Fixation from Air. Chem. Eur. J. 22, 18722–18728. doi:10.1002/chem.201604510
Hargreaves, J. S. J., Chung, Y.-M., Ahn, W.-S., Hisatomi, T., Domen, K., Kung, M. C., et al. (2020). Minimizing Energy Demand and Environmental Impact for Sustainable NH3 and H2O2 Production-A Perspective on Contributions from thermal, Electro-, and Photo-Catalysis. Appl. Catal. A: Gen. 594, 117419. doi:10.1016/j.apcata.2020.117419
Hargreaves, J. S. J. (2014). Nitrides as Ammonia Synthesis Catalysts and as Potential Nitrogen Transfer Reagents. Appl. Petrochem Res. 4, 3–10. doi:10.1007/s13203-014-0049-y
Harris, D. F., Lukoyanov, D. A., Shaw, S., Compton, P., Tokmina-Lukaszewska, M., Bothner, B., et al. (2018). Mechanism of N2Reduction Catalyzed by Fe-Nitrogenase Involves Reductive Elimination of H2. Biochemistry 57, 701–710. doi:10.1021/acs.biochem.7b01142
Hille, R. (2002). Molybdenum and Tungsten in Biology. Trends Biochem. Sci. 27, 360–367. doi:10.1016/S0968-0004(02)02107-2
Hu, Y., and Ribbe, M. W. (2015). Nitrogenase and Homologs. J. Biol. Inorg. Chem. 20, 435–445. doi:10.1007/s00775-014-1225-3
Humphreys, J., Lan, R., and Tao, S. (2021). Development and Recent Progress on Ammonia Synthesis Catalysts for Haber-Bosch Process. Adv. Energ. Sustain. Res. 2, 2000043. doi:10.1002/aesr.202000043
Jia, H.-P., and Quadrelli, E. A. (2014). Mechanistic Aspects of Dinitrogen Cleavage and Hydrogenation to Produce Ammonia in Catalysis and Organometallic Chemistry: Relevance of Metal Hydride Bonds and Dihydrogen. Chem. Soc. Rev. 43, 547–564. doi:10.1039/c3cs60206k
Kang, W., Lee, C. C., Jasniewski, A. J., Ribbe, M. W., and Hu, Y. (2021). Response to Comment on "Structural Evidence for a Dynamic Metallocofactor during N2 Reduction by Mo-Nitrogenase". Science 371, eabe5856–1385. doi:10.1126/science.abe5856
Kondinski, A., and Parac-Vogt, T. N. (2018). Keggin Structure, Quō Vādis? Front. Chem. 6, 1–7. doi:10.3389/fchem.2018.00346
Kuriyama, S., Arashiba, K., Tanaka, H., Matsuo, Y., Nakajima, K., Yoshizawa, K., et al. (2016). Direct Transformation of Molecular Dinitrogen into Ammonia Catalyzed by Cobalt Dinitrogen Complexes Bearing Anionic PNP Pincer Ligands. Angew. Chem. 128, 14503–14507. doi:10.1002/ange.201606090
Lide, D. R. (2005). CRC Handbook of Chemistry and Physics: A Ready-Reference of Chemical and Physical Data, 85th ed Edited by David R. Lide. Boca Raton, FL: CRC Press LLC, 2313–2314.
Lin, L., Gao, L., Xie, K., Jiang, R., and Lin, S. (2020). Ru-polyoxometalate as a Single-Atom Electrocatalyst for N2 Reduction to NH3 with High Selectivity at Applied Voltage: A Perspective from DFT Studies. Phys. Chem. Chem. Phys. 22, 7234–7240. doi:10.1039/d0cp00698j
Liu, R., and Streb, C. (2021). Polyoxometalate‐Single Atom Catalysts (POM‐SACs) in Energy Research and Catalysis. Adv. Energ. Mater. 11, 2101120. doi:10.1002/aenm.202101120
Long, D.-L., Kögerler, P., and Cronin, L. (2004). Old Clusters with New Tricks: Engineering S⋅⋅⋅S Interactions and Novel Physical Properties in Sulfite-Based Dawson Clusters. Angew. Chem. Int. Ed. 43, 1817–1820. doi:10.1002/anie.200352896
Lu, F., Wang, M., Li, N., and Tang, B. (2021). Polyoxometalate‐Based Nanomaterials toward Efficient Cancer Diagnosis and Therapy. Chem. Eur. J. 27, 6422–6434. doi:10.1002/chem.202004500
Lv, C., Yan, C., Chen, G., Ding, Y., Sun, J., Zhou, Y., et al. (2018). An Amorphous Noble-Metal-Free Electrocatalyst that Enables Nitrogen Fixation under Ambient Conditions. Angew. Chem. Int. Ed. Engl. 57, 6073–6076. doi:10.1002/adma.20180349810.1002/anie.201801538
Milton, R. D., Abdellaoui, S., Khadka, N., Dean, D. R., Leech, D., Seefeldt, L. C., et al. (2016). Nitrogenase Bioelectrocatalysis: Heterogeneous Ammonia and Hydrogen Production by MoFe Protein. Energy Environ. Sci. 9, 2550–2554. doi:10.1039/c6ee01432a
Milton, R. D., Cai, R., Abdellaoui, S., Leech, D., De Lacey, A. L., Pita, M., et al. (2017). Bioelectrochemical Haber-Bosch Process: An Ammonia-Producing H2 /N2 Fuel Cell. Angew. Chem. Int. Ed. 56, 2680–2683. doi:10.1002/anie.201612500
Miras, H. N., Mathis, C., Xuan, W., Long, D.-L., Pow, R., and Cronin, L. (2020). Spontaneous Formation of Autocatalytic Sets with Self-Replicating Inorganic Metal Oxide Clusters. Proc. Natl. Acad. Sci. USA 117, 10699–10705. doi:10.1073/pnas.1921536117
Nicolaides, A., and Borden, W. T. (1991). Ab Initio calculations of the Relative Strengths of the .Pi. Bonds in Acetylene and Ethylene and of Their Effect on the Relative Energies of .pi.-bond Addition Reactions. J. Am. Chem. Soc. 113, 6750–6755. doi:10.1021/ja00018a005
Peng, G., Wu, J., Wang, M., Niklas, J., Zhou, H., and Liu, C. (2020). Nitrogen-Defective Polymeric Carbon Nitride Nanolayer Enabled Efficient Electrocatalytic Nitrogen Reduction with High Faradaic Efficiency. Nano Lett. 20, 2879–2885. doi:10.1021/acs.nanolett.0c00698
Poudel, S., Colman, D. R., Fixen, K. R., Ledbetter, R. N., Zheng, Y., Pence, N., et al. (2018). Electron Transfer to Nitrogenase in Different Genomic and Metabolic Backgrounds. J. Bacteriol. 200, 1–19. doi:10.1128/JB.00757-17
Proust, A., Matt, B., Villanneau, R., Guillemot, G., Gouzerh, P., and Izzet, G. (2012). Functionalization and post-functionalization: a Step towards Polyoxometalate-Based Materials. Chem. Soc. Rev. 41, 7605–7622. doi:10.1039/c2cs35119f
Rabo, J. a., and Schoonover, M. W. (2001). Early Discoveries in Zeolite Chemistry and Catalysis at Union Carbide, and Follow-Up in Industrial Catalysis. Appl. Catal. A: Gen. 222, 261–275. doi:10.1016/S0926-860X(01)00840-7
Rausch, B., Symes, M. D., Chisholm, G., and Cronin, L. (2014). Decoupled Catalytic Hydrogen Evolution from a Molecular Metal Oxide Redox Mediator in Water Splitting. Science 345, 1326–1330. doi:10.1126/science.1257443345
Ren, X., Cui, G., Chen, L., Xie, F., Wei, Q., Tian, Z., et al. (2018). Electrochemical N2fixation to NH3under Ambient Conditions: Mo2N Nanorod as a Highly Efficient and Selective Catalyst. Chem. Commun. 54, 8474–8477. doi:10.1039/c8cc03627f
Revilla-López, G., Sans, J., Casanovas, J., Bertran, O., Puiggalí, J., Turon, P., et al. (2020). Analysis of Nitrogen Fixation by a Catalyst Capable of Transforming N2, CO2 and CH4 into Amino Acids under Mild Reactions Conditions. Appl. Catal. A: Gen. 596, 117526. doi:10.1016/j.apcata.2020.117526
Ross, J. R. H. (2019, An Introduction to Heterogeneous Catalysis and its Development through the Centuries-Chemistry in Two Dimensions, 3, 38). doi:10.1016/b978-0-444-63474-0.00001-1
Rutledge, H. L., and Tezcan, F. A. (2020). Electron Transfer in Nitrogenase. Chem. Rev. 120, 5158–5193. doi:10.1021/acs.chemrev.9b00663
Sadeghi, O., Zakharov, L. N., and Nyman, M. (2015). Aqueous Formation and Manipulation of the Iron-Oxo Keggin Ion. Science 347, 1359–1362. doi:10.1126/science.aaa4620
Schemberg, J., Schneider, K., Demmer, U., Warkentin, E., Müller, A., and Ermler, U. (2007). Towards Biological Supramolecular Chemistry: A Variety of Pocket-Templated, Individual Metal Oxide Cluster Nucleations in the Cavity of a Mo/W-Storage Protein. Angew. Chem. Int. Ed. 46, 2408–2413. doi:10.1002/anie.200604858
Shi, M.-M., Bao, D., Wulan, B.-R., Li, Y.-H., Zhang, Y.-F., Yan, J.-M., et al. (2017). Au Sub-nanoclusters on TiO2toward Highly Efficient and Selective Electrocatalyst for N2Conversion to NH3at Ambient Conditions. Adv. Mater. 29, 1606550–1606557. doi:10.1002/adma.201606550
Tan, M. L., Perrin, B. S., Niu, S., Huang, Q., and Ichiye, T. (2016). Protein dynamics and the all‐ferrous [ F e 4 S 4 ] cluster in the nitrogenase iron protein. Protein Sci. 25, 12–18. doi:10.1002/pro.2772
The Royal Society (2020). Ammonia: Zero-Carbon Fertiliser, Fuel and Energy Store. London: Policy Briefing.
Thorneley, R. N. F., Eady, R. R., and Lowe, D. J. (1978). Biological Nitrogen Fixation by Way of an Enzyme-Bound Dinitrogen-Hydride Intermediate. Nature 272, 557–558. doi:10.1038/272557a0
Van Der Ham, C. J. M., Koper, M. T. M., and Hetterscheid, D. G. H. (2014). Challenges in Reduction of Dinitrogen by Proton and Electron Transfer. Chem. Soc. Rev. 43, 5183–5191. doi:10.1039/c4cs00085d
Vicente, E. J., and Dean, D. R. (2017). Keeping the Nitrogen-Fixation Dream Alive. Proc. Natl. Acad. Sci. USA 114, 3009–3011. doi:10.1073/pnas.1701560114
Vilà-Nadal, L., and Cronin, L. (2017). Design and Synthesis of Polyoxometalate-Framework Materials from Cluster Precursors. Nat. Rev. Mater. 2. doi:10.1038/natrevmats.2017.54
Vitousek, P. M., Cassman, K., Cleveland, C., Crews, T., Christopher, B., Grimm, N. B., et al. (2002). Towards an Ecological Understanding of Biological Nitrogen Fixation Rastetter and Janet I . Sprent Published By : Springer Stable URL . Biogeochemistry 57, 1–45. doi:10.1007/978-94-017-3405-9_1
Wang, J., Yu, L., Hu, L., Chen, G., Xin, H., and Feng, X. (2018). Ambient Ammonia Synthesis via Palladium-Catalyzed Electrohydrogenation of Dinitrogen at Low Overpotential. Nat. Commun. 9. doi:10.1038/s41467-018-04213-9
Wang, X., Feng, Z., Xiao, B., Zhao, J., Ma, H., Tian, Y., et al. (2020). Polyoxometalate-based Metal-Organic Framework-Derived Bimetallic Hybrid Materials for Upgraded Electrochemical Reduction of Nitrogen. Green. Chem. 22, 6157–6169. doi:10.1039/d0gc01149e
Yang, J., Xie, X., Wang, X., Dixon, R., and Wang, Y.-P. (2014). Reconstruction and Minimal Gene Requirements for the Alternative Iron-Only Nitrogenase in Escherichia coli. Proc. Natl. Acad. Sci. 111, E3718–E3725. doi:10.1073/pnas.1411185111
Keywords: nitrogen fixation, polyoxomatalate, nitrogenase, compuatational chemistry, metabolic modelling, Haber Bosch, catalyst—N
Citation: González-Cabaleiro R, Thompson JA and Vilà-Nadal L (2021) Looking for Options to Sustainably Fixate Nitrogen. Are Molecular Metal Oxides Catalysts a Viable Avenue?. Front. Chem. 9:742565. doi: 10.3389/fchem.2021.742565
Received: 16 July 2021; Accepted: 17 August 2021;
Published: 14 September 2021.
Edited by:
Jennifer Hiscock, University of Kent, United KingdomReviewed by:
Subhamay Pramanik, University of Kansas, United StatesMatthew A. Addicoat, Nottingham Trent University, United Kingdom
Copyright © 2021 González-Cabaleiro, Thompson and Vilà-Nadal. This is an open-access article distributed under the terms of the Creative Commons Attribution License (CC BY). The use, distribution or reproduction in other forums is permitted, provided the original author(s) and the copyright owner(s) are credited and that the original publication in this journal is cited, in accordance with accepted academic practice. No use, distribution or reproduction is permitted which does not comply with these terms.
*Correspondence: Rebeca González-Cabaleiro, ci5nb256YWxlemNhYmFsZWlyb0B0dWRlbGZ0Lm5s; Laia Vilà-Nadal, bGFpYS52aWxhLW5hZGFsQGNoZW0uZ2xhLmFjLnVr