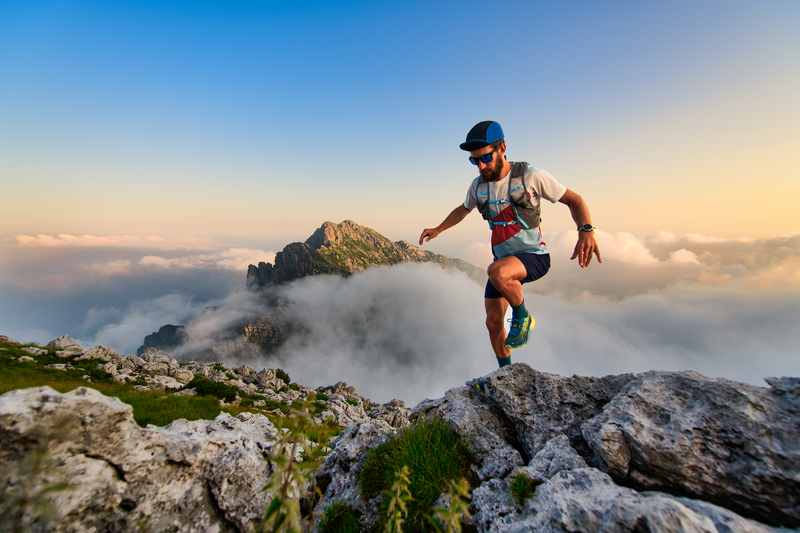
95% of researchers rate our articles as excellent or good
Learn more about the work of our research integrity team to safeguard the quality of each article we publish.
Find out more
ORIGINAL RESEARCH article
Front. Chem. , 19 August 2021
Sec. Theoretical and Computational Chemistry
Volume 9 - 2021 | https://doi.org/10.3389/fchem.2021.734460
This article is part of the Research Topic Design of Two-Dimensional Functional Materials and Nanodevices View all 18 articles
The CO2 electrochemical reduction reaction (CO2RR) has been a promising conversion method for CO2 utilization. Currently, the lack of electrocatalysts with favorable stability and high efficiency hindered the development of CO2RR. Nitrogen-doped graphene nanocarbons have great promise in replacing metal catalysts for catalyzing CO2RR. By using the density functional theory (DFT) method, the catalytic mechanism and activity of CO2RR on 11 types of nitrogen-doped graphene have been explored. The free energy analysis reveals that the zigzag pyridinic N- and zigzag graphitic N-doped graphene possess outstanding catalytic activity and selectivity for HCOOH production with an energy barrier of 0.38 and 0.39 eV, respectively. CO is a competitive product since its free energy lies only about 0.20 eV above HCOOH. The minor product is CH3OH and CH4 for the zigzag pyridinic N-doped graphene and HCHO for zigzag graphitic N-doped graphene, respectively. However, for Z-pyN, CO2RR is passivated by too strong HER. Meanwhile, by modifying the pH value of the electrolyte, Z-GN could be selected as a promising nonmetal electrocatalyst for CO2RR in generating HCOOH.
As one of the greenhouse gases, the continual accumulation of CO2 causes global warming, which significantly hinders the sustainable development of human society (Thomas et al., 2004; Lewis et al., 2006; Cook et al., 2010). The unbalanced CO2 emission and consumption is becoming a pressing issue (Kondratenko et al., 2013; Appel et al., 2013). In this aspect, CO2 electrochemical reduction reaction (CO2RR) by using the renewable energy sources (Yi et al., 2019; Wang et al., 2020; Lu et al., 2021) offers a promising way to produce fuels and value-added chemicals. Up to now, the major obstacle for CO2RR is the lack of electrocatalysts with high stability and efficiency. Particularly, the cathode electrocatalyst materials play a key role in the complicated product distribution of CO2RR (Lim et al., 2014; Zhu et al., 2016). Therefore, searching for suitable electrocatalysts for CO2RR is one of the hot topics in recent years. Till now, a lot of electrocatalysts for CO2RR have been studied, including noble metals (Zhu et al., 2013; Kang et al., 2014; Gao et al., 2015; Kim et al., 2015), base metals (Hori et al., 1985; Hori et al., 1986; Nie et al., 2013; Zhang et al., 2014a), alloys (Kim et al., 2014; Bai et al., 2017), and metal oxides (Lee et al., 2015; Ren et al., 2015). It is well known that Ag and Au are prone to produce CO via the two-electron reaction pathway (Zhu et al., 2013; Kim et al., 2015). In addition, Cu is recognized as a state-of-the-art CO2RR catalyst for generating multi-electron products, such as CO, HCOOH, CH3OH, and CH4 (Hori et al., 1985; Hori et al., 1986; Nie et al., 2013). However, the high cost, low efficiency due to the competitive hydrogen evolution reaction (HER), and high overpotential restrict their practical implementation and industrial-scale development in CO2RR (Lim et al., 2014).
To solve the above issues, metal-free electrocatalysts based on carbon materials have been studied, owing to their low cost, high stability, outstanding mechanical flexibility, and superior structural durability. The introduction of heteroatoms (such as N, B, and S) could not only modify the electronic structure of carbon materials but also contribute to take advantage of the existing defects appropriately (Wang X. et al., 2014). For N-doped carbon nanofibers (NCNFs), it shows negligible overpotential (0.17 V) and 13 times higher current density than bulk Ag catalyst for CO2RR (Kumar et al., 2013). In addition, N-doped carbon nanotubes (NCNTs) (Sharma et al., 2015), N-doped nanoporous carbon–carbon nanotube composite membrane (HNCM/CNT) (Wang et al., 2017), and polyethylenimine functionalized NCNTs have been proven to be highly active and stable electrocatalysts for CO2RR (Zhang et al., 2014b). Remarkably, N-doped graphene possesses excellent durability in the CO2RR process, achieving a maximum faradaic efficiency (FE) of 73% for formate with overpotential of 0.84 V (Wang et al., 2016). N-doped graphene quantum dots (NGQDs) could catalyze carbon dioxide into multicarbon hydrocarbons and oxygenates at high FE (up to 90%), with excellent selectivity (45% for ethylene and ethanol conversions) (Wu et al., 2016).
With respect to the active sites of nitrogen-doped carbon materials for CO2RR, it is a controversial issue among the pyridinic N, pyrrolic N, graphitic N, and the C adjacent to N. Generally, these potential active sites coexist in the carbon materials, which adds to the difficulty in identifying the active site. A theoretical study indicates that for CO2 electroreduction to CO on NCNTs, the optimal active site is pyridinic N, followed by pyrrolic N and graphitic N (Wu et al., 2015). Another study about CO2RR on NCNTs emphasizes that the presence of graphitic and pyridinic N defects remarkably increases the selectivity toward CO formation and decreases the absolute overpotential (Sharma et al., 2015). For N-doped graphene-like material/carbon paper electrodes (NGM/CP), the FE is as high as 93.5% in producing CH4, which is ascribed to the reactive pyridinic and pyrrolic N sites (Sun et al., 2016). A theoretical study suggested that COOH production on pyrrolic N3 is downhill by −0.21 eV, while it is uphill for pyridinic and graphitic N (Liu et al., 2016). Overall, both the experimental and theoretical studies indicate that N-doped carbon materials show significant catalytic performance of CO2RR.
Inspired by these studies, we studied CO2RR on N-doped graphene from the perspective of theoretical calculation in this work. To make a systematic comparison, N was doped into graphene at in-plane, zigzag edge, armchair edge, and pyrrolic edge sites, respectively. It would contribute to identifying the most dominant structure and providing a valuable design strategy for further activity enhancement in the experiment. In this study, the first-principle calculation has been performed to uncover the CO2RR reaction pathways and electrocatalytic activity on different edges of N-doped (zigzag edge, armchair edge, and pyrrolic edge) graphene structures within a unified thermodynamic reaction scheme.
The geometry optimization and energy calculations were performed within the density functional theory (DFT) framework (Kohn and Sham, 1965) by using the Vienna ab initio simulation package (VASP) (Kresse and Furthmüller, 1996a). The ion–electron interaction was described by the projector-augmented wave (PAW) potentials (Blöchl, 1994). The generalized gradient approximation parameterized by Perdew, Burke, and Ernzerhof was utilized as the exchange-correlation functional (Perdew et al., 1996). The kinetic energy cutoff of 400 eV was adopted for the plane-wave expansion. The armchair-edged ribbon, zigzag-edged ribbon, and periodic graphene slab were sampled with 4 × 1 × 1, 1 × 4×1, and 4 × 4 × 1 Monkhorst−Pack k-point grids (Delley, 2000), respectively. During the geometry optimization, all atoms were relaxed until the total energy was converged to 1.0 × 10–5 eV/atom, and the force was converged to 0.01 eV/Å. In addition, we considered the van der Waals (vdW) interactions by employing the semiempirical DFT-D2 forcefield approach (Grimme, 2006).
The lattice parameters of 8.52 Å × 24.6 Å and 25.6 Å × 9.84 Å were set to model the armchair-edged graphene nanoribbon (including pyrrolic edge) and zigzag-edged graphene nanoribbon, respectively. The lattice parameters of 9.84 × 9.84Å were adopted to model the periodic graphene slab. Perpendicular to all graphene structures, a vacuum layer of 15 Å was set, which was sufficiently large to minimize the image interactions.
The adsorption energy
where Esubstrate+adsorbate is the total energy of the substrate with adsorbed molecules. Esubstrate and Eadsorbate are the energy of the isolated substrate and free molecule, respectively.
The computational hydrogen electrode (CHE) model (Norskov et al., 2004) was adopted to evaluate the free energy change during the CO2RR process. In the CHE model, the hydrogen atom is in equilibrium with the proton/electron pair at 298.15 K and 1 atm of pressure. In other words, the half chemical potential of gas-phase H2 is equal to that of a proton/electron pair at 0 V in an aqueous solution.
The Gibbs free energy change (ΔG) for each elementary CO2RR step involving proton/electron pair transfer was calculated by the expression (Norskov et al., 2004; Zuluaga and Stolbov, 2011):
where ΔE is the change of reaction energy based on DFT calculations. ΔZPE and ΔS are the change of zero-point energy and entropy, respectively. T refers to the temperature (298.15 K). The zero-point energy (ZPE) of adsorbates has been calculated from the vibrational frequencies. For the free molecules (CO2, CO, HCOOH, CH4, CH3OH, etc.) the vibrational frequencies and entropies are obtained from the NIST database (http://webbook.nist.gov/chemistry/). ΔGU = -neU, where n is the number of transferred electrons, e is the elementary charge of an electron, and U is the electrode potential vs. RHE. ΔGpH= 2.303 kBT *pH, kB is the Boltzmann constant. In this work, the value of pH was set as 0 for the acid medium (Faccio et al., 2010; Shang et al., 2010). Approximate solvation corrections with a dielectric constant of ε = 80 are applied for the simulation of an aqueous environment (Mathew et al., 2019).
In previous reports, the N-doped graphene materials have been widely studied as ORR electrocatalysts, which showed better stability and tolerance to methanol crossover effect than commercial Pt/C catalyst (Geng et al., 2011; Lin et al., 2013; Gong et al., 2009; Qu et al., 2010). Under different temperatures, the synthesizability of each type of the N-doped graphene materials is different. It is relatively easy to synthesize different types of N-doped graphene by controlling the temperature (Lin et al., 2013). The studied structures include five N-doped armchair graphene types, four N-doped zigzag graphene types, in-plane graphitic N (GN), and pyrrolic edge N (PyrroN)-doped graphene. For N-doped armchair graphene, it includes graphitic N (A-GN), pyridinic N (A-pyN), hydrogenated pyridinic N (A-pyN-H), oxidized pyridinic N (A-pyN-O), and pyridinic N hydroxide (A-pyN-OH), as shown in Figure 1. For N-doped zigzag graphene, four structures are considered, i.e., graphitic N (Z-GN), pyridinic N (Z-pyN), hydrogenated pyridinic N (Z-pyN-H), and oxidized pyridinic N (Z-pyN-O). These doped structures could be generated at high temperatures in the pyrolysis process of N-containing compounds (Wu et al., 2011; Li et al., 2012; Wang Q. et al., 2014; Holby et al., 2014).
FIGURE 1. The structures of N-doped graphene. The gray, blue, white, and red balls represent C, N, H, and O atoms, respectively.
During the CO2RR process on the studied compounds, the intermediates mainly include CO2, COOH, HCOO, HCOOH, CO + H2O, COHOH, H2COO, and COH + H2O. By exploring different adsorption sites (N and its adjacent carbon atoms), the most favorable adsorption configurations and sites are obtained (Supplementary Figures 1–8). Since the two main reactions on various N-doped graphene are HCOOH and CO generation pathways, we focus on the adsorption energies of CO2, COOH, HCOO, HCOOH, and CO as listed in Table 1, together with the bond distance between the adsorbed intermediates and catalyst surface. It is seen that the adsorption of CO2 molecule is weak all the time (-0.06 eV∼ -0.13 eV), and linear structure is maintained above the surface. To achieve high selectivity for HCOOH or CO, COOH (or HCOO) should be adsorbed strongly, but HCOOH or CO should be adsorbed weakly for desorption. Therefore, strong COOH (HCOO) binding but weak HCOOH (CO) adsorption is essential for the formation of HCOOH or (CO) (Sharma et al., 2015; Wu et al., 2015).
TABLE 1. The calculated adsorption energies (Eads, eV) and the shortest distances (d, Å) between the intermediate and N-doped graphene.
As shown in Supplementary Figures 2, 3, COOH could not be absorbed on GN and PyrroN, and is weakly adsorbed on A-pyN-H (-0.20 eV), Z-pyN-H (-0.43 eV), and Z-pyN-O (−0.59 eV) (Table 1). For the remaining structures, the adsorption of COOH is relatively strong, with the adsorption energy ranging from −0.95 to −2.48 eV. However, HCOO exists only on four N-doped graphene structures, that is, A-pyN, A-pyN-O, A-pyN-OH, and Z-GN. The adsorption energies for the four structures are in the range of −1.87 eV∼ −0.84 eV (Table 1).
For HCOOH, the adsorption energies for the studied compounds are in the range of −0.43 ∼ −0.06 eV, which are relatively weak and facilitate its desorption from the catalyst surface. Similar to the HCOOH molecule, the adsorption energies of CO are in the range of −0.26 ∼ −0.03 eV (Table 1).
The possible reaction pathways for the studied compounds are summarized in Figure 2. Based on the computational hydrogen electrode (CHE) model (Norskov et al., 2004), the limiting potential is obtained by UL = −ΔGMAX/e, where ΔGMAX denotes the maximum free energy difference between the two successive reaction steps. The reduction step corresponding to the limiting potential is defined as the potential determining step (PDS).
FIGURE 2. The free energy change for reaction pathways of CO2RR on various N-doped graphene. (A) A-GN, (B) A-pyN, (C) A-pyN-H, (D) A-pyN-O, (E) A-pyN-OH, (F) Z-GN, (G) Z-pyN, (H) Z-pyN-H, (I) Z-pyN-O, (J) GN.
As shown in Figures 2A–E, the energy of CO2 increases by 0.25–0.37 eV from the free molecule to the adsorbed state. After CO2 is adsorbed on the catalyst surface, it would be hydrogenated by (H+ + e−) pair. The formation of an O-H bond would produce COOH, while the formation of the C-H bond would generate the HCOO intermediate.
The reaction of CO2+H++e−→*COOH on A-GN, A-pyN, A-pyN-H, A-pyN-O, and A-pyN-OH is uphill by 1.16, 0.84, 2.14, 1.38, and 1.41 eV, respectively. For CO2+ H++e−→*HCOO, the energy increases by 1.73, 1.38, and 1.81 eV for A-pyN, A-pyN-O, and A-pyN-OH, respectively.
The hydrogenation of COOH would generate COHOH, HCOOH, and CO + H2O. Due to the large energy increase for producing COHOH, that is, 1.55, 0.96, and 1.26 eV for A-GN, A-pyN, and A-pyN-OH, respectively, further discussion is omitted. In COOH, if the OH moiety binds (H++e−), it would produce CO + H2O. If the carbon atom in COOH binds (H++e−), it would produce HCOOH. The production of HCOOH and CO is all thermodynamically downhill.
Similarly, the hydrogenation of HCOO may produce H2COO and HCOOH. As *HCOO→*H2COO step is endothermic with a large free energy increase (0.88 eV for A-pyN, 0.93 eV for A-pyN-O, and 1.86 eV for A-pyN-OH), further discussion is not provided. Thus, the final product from HCOO is HCOOH.
As illustrated in Figures 2A–E, the COOH intermediate has better performance in producing HCOOH than HCOO. For CO2→ *CO2→*COOH→*HCOOH/*CO, the PDS is *CO2→*COOH (Table 2), which is in agreement with the previous study (Wu et al., 2015). According to the free energy barrier (Figures 2A–E), A-pyN exhibits the highest catalytic activity toward HCOOH with a free energy barrier of 0.84 eV (Figure 2B). The order of catalytic activity for COOH to HCOOH/CO is A-pyN > A-GN > A-pyN-O > A-pyN-OH > A-pyN-H. In addition, CO2→ *CO2→*COOH→*CO+*H2O is the secondary pathway with slightly larger endothermic energy than CO2→ *CO2→*COOH→*HCOOH.
TABLE 2. Potential determining steps (PDSs), limiting potentials (UL/V), and overpotentials (ƞ/V) for CO2RR on Z-GN and Z-pyN. U0 is the equilibrium potential. Comparison has been made with previous studies. UL, U0, and ƞ are all vs. the RHE.
The reaction pathways on N-doped zigzag graphene nanoribbons (Figures 2F–I) are similar to those on N-doped armchair graphene nanoribbons. The HCOO intermediate could only stably exist on Z-GN among these N-doped zigzag graphene nanoribbons. To produce HCOOH, the CO2→*CO2→*COOH→*HCOOH pathway is more favorable than the CO2→*CO2→*HCOO→*HCOOH pathway (Figure 2F). In particular, on Z-GN, the hydrogenation of HCOO generates not only HCOOH but also O + HCHO with an energy barrier of 0.40 eV (Figure 2F). As illustrated in Figure 3, after the formation of O + HCOO, the remaining O atom could be easily hydrogenated into water due to the downhill process. The PDS for producing HCHO is the HCOO formation step with UL = -0.81 V.
For the *CO2→*COOH step, it occurred on Z-GN and Z-pyN most easily, in which the energy is uphill by 0.39 eV for Z-GN and downhill by -0.11 eV for Z-pyN, respectively (Figures 2F,G). While for the other two structures, large uphill energy barriers are required, that is, 1.88 eV for Z-pyN-H and 1.72 eV for Z-pyN-O, respectively. After the formation of COOH, its hydrogenation may generate HCOOH, CO + H2O, or COHOH, in which the formation of HCOOH is the most favorable, followed by CO + H2O and COHOH. Our calculations indicated that the COOH intermediate on Z-pyN needs an energy barrier of 0.83 eV to form COHOH (Figure 4). After the formation of COHOH, an energy increase of 0.41 eV is required to produce COH + H2O. The further hydrogenation of COH is relatively easy due to the downhill energy process to release the two competitive final products, that is, CH3OH and CH4. A previous study indicated that the formation of CH4 and CH3OH is through CO intermediate (Hori et al., 2008), which is different from our results.
FIGURE 4. The free energy change for the reaction of CO2 + 8H+ + 8e−→ CH4 + H2O and CO2 + 6H+ + 6e−→ CH3OH + H2O on Z-pyN.
As mentioned above, the pyrrolic N-doped structure has no catalytic activity for CO2RR. For GN, the free energy increase is the largest among all the N-doped graphene structures (2.55 eV). Thus, the catalytic activity of GN is omitted.
In a word, for the studied structures, the most favorable product is HCOOH, followed by CO and COHOH. In particular, the formation of HCOOH and CO is competitive since the free energy of CO is more thermodynamically favorable by only about 0.20 eV than that of HCOOH. This energy difference is similar to the value of 0.28 eV reported earlier (Liu et al., 2016). In a word, Z-pyN and Z-GN possess the highest catalytic activity toward HCOOH due to the smallest limiting potential of −0.38 and −0.39 V, respectively (Table 2), which is lower than −0.44 for PyrroN3 (Liu et al., 2016).
Hydrogen evolution reaction (HER) is the competitive reaction for CO2RR since the evolution of H would consume the proton–electron pair (H++e−) and passivate the catalytic activity of CO2RR. For the studied structures, the results showed that Z-pyN-O and Z-pyN have large energetic downhill for the adsorption of H*, indicating the enhanced HER in thermodynamic (Figure 5). For Z-GN and A-pyN, they have a negligible free energy barrier (0.03 and 0.04 eV) of H*. For the remaining structures, HER is hindered by large free energy barriers. Therefore, for the most favorable Z-pyN and Z-GN, CO2RR would be suppressed by HER. However, by choosing a suitable electrolyte, the activation energy of HER would be increased. For instance, according to the expression ΔGpH= 2.303kBT pH, in which pH = 0 is selected in the above study, ΔGpH = 0.42 eV is obtained for pH = 7.0. Thus, the activation energy of HER on Z-GN would be increased from −0.03 to 0.39 eV, comparable to the free energy barrier of 0.38 eV in the CO2RR process. Thus, the HER could be suppressed by increasing the pH value for Z-GN. While for Z-pyN, CO2RR is passivated by too strong HER. In a word, Z-GN could be selected as a promising nonmetal electrocatalyst for CO2RR in generating HCOOH.
FIGURE 5. The free energy changes for hydrogen evolution reaction (HER) on various N-doped graphene.
We have performed the DFT method to elucidate the reaction mechanism and activity of CO2RR on 11 types of N-doped graphene catalysts. It indicates that for all the studied structures, the formation of HCOOH is the most favorable, followed by CO. Among these structures, Z-pyN- and Z-GN-doped graphene exhibit the best catalytic activity for producing HCOOH with free energy barriers of 0.38 and 0.39 eV, respectively. The potential determining step (PDS) is CO2→*CO2 for Z-pyN and *CO2→*COOH for Z-GN, respectively. Meanwhile, CO is the competitive product which lies 0.20 eV above HCOOH. For the zigzag pyridinic N-doped graphene, it could also produce CH3OH and CH4 as the minor products which need to overcome an energy barrier of 0.83 eV. The minor product for the zigzag graphitic N-doped graphene is HCHO, with an energy barrier of 0.81 eV. However, for Z-pyN, CO2RR is passivated by too strong HER. Meanwhile, by modifying the pH value of electrolyte, Z-GN could be selected as a promising nonmetal electrocatalyst for CO2RR in generating HCOOH.
The original contributions presented in the study are included in the article/Supplementary Material; further inquiries can be directed to the corresponding author.
The author confirms being the sole contributor to this work and has approved it for publication.
We are grateful for funding support from the National Key R&D Program of China (2019YFA0308000), the Natural Science Foundation of China (no. 21873050), and the Priority Academic Program Development of Jiangsu Higher Education Institutions.
The author declares that the research was conducted in the absence of any commercial or financial relationships that could be construed as a potential conflict of interest.
All claims expressed in this article are solely those of the authors and do not necessarily represent those of their affiliated organizations, or those of the publisher, the editors and the reviewers. Any product that may be evaluated in this article, or claim that may be made by its manufacturer, is not guaranteed or endorsed by the publisher.
The Supplementary Material for this article can be found online at: https://www.frontiersin.org/articles/10.3389/fchem.2021.734460/full#supplementary-material
Appel, A. M., Bercaw, J. E., Bocarsly, A. B., Dobbek, H., DuBois, D. L., Dupuis, M., et al. (2013). Frontiers, Opportunities, and Challenges in Biochemical and Chemical Catalysis of CO2Fixation. Chem. Rev. 113 (8), 6621–6658. doi:10.1021/cr300463y
Bai, X., Chen, W., Zhao, C., Li, S., Song, Y., Ge, R., et al. (2017). Exclusive Formation of Formic Acid from CO2 Electroreduction by a Tunable Pd-Sn Alloy. Angew. Chem. 129 (40), 12387–12391. doi:10.1002/ange.201707098
Blöchl, P. E. (1994). Projector Augmented-Wave Method. Phys. Rev. B 50 (24), 17953–17979. doi:10.1103/PhysRevB.50.17953
Cook, T. R., Dogutan, D. K., Reece, S. Y., Surendranath, Y., Teets, T. S., and Nocera, D. G. (2010). Solar Energy Supply and Storage for the Legacy and Nonlegacy Worlds. Chem. Rev. 110 (11), 6474–6502. doi:10.1021/cr100246c
Delley, B. (2000). From Molecules to Solids with the DMol3 Approach. J. Chem. Phys. 113 (18), 7756–7764. doi:10.1063/1.1316015
Faccio, R., Fernández-Werner, L., Pardo, H., Goyenola, C., Ventura, O. N., and Mombrú, Á. W. (2010). Electronic and Structural Distortions in Graphene Induced by Carbon Vacancies and Boron Doping. J. Phys. Chem. C 114 (44), 18961–18971. doi:10.1021/jp106764h
Gao, D., Zhou, H., Wang, J., Miao, S., Yang, F., Wang, G., et al. (2015). Size-Dependent Electrocatalytic Reduction of CO2over Pd Nanoparticles. J. Am. Chem. Soc. 137 (13), 4288–4291. doi:10.1021/jacs.5b00046
Geng, D., Chen, Y., Chen, Y., Li, Y., Li, R., Sun, X., et al. (2011). High Oxygen-Reduction Activity and Durability of Nitrogen-Doped Graphene. Energy Environ. Sci. 4, 760–764. doi:10.1039/C0EE00326C
Gong, K., Du, F., Xia, Z., Durstock, M., and Dai, L. (2009). Nitrogen-Doped Carbon Nanotube Arrays with High Electrocatalytic Activity for Oxygen Reduction. Science 323 (5915), 760–764. doi:10.1126/science.1168049
Grimme, S. (2006). Semiempirical GGA-type Density Functional Constructed with a Long-Range Dispersion Correction. J. Comput. Chem. 27 (15), 1787–1799. doi:10.1002/jcc.20495
Holby, E. F., Wu, G., Zelenay, P., and Taylor, C. D. (2014). Structure of Fe-Nx-C Defects in Oxygen Reduction Reaction Catalysts from First-Principles Modeling. J. Phys. Chem. C 118 (26), 14388–14393. doi:10.1021/jp503266h
Hori, Y., Kikuchi, K., Kikuchi, S., and Suzuki, S. (1985). Production of Co and Ch4in Electrochemical Reduction of Co2at Metal Electrodes in Aqueous Hydrogencarbonate Solution. Chem. Lett. 14 (11), 1695–1698. doi:10.1246/cl.1985.1695
Hori, Y., Kikuchi, K., Murata, A., and Suzuki, S. (1986). Production of Methane and Ethylene in Electrochemical Reduction of Carbon Dioxide at Copper Electrode in Aqueous Hydrogencarbonate Solution. Chem. Lett. 15 (6), 897–898. doi:10.1246/cl.1986.897
Hori, Y., Vayenas, C. G., White, R. E., and Gamboa-Aldeco, M. E. (2008). Electrochemical CO2 Reduction on Metal Electrodes. New York: Springer, 89–189. doi:10.1007/978-0-387-49489-0_342
Kang, P., Zhang, S., Meyer, T. J., and Brookhart, M. (2014). Rapid Selective Electrocatalytic Reduction of Carbon Dioxide to Formate by an Iridium Pincer Catalyst Immobilized on Carbon Nanotube Electrodes. Angew. Chem. Int. Ed. 53 (33), 8709–8713. doi:10.1002/anie.201310722
Kim, C., Jeon, H. S., Eom, T., Jee, M. S., Kim, H., Friend, C. M., et al. (2015). Achieving Selective and Efficient Electrocatalytic Activity for CO2 Reduction Using Immobilized Silver Nanoparticles. J. Am. Chem. Soc. 137 (43), 13844–13850. doi:10.1021/jacs.5b06568
Kim, D., Resasco, J., Yu, Y., Asiri, A. M., and Yang, P. (2014). Synergistic Geometric and Electronic Effects for Electrochemical Reduction of Carbon Dioxide Using Gold-Copper Bimetallic Nanoparticles. Nat. Commun. 5, 4948. doi:10.1038/ncomms5948
Kohn, W., and Sham, L. J. (1965). Self-Consistent Equations Including Exchange and Correlation Effects. Phys. Rev. 140 (4A), A1133–A1138. doi:10.1103/PhysRev.140.A1133
Kondratenko, E. V., Mul, G., Baltrusaitis, J., Larrazábal, G. O., and Pérez-Ramírez, J. (2013). Status and Perspectives of CO2 Conversion into Fuels and Chemicals by Catalytic, Photocatalytic and Electrocatalytic Processes. Energ. Environ. Sci. 6 (11), 3112–3135. doi:10.1039/C3EE41272E
Kresse, G., and Furthmüller, J. (1996b). Efficiency of Ab-Initio Total Energy Calculations for Metals and Semiconductors Using a Plane-Wave Basis Set. Comput. Mater. Sci. 6 (1), 15–50. doi:10.1016/0927
Kresse, G., and Furthmüller, J. (1996a). Efficient Iterative Schemes Forab Initiototal-Energy Calculations Using a Plane-Wave Basis Set. Phys. Rev. B 54 (16), 11169–11186. doi:10.1103/PhysRevB.54.11169
Kumar, B., Asadi, M., Pisasale, D., Sinha-Ray, S., Rosen, B. A., Haasch, R., et al. (2013). Renewable and Metal-free Carbon Nanofibre Catalysts for Carbon Dioxide Reduction. Nat. Commun. 4, 2819. doi:10.1038/ncomms3819
Lee, S., Ocon, J. D., Son, Y.-i., and Lee, J. (2015). Alkaline CO2 Electrolysis toward Selective and Continuous HCOO- Production over SnO2 Nanocatalysts. J. Phys. Chem. C 119 (9), 4884–4890. doi:10.1021/jp512436w
Lewis, N. S., Nocera, D. G., and NoceraD, G. (2006). Powering the Planet: Chemical Challenges in Solar Energy Utilization. Proc. Natl. Acad. Sci. 103 (43), 15729–15735. doi:10.1073/pnas.0603395103
Li, W., Wu, J., Higgins, D. C., Choi, J.-Y., and Chen, Z. (2012). Determination of Iron Active Sites in Pyrolyzed Iron-Based Catalysts for the Oxygen Reduction Reaction. ACS Catal. 2 (12), 2761–2768. doi:10.1021/cs300579b
Lim, R. J., Xie, M., Sk, M. A., Lee, J.-M., Fisher, A., Wang, X., et al. (2014). A Review on the Electrochemical Reduction of CO2 in Fuel Cells, Metal Electrodes and Molecular Catalysts. Catal. Today 233, 169–180. doi:10.1016/j.cattod.2013.11.037
Lin, Z., Waller, G. H., Liu, Y., Liu, M., and Wong, C.-p. (2013). 3D Nitrogen-Doped Graphene Prepared by Pyrolysis of Graphene Oxide with Polypyrrole for Electrocatalysis of Oxygen Reduction Reaction. Nano Energy 2, 241–248. doi:10.1016/j.nanoen.2012.09.002
Liu, Y., Zhao, J., and Cai, Q. (2016). Pyrrolic-nitrogen Doped Graphene: a Metal-free Electrocatalyst with High Efficiency and Selectivity for the Reduction of Carbon Dioxide to Formic Acid: a Computational Study. Phys. Chem. Chem. Phys. 18, 5491–5498. doi:10.1039/C5CP07458D
Lu, Z. (2021). Computational Discovery of Energy Materials in the Era of Big Data and Machine Learning: A Critical Review. Mater. Rep. Energ., 100047. doi:10.1016/j.matre.2021.100047
Lu, Z., Yang, Z., Li, C., Wang, K., Han, J., Tong, P., et al. (2021). Modulating Nanoinhomogeneity at Electrode-Solid Electrolyte Interfaces for Dendrite‐Proof Solid‐State Batteries and Long‐Life Memristors. Adv. Energ. Mater. 11 (16), 2003811. doi:10.1002/aenm.202003811
Mathew, K., Kolluru, V. S. C., Mula, S., Steinmann, S. N., and Hennig, R. G. (2019). Implicit Self-Consistent Electrolyte Model in Plane-Wave Density-Functional Theory. J. Chem. Phys. 151 (23), 234101. doi:10.1063/1.5132354
Nie, X., Esopi, M. R., Janik, M. J., and Asthagiri, A. (2013). Selectivity of CO2Reduction on Copper Electrodes: The Role of the Kinetics of Elementary Steps. Angew. Chem. Int. Ed. 52 (9), 2459–2462. doi:10.1002/anie.201208320
Nørskov, J. K., Rossmeisl, J., Logadottir, A., Lindqvist, L., Kitchin, J. R., Bligaard, T., et al. (2004). Origin of the Overpotential for Oxygen Reduction at a Fuel-Cell Cathode. J. Phys. Chem. B 108 (46), 17886–17892. doi:10.1021/jp047349j
Perdew, J. P., Burke, K., and Ernzerhof, M. (1996). Generalized Gradient Approximation Made Simple. Phys. Rev. Lett. 77 (18), 3865–3868. doi:10.1103/PhysRevLett.77.3865
Qu, L., Liu, Y., Baek, J.-B., and Dai, L. (2010). Nitrogen-Doped Graphene as Efficient Metal-free Electrocatalyst for Oxygen Reduction in Fuel Cells. ACS Nano 4 (3), 1321–1326. doi:10.1021/nn901850u
Ren, D., Deng, Y., Handoko, A. D., Chen, C. S., Malkhandi, S., and Yeo, B. S. (2015). Selective Electrochemical Reduction of Carbon Dioxide to Ethylene and Ethanol on Copper(I) Oxide Catalysts. ACS Catal. 5 (5), 2814–2821. doi:10.1021/cs502128q
Shang, Y., Zhao, J.-x., Wu, H., Cai, Q.-h., Wang, X.-g., and Wang, X.-z. (2010). Chemical Functionalization of Pyridine-like and Porphyrin-like Nitrogen-Doped Carbon (CN X ) Nanotubes with Transition Metal (TM) Atoms: a Theoretical Study. Theor. Chem. Acc. 127 (5-6), 727–733. doi:10.1007/s00214-010-0784-9
Sharma, P. P., Wu, J., Yadav, R. M., Liu, M., Wright, C. J., Tiwary, C. S., et al. (2015). Nitrogen-Doped Carbon Nanotube Arrays for High-Efficiency Electrochemical Reduction of CO2: On the Understanding of Defects, Defect Density, and Selectivity. Angew. Chem. Int. Ed. 54 (46), 13701–13705. doi:10.1002/anie.201506062
Sun, X., Kang, X., Zhu, Q., Ma, J., Yang, G., Liu, Z., et al. (2016). Very Highly Efficient Reduction of CO2to CH4using Metal-free N-Doped Carbon Electrodes. Chem. Sci. 7, 2883–2887. doi:10.1039/C5SC04158A
Thomas, C. D., Cameron, A., Green, R. E., Bakkenes, M., Beaumont, L. J., Collingham, Y. C., et al. (2004). Extinction Risk from Climate Change. Nature 427 (6970), 145–148. doi:10.1038/nature02121
Wang, H., Chen, Y., Hou, X., Ma, C., and Tan, T. (2016). Nitrogen-doped Graphenes as Efficient Electrocatalysts for the Selective Reduction of Carbon Dioxide to Formate in Aqueous Solution. Green. Chem. 18, 3250–3256. doi:10.1039/C6GC00410E
Wang, H., Jia, J., Song, P., Wang, Q., Li, D., Min, S., et al. (2017). Efficient Electrocatalytic Reduction of CO2by Nitrogen-Doped Nanoporous Carbon/Carbon Nanotube Membranes: A Step towards the Electrochemical CO2Refinery. Angew. Chem. Int. Ed. 56 (27), 7847–7852. doi:10.1002/anie.201703720
Wang, Q., Zhou, Z.-Y., Lai, Y.-J., You, Y., Liu, J.-G., Wu, X.-L., et al. (2014b). Phenylenediamine-Based FeNx/C Catalyst with High Activity for Oxygen Reduction in Acid Medium and its Active-Site Probing. J. Am. Chem. Soc. 136 (31), 10882–10885. doi:10.1021/ja505777v
Wang, X., Niu, H., Liu, Y., Shao, C., Robertson, J., Zhang, Z., et al. (2020). Theoretical Investigation on Graphene-Supported Single-Atom Catalysts for Electrochemical CO2 Reduction. Catal. Sci. Technol. 10 (24), 8465–8472. doi:10.1039/d0cy01870h
Wang, X., Sun, G., Routh, P., Kim, D.-H., Huang, W., and Chen, P. (2014a). Heteroatom-doped Graphene Materials: Syntheses, Properties and Applications. Chem. Soc. Rev. 43, 7067–7098. doi:10.1039/C4CS00141A
Wu, G., More, K. L., Johnston, C. M., and Zelenay, P. (2011). High-Performance Electrocatalysts for Oxygen Reduction Derived from Polyaniline, Iron, and Cobalt. Science 332 (6028), 443–447. doi:10.1126/science.1200832
Wu, J., Liu, M., Sharma, P. P., Liu, C. S., Zou, X., Zhou, X. D., et al. (2015). Achieving Highly Efficient, Selective, and Stable CO2 Reduction on Nitrogen-Doped Carbon Nanotubes. ACS Nano 9 (5), 5364–5371. doi:10.1021/acsnano.5b01079
Wu, J., Ma, S., Sun, J., Gold, J. I., Tiwary, C., Kim, B., et al. (2016). A Metal-free Electrocatalyst for Carbon Dioxide Reduction to Multi-Carbon Hydrocarbons and Oxygenates. Nat. Commun. 7, 13869. doi:10.1038/ncomms13869
Yi, J., Chen, J., Yang, Z., Dai, Y., Li, W., Cui, J., et al. (2019). Facile Patterning of Laser‐Induced Graphene with Tailored Li Nucleation Kinetics for Stable Lithium‐Metal Batteries. Adv. Energ. Mater. 9 (38), 1901796. doi:10.1002/aenm.201901796
Zhang, S., Kang, P., and Meyer, T. J. (2014a). Nanostructured Tin Catalysts for Selective Electrochemical Reduction of Carbon Dioxide to Formate. J. Am. Chem. Soc. 136 (5), 1734–1737. doi:10.1021/ja4113885
Zhang, S., Kang, P., Ubnoske, S., Brennaman, M. K., Song, N., House, R. L., et al. (2014b). Polyethylenimine-Enhanced Electrocatalytic Reduction of CO2to Formate at Nitrogen-Doped Carbon Nanomaterials. J. Am. Chem. Soc. 136 (22), 7845–7848. doi:10.1021/ja5031529
Zhu, D. D., Liu, J. L., and Qiao, S. Z. (2016). Recent Advances in Inorganic Heterogeneous Electrocatalysts for Reduction of Carbon Dioxide. Adv. Mater. 28 (18), 3423–3452. doi:10.1002/adma.201504766
Zhu, W., Metin, Ö., Lv, H., Guo, S., Wright, C. J., Sun, X., et al. (2013). Monodisperse Au Nanoparticles for Selective Electrocatalytic Reduction of CO2 to CO. J. Am. Chem. Soc. 135 (45), 16833–16836. doi:10.1021/ja409445p
Keywords: density functional theory, N-doped graphene, CO2 reduction reaction, catalytic activity, Gibbs free energy
Citation: Sun X (2021) Achieving Selective and Efficient Electrocatalytic Activity for CO2 Reduction on N-Doped Graphene. Front. Chem. 9:734460. doi: 10.3389/fchem.2021.734460
Received: 01 July 2021; Accepted: 12 July 2021;
Published: 19 August 2021.
Edited by:
Zhaofu Zhang, University of Cambridge, United KingdomReviewed by:
Liu Xuefei, Guizhou Normal University, ChinaCopyright © 2021 Sun. This is an open-access article distributed under the terms of the Creative Commons Attribution License (CC BY). The use, distribution or reproduction in other forums is permitted, provided the original author(s) and the copyright owner(s) are credited and that the original publication in this journal is cited, in accordance with accepted academic practice. No use, distribution or reproduction is permitted which does not comply with these terms.
*Correspondence: Xiaoxu Sun, eHhzdW5AY2lhYy5hYy5jbg==
Disclaimer: All claims expressed in this article are solely those of the authors and do not necessarily represent those of their affiliated organizations, or those of the publisher, the editors and the reviewers. Any product that may be evaluated in this article or claim that may be made by its manufacturer is not guaranteed or endorsed by the publisher.
Research integrity at Frontiers
Learn more about the work of our research integrity team to safeguard the quality of each article we publish.