- 1Industry 4.0 Convergence Bionics Engineering, Pukyong National University, Busan, South Korea
- 2Department of Chemistry and Nanoscience, Ewha Womans University, Seoul, South Korea
- 3Department of Chemistry, Pusan National University, Busan, South Korea
- 4Department of Energy Systems Research, Ajou University, Suwon, South Korea
- 5Department of Chemistry, Pukyong National University, Busan, South Korea
- 6Department of Chemistry, Ajou University, Suwon, South Korea
The ability to detect hypochlorite (HOCl/ClO−) in vivo is of great importance to identify and visualize infection. Here, we report the use of imidazoline-2-thione (R1SR2) probes, which act to both sense ClO− and kill bacteria. The N2C=S moieties can recognize ClO− among various typical reactive oxygen species (ROS) and turn into imidazolium moieties (R1IR2) via desulfurization. This was observed through UV–vis absorption and fluorescence emission spectroscopy, with a high fluorescence emission quantum yield (ՓF = 43–99%) and large Stokes shift (∆v∼115 nm). Furthermore, the DIM probe, which was prepared by treating the DSM probe with ClO−, also displayed antibacterial efficacy toward not only Escherichia coli (E. coli) and Staphylococcus aureus (S. aureus) but also methicillin-resistant Staphylococcus aureus (MRSA) and extended-spectrum ß-lactamase–producing Escherichia coli (ESBL-EC), that is, antibiotic-resistant bacteria. These results suggest that the DSM probe has great potential to carry out the dual roles of a fluorogenic probe and killer of bacteria.
Introduction
Invasion of microorganisms such as bacteria and viruses can cause infectious diseases. Due to the worldwide increase in cases of severe bacterial diseases, scientists have attempted to develop technologies that serve as both fluorogenic probes for the identification of infection and antibacterial agents. Unfortunately, the continued overuse of antibiotics coupled with the rapid spread of resistance mechanisms has rendered many antibiotics inactive (Kardas et al., 2005). As a result, new pathogens have come into being that are multidrug resistant (MDR), such as methicillin-resistant Staphylococcus aureus (MRSA) and extended-spectrum ß-lactamase–producing Escherichia coli (ESBL-EC), which have undermined most clinically useful antibiotics. Therefore, the emergence of drug-resistant bacteria is one of the growing challenges to anti-infection therapy. At the same time, emerging infectious diseases need very urgent and immediate treatment due to their rapid spread. In this regard, theragnostics, a treatment strategy that combines therapeutics with diagnostics, could be embraced by clinicians and patients (Pene et al., 2009). In recent years, material-based approaches have found preliminary use for the treatment of bacterial infections (Kurapati et al., 2016; Gupta et al., 2019) and for the image-guided treatment of bacterial infections (Kim et al., 2018; Lee S. et al., 2020). Such methods have provided an approach that can produce the desired therapeutic effect with a reduced potential to develop drug-resistant bacteria (Lee et al., 2016; Li et al., 2018a; Li et al., 2018b; Li et al., 2019). Among the various reactive oxygen species (ROS), hypochlorite (HOCl/ClO−) acts as a powerful microbicidal agent in the innate immune system. ClO− is mainly produced by the myeloperoxidase (MPO)-catalyzed reaction of H2O2 and Cl− in immunocytes (Domigan et al., 1995; Xu et al., 2013; Pak et al., 2018; Wu et al., 2019; Nguyen et al., 2020). The regulated production of microbicidal HOCl is required for the host to control invading microbes. On the other hand, OCl− reacts rapidly with a variety of biomolecules and is connected with various disorders (Winterbourn et al., 2000; Krasowska and Konat, 2004; Jeitner et al., 2005).
A fundamentally important yet challenging feature of studies in this area is the design of new chemorecognition processes. Imidazolium salts with good water solubility and stability have been used as fluorescence sensors in aqueous solution (Xu et al., 2010; Kim et al., 2012; Xu et al., 2015). To take advantage of the properties of imidazolium salts, we designed imidazoline-2-thione (R1SR2) probes to be ClO− fluorescent probes capable of inducing bacterial growth inhibition. Furthermore, the photophysical properties of R1IR2 and R1SR2 were examined via not only experimental results but also time-dependent DFT (TD-DFT) calculation. Bacterial growth was significantly reduced by the imidazolium moieties (R1IR2) that were generated by the treatment of R1SR2 with ClO−. Among the pairs of R1IR2 and R1SR2, the DSM probe showed excellent selectivity and sensitivity toward ClO−. Also, the DIM probe showed antibacterial efficacy toward not only E. coli and S. aureus but also methicillin-resistant S. aureus (MRSA) and extended-spectrum ß-lactamase–producing E. coli (ESBL-EC). The DIM probe initially induces electrostatic interactions between the cationic imidazolium salts and the negatively charged bacterial surface, followed by structural perturbation, resulting in bacterial cell death. Similar membrane disruption through interactions of cationic imidazolium groups has been suggested by previous reports (Riduan and Zhang, 2013). Overall, this report demonstrates the importance and benefits of the new fluorogenic probe DSM for anti-pathogenic diagnostic and therapeutic applications.
Experimental Design
For the synthesis of R1SR2, a mixture of R1IR2 (0.1 mmol), sulfur (1.0 mmol), and sodium methoxide (1.0 mmol) in anhydrous methanol (20 ml) was stirred overnight at room temperature. After the solvent was removed, the crude product was dissolved in DW and extracted by MC 3 times. The organic phase was collected and dried over Na2SO4. It was purified by silica gel column chromatography, using H/MC (9/1) as the eluent to get a white solid as the product (yield ∼90%).
BSB: 1H NMR (400 MHz, chloroform-d) δ 7.77 (dd, J = 6.3, 3.3 Hz, 2H), 7.69–7.61 (m, 2H), 7.41–7.32 (m, 4H), 7.20–7.09 (m, 4H), 6.97–6.90 (m, 2H), 5.80 (s, 4H). 13C NMR (101 MHz, chloroform-d) δ 174.85, 134.28, 133.18, 131.93, 130.55, 129.36, 128.02, 127.84, 127.82, 125.25, 122.77, 105.92, 48.67. ESI HRMS m/z = 536.9630 [M + H]+, calc. for C25H18Br2N2S = 535.96.
BSM: 1H NMR (400 MHz, chloroform-d) δ 7.92–7.85 (m, 1H), 7.81–7.73 (m, 1H), 7.67–7.59 (m, 1H), 7.53 (s, 1H), 7.40 (pd, J = 6.8, 1.6 Hz, 2H), 7.31 (s, 1H), 7.16–7.06 (m, 2H), 6.89–6.81 (m, 1H), 5.73 (s, 2H), 3.93 (d, J = 0.6 Hz, 3H). 13C NMR (101 MHz, chloroform-d) δ 174.26, 134.36, 133.07, 132.92, 131.88, 130.53, 130.46, 129.24, 127.94, 127.86, 127.83, 127.70, 125.21, 125.09, 122.67, 105.70, 105.04, 48.35, 31.68. ESI HRMS m/z = 383.0212 [M + H]+, calc. for C19H15BrN2S = 382.01.
CSB: 1H NMR (400 MHz, chloroform-d) δ 8.04 (dt, J = 7.8, 0.9 Hz, 2H), 7.75 (d, J = 7.5 Hz, 2H), 7.62 (dd, J = 7.7, 1.6 Hz, 1H), 7.48–7.33 (m, 6H), 7.24–7.17 (m, 4H), 7.08 (dtd, J = 16.7, 7.4, 1.7 Hz, 2H), 6.82–6.75 (m, 1H), 5.69 (s, 2H), 4.42 (td, J = 6.8, 2.1 Hz, 4H), 2.06 (dq, J = 31.4, 7.4 Hz, 4H). 13C NMR (101 MHz, chloroform-d) δ 140.43, 133.08, 129.23, 127.94, 127.75, 125.82, 125.11, 122.99, 120.51, 119.03, 108.77, 105.76, 105.04, 77.42, 77.10, 76.78, 48.33, 44.73, 42.65. ESI HRMS m/z = 612.1080 [M + Na]+, calc. for C34H28BrN3S = 589.12.
CSC: 1H NMR (400 MHz, chloroform-d) δ 8.03 (dt, J = 7.9, 1.0 Hz, 4H), 7.74 (dd, J = 6.3, 3.3 Hz, 2H), 7.46–7.35 (m, 10H), 7.23–7.13 (m, 6H), 4.34 (dt, J = 18.7, 6.8 Hz, 8H), 2.10–2.00 (m, 4H), 1.94 (q, J = 7.2 Hz, 4H), 1.30–1.20 (m, 4H), 0.90–0.79 (m, 4H). 13C NMR (101 MHz, chloroform-d) δ 172.90, 140.42, 131.88, 130.15, 127.69, 125.78, 124.96, 122.97, 120.49, 119.00, 108.77, 104.90, 77.42, 77.11, 76.79, 44.41, 42.64, 26.09, 25.31. ESI HRMS m/z = 665.2709 [M + Na]+, calc. for C43H38N4S = 642.28.
CSD: 1H NMR (600 MHz, chloroform-d) δ 8.03 (dt, J = 7.7, 1.0 Hz, 2H), 7.79 (d, J = 1.9 Hz, 1H), 7.75 (ddd, J = 8.1, 2.2, 1.2 Hz, 2H), 7.45–7.37 (m, 6H), 7.23–7.16 (m, 5H), 6.67–6.64 (m, 1H), 5.61 (s, 2H), 4.41 (q, J = 7.0 Hz, 4H), 2.14–1.96 (m, 4H). 13C NMR (101 MHz, chloroform-d) δ 173.81, 140.41, 135.38, 133.57, 131.64, 131.14, 130.29, 128.96, 127.78, 127.72, 125.82, 125.22, 123.16, 122.99, 121.99, 120.52, 119.05, 108.74, 105.56, 105.20, 77.42, 77.11, 76.79, 47.86, 44.76, 42.64, 26.05, 25.29. ESI HRMS m/z = 690.0185 [M + Na]+, calc. for C34H27Br2N3S = 667.03.
CSM: 1H NMR (400 MHz, chloroform-d) δ 8.04 (dt, J = 7.7, 1.0 Hz, 2H), 7.74 (dd, J = 6.6, 3.0 Hz, 2H), 7.62 (dd, J = 7.7, 1.5 Hz, 1H), 7.48–7.33 (m, 6H), 7.26 (s, 7H), 7.24–7.15 (m, 4H), 7.08 (dtd, J = 16.7, 7.4, 1.7 Hz, 2H), 6.78 (dd, J = 7.4, 1.9 Hz, 1H), 5.69 (s, 2H), 4.42 (dd, J = 7.3, 5.7 Hz, 4H), 2.10 (p, J = 6.8 Hz, 2H), 2.01 (p, J = 7.0 Hz, 2H). 13C NMR (101 MHz, chloroform-d) δ 173.25, 140.43, 132.81, 131.89, 130.28, 127.79, 127.63, 125.78, 125.03, 124.94, 122.96, 120.48, 119.00, 108.78, 104.91, 104.80, 44.47, 42.65, 31.31, 26.12, 25.38. ESI HRMS m/z = 458.1661 [M + Na]+, calc. for C28H25N3S = 435.18.
DSB: 1H NMR (400 MHz, chloroform-d) δ 7.84–7.73 (m, 3H), 7.69–7.60 (m, 1H), 7.43–7.34 (m, 3H), 7.33 (s, 1H), 7.31–7.22 (m, 3H), 7.20–7.09 (m, 2H), 6.96–6.89 (m, 1H), 6.82 (dd, J = 8.3, 0.8 Hz, 1H), 5.79 (s, 2H), 5.73 (s, 2H). 13C NMR (101 MHz, chloroform-d) δ 174.80, 135.49, 134.20, 133.54, 133.21, 131.87, 131.74, 131.22, 130.60, 130.54, 129.41, 129.08, 128.02, 127.84, 127.79, 125.38, 123.27, 122.78, 122.13, 106.09, 105.72, 48.70, 48.21. ESI HRMS m/z = 614.8735 [M + H]+, calc. for C25H17Br3N2S = 613.87.
DSD: 1H NMR (400 MHz, chloroform-d) δ 7.84–7.74 (m, 4H), 7.40 (dd, J = 6.3, 3.2 Hz, 2H), 7.34 (s, 2H), 7.28 (dd, J = 8.3, 1.9 Hz, 2H), 6.80 (dd, J = 8.3, 0.7 Hz, 2H), 5.72 (s, 4H). 13C NMR (101 MHz, chloroform-d) δ 174.73, 135.53, 133.44, 131.67, 131.22, 130.59, 129.05, 127.82, 125.50, 123.29, 122.20, 105.89, 48.24. ESI HRMS m/z = 692.7840 [M + H]+, calc. for C25H16Br4N2S = 691.78.
DSM: 1H NMR (400 MHz, chloroform-d) δ 7.93–7.85 (m, 1H), 7.79 (dd, J = 7.5, 2.0 Hz, 2H), 7.53 (s, 1H), 7.48–7.36 (m, 2H), 7.28 (s, 1H), 7.22 (d, J = 2.0 Hz, 3H), 6.74 (dd, J = 8.3, 0.8 Hz, 1H), 5.66 (s, 2H), 3.92 (s, 3H). 13C NMR (101 MHz, chloroform-d) δ 174.15, 135.38, 133.61, 132.83, 131.65, 131.12, 130.56, 130.44, 129.07, 127.84, 127.73, 125.34, 125.24, 123.19, 122.01, 105.51, 105.22, 47.89, 31.71. ESI HRMS m/z = 460.9317 [M + H]+, m/z = 482.9137 [M+Na]+ calc. for C19H15Br2N2S = 459.93.
Results and Discussion
Molecular Design, Synthesis, and Characterization
As shown in Scheme 1, R1IR2 was synthesized from 2,3-Diaminonaphthalene in a 3-step process as follows: imidazole cyclization, alkylation, and imidazolium salt formation. Several bromide and carbazole derivatives have shown antibacterial activity (Yaqub et al., 2013; Gottardi et al., 2014; Bashir et al., 2015; Liu et al., 2015; Salih et al., 2016; Popescu et al., 2021). Thus, the introduction of bromobenzyl, dibromobenzyl, and carbazole groups is expected to increase the antibacterial effect of the probes. Then, the R1IR2 salt was treated with sulfur and CH3ONa as a catalyst in ACN, leading to the formation of the corresponding molecule R1SR2. All synthetic processes and collected structures are detailed in the experimental section and the supporting information. Several products were characterized not only by 1H NMR, 1C NMR, and HRMS spectra (Supplementary Material) but also by crystallization structures (Figure 1; Supplementary Figures S37, S38; Supplementary Tables S1, S2), which have not been reported in previous studies on similar N2C=S structures (Xu et al., 2015; Xu et al., 2016). In particular, the length of the thioketone in the N2C=S type was found to be 1.657–1.671 Å (Table 1), which is longer than that in other thioketone types such as thiobenzophenone, thioformaldehyde, thioacetone, etc. (∼1.63–1.64 Å) due to the presence of C–N π-bonds (Mullen and Hellner, 1978; Allen et al., 1987). Thus, the N2C=S bond can make the compound more active and sensitive toward ROS/RNS.
To better understand not only the molecular structures but also the molecular orbitals and energy levels of R1IR2 and R1SR2, geometrical optimization was performed through theoretical DFT calculations in the Gaussian 09 program package using the B3LVPs functional with the 6-31+g(2d,p) basis set (Pham et al., 2020). The optimized structures without imaginary frequencies were similar to the crystallization structures in terms of several critical bond lengths and angles (Table 1). Their molecular orbitals and energy levels from HOMO+2 to LUMO+2 are shown in Supplementary Tables S1–S5. The HOMO of CIR2 is located in the carbazole moiety, and the LUMO is located in the naphthalene–imidazolium salt center core. However, the HOMO and LUMO of BIR2 and DIR2 are concentrated in the naphthalene–imidazolium salt core (Figure 2). Similarly, the HOMO of CSR2 is located in the carbazole moiety, whereas the HOMO and LUMO of BSR2 and DSR2 are located in the naphthalene and imidazoline-2-thione moieties. The difference originates from the introduction of the carbazole moiety, which is known as a strong donor and fluorescence quencher (Ledwon, 2019; Rehmat et al., 2020; Saritha et al., 2020). Thus, the energy gap between the LUMO and the HOMO (Eg) of CIR2 (1.80–2.03 eV) is significantly lower than those of BIR2 and DIR2 (3.77–3.86 eV). The Eg of CSR2 (3.79–3.86 eV) is lower than those of BIR2 and DIR2 (4.08–4.14 eV). The reduction in difference is assigned to the strong electron acceptor ability of imidazolium salts, which enhances electron transfer from the carbazole electron donor to the imidazolium salt electron acceptor (vs. from the carbazole to the naphthalene and imidazoline-2-thione moieties).
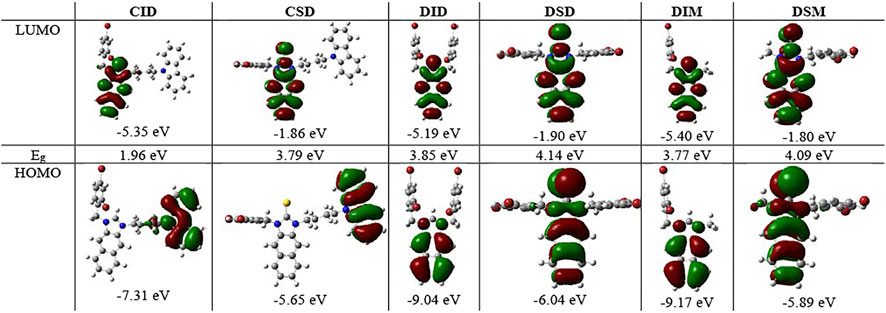
FIGURE 2. Molecular orbitals and associated energies of CID and CSD, DID and DSD, DIM and DSM, and the energy gap (Eg) between the HOMO and the LUMO.
Photophysical Properties and Theoretical Calculations
The UV–vis absorption and fluorescence emission spectroscopy of R1IR2 and R1SR2 were examined in various solvents (Supplementary Figures S39–S47). At the same time, time-dependent DFT (TD-DFT) calculations were carried out in the optimized structures using a hybrid functional method, a gradient-corrected method, and a popular local method (Adamo and Jacquemin, 2013; Pham et al., 2021a) to better understand their photophysical properties. The optical excitation energies of R1IR2 and R1SR2 were determined using the CAM-B3LYP functional with the Def-2-TZVP basis set and the TPSSTPSS functional with the 6-31+G (2 days, p) basis set, respectively. The results corresponded well to the experimental data. R1SR2 showed absorption peaks at approximately 350 nm, with a high molar absorption coefficient (ɛ = 31.4–62.8 × 103) and weak emission (ՓF = 0.1–1.8%) (Figure 3B; Table 2). On the other hand, R1IR2 exhibited absorption peaks at about 325 nm, with a lower molar absorption coefficient (ε = 7.2–13.3 ×103) (Figure 3A; Table 2). R1IR2 without a carbazole moiety showed a strong emission peak at approximately 440 nm (ՓF = 26–63%) and a large Stokes shift (∆v ∼ 115 nm). In sharp contrast, R1IR2 with a carbazole moiety exhibited weak emission (ՓF = 3.8–8.2%) due to photoinduced electron transfer (PET) from the carbazole donor to the naphthalene–imidazolium salt acceptor (Sun et al., 2019). The absorption of R1IR2 is assigned to the S0 → S1 transition and its emission is assigned to the S1′ → S0 transition, with the orbital contribution located in the naphthalene–imidazolium salt core (Supplementary Table S12). R1SR2 exhibited S0 → S3 absorption and S2′ → S1 emission in the absence of carbazole groups, while it showed S0 ↔ Sn/Sn′ transition at the higher level of Sn/Sn′ in the presence of carbazole groups. The absorption band is contributed by other orbitals in addition to the HOMO and the LUMO (Supplementary Table S13), but natural transition orbitals (NTOs) showed a similar electronic transition in the naphthalene and imidazoline-2-thione moieties of R1SR2 (Supplementary Table S14), which demonstrates that the two have the same UV/vis absorption spectra (Figure 3B).
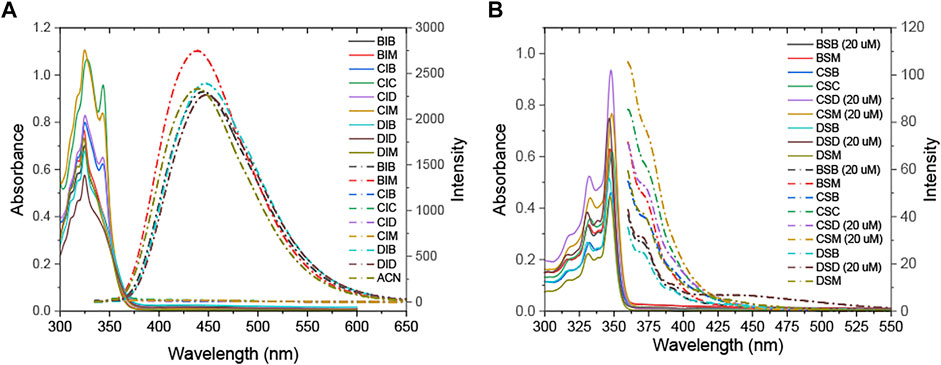
FIGURE 3. UV–vis (solid line) and fluorescence emission (dashed/dotted line) spectra of (A) R1IR2 (80 µM) and (B) R1SR2 (10 µM) (in ACN).
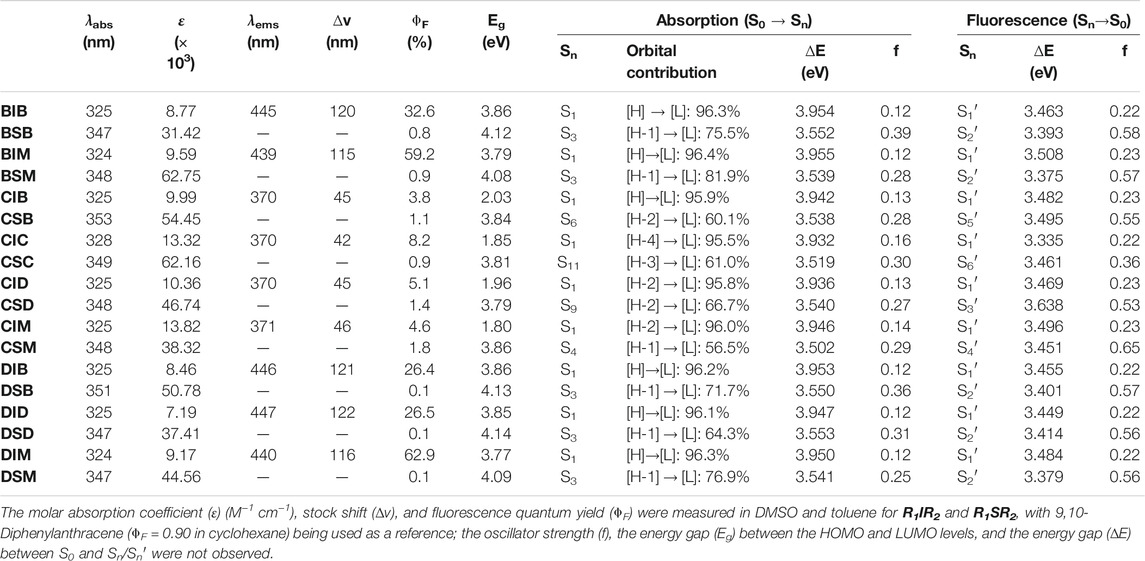
TABLE 2. Photophysical properties of R1IR2 and R1SR2 according to ACN and computational calculations.
The fluorescence emission quantum yield of BIM and DIM (ՓF = 59.2–62.9%) is significantly greater than that of BIB, DIB, and DID (26.4–32.6%) in DMSO, which is attributable to the absence or presence of the second (di)bromobenzyl group. The S1 absorption energy of BIB and BIM vs. DIB, DID, and DIM is similar, whereas the S1′ emission energy of BIM and DIM is higher than that of BIB and DIB or DID, respectively. Thus, the energy gap (∆E) between the S1 absorption and the S1′ emission of R1IM (R1 = B or D) is lower than that of R1IR2 (R1, R2 = B or D) (Figure 4). Moreover, the energy relaxation wastage of R1IM (R1 = B or D) from the S1 absorption level to the S1′ emission level is less than that of R1IR2 (R1, R2 = B or D), leading to the increase in fluorescence emission quantum yield of BIM and DIM.
At the different volume fractions of PBS buffer with a pH value of 7.4 (0–99.5%), the fluorescence emission of DIM and DID in DMF is maintained owing to the high water solubility of imidazolium salt groups (Figure 5B; Supplementary Figures S48, S49). We further examined their fluorescence emission in the aggregate state. Interestingly, the emission peak of DIM at 450 nm decreased, whereas a 335–350-nm emission band slightly increased with the increasing of toluene (Tol) concentration (0–99.5%) (Figure 5A). DIM showed the ACQ effect on its core structure in DMF being quenched in the high concentration of toluene (Supplementary Figure S49); at the same time, it showed blueshift emission assigned from the rotation of bromobenzyl groups around the imidazolium salt core.
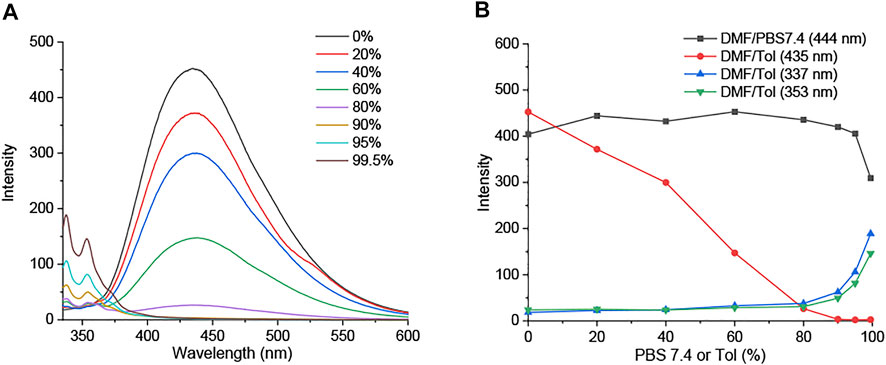
FIGURE 5. (A) Fluorescence emission spectra of DIM (5 µM) in DMF/Tol (0–99.5%). (B) Fluorescence intensity at emission wavelength of DIM (5 µM) in DMF/PBS buffer (pH 7.4) and DMF/Tol (0–99.5%).
Antibacterial Activity
To compare the antibacterial activity of R1IR2 and R1SR2, we calculated the concentration (µM) (CFU50) at which the CFU rate equals 50% and the P=CFU50(R1IR2)/CFU50(R1SR2) between the imidazolium salt and imidazoline-2-thiones (Table 3). All R1SR2 showed weak antibacterial ability toward E. coli, S. aureus, extended-spectrum ß-lactamase–producing E. coli (ESBL-EC), E. coli expressing green fluorescent protein (EC-GFP), and methicillin-resistant S. aureus (MRSA) bacteria with CFU50 > 128.0 µM. In sharp contrast, almost all imidazolium salts (R1IR2) exhibited a stronger antibacterial effect, including against ESBL-EC and MRSA (p > 2.4). The antibacterial efficiency of R1IR2 is quite similar to that of dehydroepiandrosterone-derived (Hryniewicka et al., 2021), peptide-conjugated (Reinhardt et al., 2014), ethoxyether-functionalized (Huang et al., 2011), amino acid–derived (Valls et al., 2020), polydiacetylene-conjugated (Lee et al., 2016), and unsymmetrically substituted (Çoban et al., 2017; Duman et al., 2019) imidazolium salts in previous reports. Furthermore, the antibacterial activity of imidazolium salts (R1IR2) increased following the substitution of methyl and bromobenzyl with dibromobenzyl groups and the change from one to two dibromobenzyl groups (Table 3).
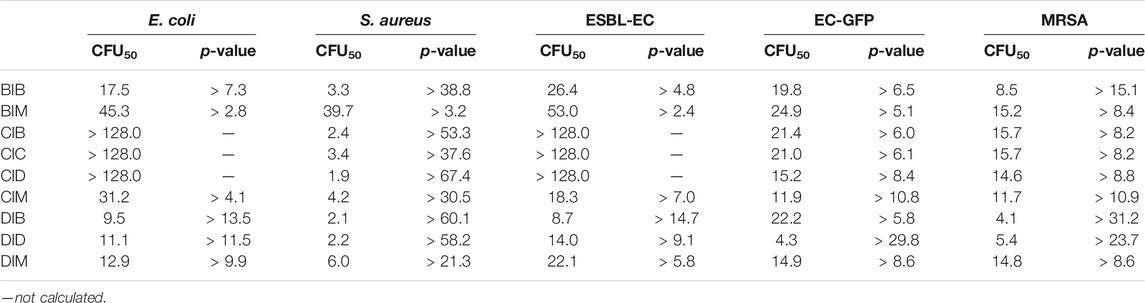
TABLE 3. CFU50 (µM) and p-value of R1IR2 and R1SR2 toward E. coli, S. aureus, ESBL-EC, EC-GFP, and MRSA bacteria (CFU50(R1SR2) > 128.0 µM).
On the other hand, the incorporation of dibromobenzyl groups enhanced the antibacterial effect toward Gram-positive bacteria such as S. aureus and MRSA. The introduction of a carbazole moiety increased the strength of the antibacterial effect toward S. aureus (CFU50 = 1.9–4.2 µM, p > 37.6), whereas the antibacterial ability was moderate toward EC-GFP and MRSA and weak toward E. coli and ESBL-EC (CFU50 > 128.0 µM). The negative amino group of carbazole affects the antibacterial ability of a positively charged imidazolium salt toward Gram-negative bacteria and enhances the antibacterial ability toward Gram-positive bacteria. In sum, the imidazolium salts DIM and DID showed strong antibacterial effects (p > 5.8) compared to the imidazoline-2-thiones (R1SR2) DSM and DSD, respectively. Thus, DSM and DSD were potentially selected for OFF-ON antibacterial fluorescent probes.
ClO− Response
Recognition of ROS/RNS by DSM was observed through UV–vis absorption and fluorescence emission spectroscopy in PBS buffer with a pH value of 7.4 (0.5% DMF). The absorbance band (300–375 nm) of DSM (5 µM) is decreased, and its blue fluorescence emission is significantly enhanced after 30 min of incubation in ClO− (50 µM). In sharp contrast, the UV–vis absorption spectra of DSM are slightly changed, and its fluorescence emission is quenched upon exposure to other types of ROS/RNS, even at higher concentrations (Figure 6A; Supplementary Figure S51). On the other hand, when DSD (5 µM) was treated with ClO− (0–160 µM), its fluorescence emission was also inhibited (Supplementary Figure S50A). DSD cannot react to ClO− owing to steric hindrance between the two dibromobenzyl groups. Thus, DSM showed a highly selective response to ClO− among various ROS/RNS, relative to other R1SR2 (Figure 6A). Upon the gradual addition of ClO− (0–65 µM) to DSM (5 µM) in PBS buffer with a pH value of 7.4 (0.5% DMF), the absorption band at 300–375 nm decreased, and an absorption peak appeared at about 325 nm (Figure 7A). Furthermore, the fluorescence intensity of DSM (5 µM) at ∼445 nm was significantly increased in the presence of ClO− (50–65 µM) (Figure 7A), which is attributed to the appearance of DIMvia desulfurization (Figure 6B). Thiourea, a basic form of N2C=S, occurs in two tautomeric isomers, a thione form and a thiol form. The thiol form of DSM can react with reactive ClO−, leading to the breakage of the C–S bond and the formation of DIM (Figure 6B). The reaction of this fused imidazolium salt was referred to in several previous reports (Xu et al., 2015; Xu et al., 2016). The conversion of DSM to imidazolium salt was examined via the reaction of DSM and ClO− under similar conditions. The obtained main product (DSM′) was confirmed with DIM by 1H NMR, 13C NMR, and ESI-HRMS (Supplementary Figures S35, S36B). Furthermore, DSM was highly sensitive to ClO−, with a detection limit (LOD) of 0.13 µM (Supplementary Figure S50B), which is lower than that of many ClO− probes in previous reports (Xiao et al., 2015; Shen et al., 2017; Wang et al., 2018; Lee S. C. et al., 2020; Nguyen et al., 2020).
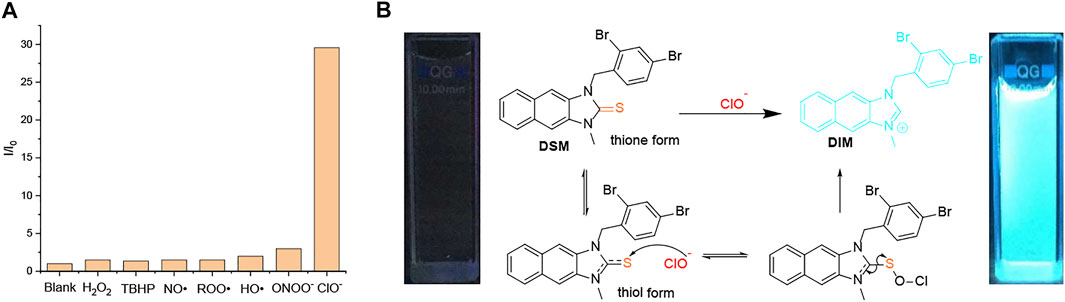
FIGURE 6. (A) Fluorescence intensity ratio (I/I0) of DSM (5 µM) in PBS buffer with a pH value of 7.4 (0.5% DMF) in the presence of ClO− (50 µM), ROO• (1 mM), NO• (1 mM), H2O2 (1 mM), TBHP (1 mM), ONOO− (200 µM), and •OH (200 µM). (B) Proposed desulfurization mechanism of DSMvia hypochlorite (ClO−).
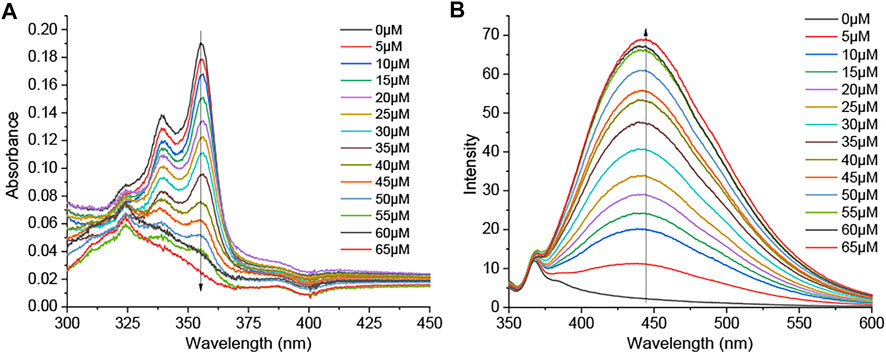
FIGURE 7. (A) UV–vis absorption and (B) fluorescence emission spectra (λex = 325 nm; slit 5/5) of DSM (5 µM) upon treatment with ClO− (0–65 µM) in PBS with a pH value of 7.4 (0.05% DMF).
Owing to the high fluorescence emission quantum yield and antibacterial activity of DIM, DSM can act as a potential OFF-ON fluorescent and antibacterial probe in the presence of ClO−. Consequently, it was further studied in an antibacterial test. ESBL-EC and MRSA were treated with DSM (0–16 µM) and/or ClO− (0–140 µM). The CFU percentage was measured after 18 h of incubation. The growth of bacteria was slightly inhibited in the presence of either ClO− (0–140 µM) or DSM (0–16 µM) (Figure 8). In contrast, their CFU percentages decreased under simultaneous treatment with ClO− and DSM. At ClO− concentrations of 20 and 80 μM, the lowest CFU rates were achieved at DSM concentrations of 2 and 8 μM, respectively. At a higher concentration of ClO− (140 µM), the lowest CFU rates were observed to be 59.6 and 55.9% at 16 µM of DSM toward ESBL-EC and MRSA bacteria, respectively. This demonstrates that DSM is converted to DIM upon ClO− treatment and subsequently inhibits bacterial growth. Therefore, DSM can be applied as a potential ClO−-activated fluorophore for enhanced fluorescence emission and antibacterial activity. This finding is unprecedented with regard to previous reports of ClO− fluorescent probes (Jiao et al., 2018; Pham et al., 2021b; Kwon et al., 2021).
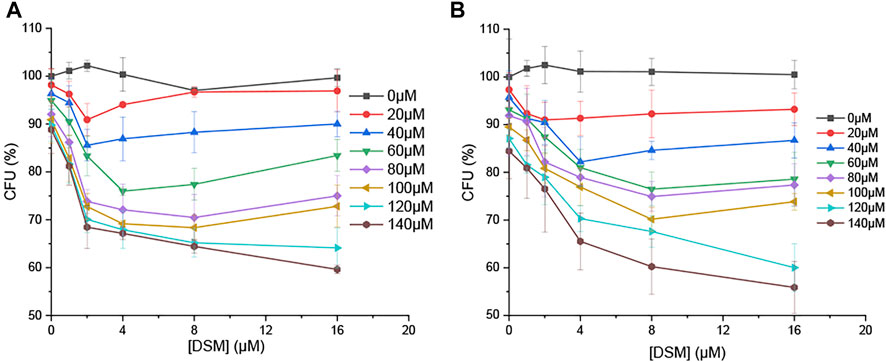
FIGURE 8. CFU percentage (%) of bacteria in the presence of DSM (0, 1, 2, 4, 8, and 16 µM) and ClO− (0, 20, 40, 60, 80, 100, 120, and 140 µM) toward (A) ESBL-EC and (B) MRSA.
Conclusion
A series of imidazolium salts (R1IR2) and imidazoline-2-thiones (R1SR2) (R1, R2 = methyl, dibromobenzyl, and carbazole groups) were synthesized and characterized by 1HNMR, 13CNMR, mass spectra, X-ray crystal structures, and DFT calculation–based molecular orbital analysis. The excitation wavelengths of the molecules were theoretically examined via TD-DFT with various functions and basis sets. Imidazolium salts (R1IR2) without a carbazole moiety showed high fluorescence emission, whereas imidazoline-2-thiones (R1SR2) exhibited weak emission. The antibacterial activities of these compounds against E. coli, S. aureus, ESBL-EC, EC-GFP, and MRSA were studied. Among these structures, DSM/DIM and DID/DSD showed high antibacterial activity ratios that would be useful for the design of OFF-ON antibacterial probes. However, DSD rarely reacted with ClO− due to steric hindrance, whereas DIM showed a high fluorescence emission quantum yield (ՓF = 62.9%) that would be useful for bacterial imaging, and DSM exhibited a highly selective ClO− response with an LOD of 0.13 µM. Finally, the DSM probe was converted to DIM upon ClO− treatment and inhibited bacterial growth.
Data Availability Statement
The original contributions presented in the study are included in the article/Supplementary Material; further inquiries can be directed to the corresponding authors.
Author Contributions
TP conceived and designed the probes and calculated the DFT in the Gaussian 09 package, V-NN designed the probes, YC synthesized the probes, DK and O-SJ calculated the X-ray crystal structures, DL and HK performed the antibacterial test, ML performed the computational study, and JY, HK, and SL designed the probes and wrote the manuscript.
Funding
This research was supported by the Basic Science Research Program through the National Research Foundation of Korea (NRF), funded by the Ministry of Education (no. 2017R1A6A3A04004954 for SL).
Conflict of Interest
The authors declare that the research was conducted in the absence of any commercial or financial relationships that could be construed as a potential conflict of interest.
The handling Editor declared a past coauthorship with one of the authors, JY.
Supplementary Material
The Supplementary Material for this article can be found online at: https://www.frontiersin.org/articles/10.3389/fchem.2021.713078/full#supplementary-material
References
Adamo, C., and Jacquemin, D. (2013). The Calculations of Excited-State Properties with Time-dependent Density Functional Theory. Chem. Soc. Rev. 42, 845–856. doi:10.1039/c2cs35394f
Allen, F. H., Kennard, O., Watson, D. G., Brammer, L., Orpen, A. G., and Taylor, R. (1987). Tables of Bond Lengths Determined by X-ray and Neutron Diffraction. Part 1. Bond Lengths in Organic Compounds. J. Chem. Soc. 2, S1–S19. doi:10.1039/p298700000s1
Bashir, M., Bano, A., Ijaz, A., and Chaudhary, B. (2015). Recent Developments and Biological Activities of N-Substituted Carbazole Derivatives: a Review. Molecules 20, 13496–13517. doi:10.3390/molecules200813496
Çoban, E. P., Fırıncı, R., Biyik, H., and Günay, M. E. (2017). Unsymmetrically Substituted Imidazolium Salts: Synthesis, Characterization and Antimicrobial Activity. Braz. J. Pharm. Sci. 53. doi:10.1590/s2175-97902017000115075
Domigan, N. M., Charlton, T. S., Duncan, M. W., Winterbourn, C. C., and Kettle, A. J. (1995). Chlorination of Tyrosyl Residues in Peptides by Myeloperoxidase and Human Neutrophils. J. Biol. Chem. 270, 16542–16548. doi:10.1074/jbc.270.28.16542
Duman, A. N., Ozturk, I., Tunçel, A., Ocakoglu, K., Colak, S. G., Hoşgör-Limoncu, M., et al. (2019). Synthesis of New Water-Soluble Ionic Liquids and Their Antibacterial Profile against Gram-Positive and Gram-Negative Bacteria. Heliyon 5, e02607. doi:10.1016/j.heliyon.2019.e02607
Gottardi, W., Klotz, S., and Nagl, M. (2014). Superior Bactericidal Activity of N -bromine Compounds Compared to Their N -chlorine Analogues Can Be Reversed under Protein Load. J. Appl. Microbiol. 116, 1427–1437. doi:10.1111/jam.12474
Gupta, A., Mumtaz, S., Li, C.-H., Hussain, I., and Rotello, V. M. (2019). Combatting Antibiotic-Resistant Bacteria Using Nanomaterials. Chem. Soc. Rev. 48, 415–427. doi:10.1039/c7cs00748e
Hryniewicka, A., Niemirowicz-Laskowska, K., Wielgat, P., Car, H., Hauschild, T., and Morzycki, J. W. (2021). Dehydroepiandrosterone Derived Imidazolium Salts and Their Antimicrobial Efficacy. Bioorg. Chem. 108, 104550. doi:10.1016/j.bioorg.2020.104550
Huang, R. T. W., Peng, K. C., Shih, H. N., Lin, G. H., Chang, T. F., Hsu, S. J., et al. (2011). Antimicrobial Properties of Ethoxyether-Functionalized Imidazolium Salts. Soft Matter 7, 8392–8400. doi:10.1039/c1sm05759f
Jeitner, T. M., Xu, H., and Gibson, G. E. (2005). Inhibition of the Alpha-Ketoglutarate Dehydrogenase Complex by the Myeloperoxidase Products, Hypochlorous Acid and Mono-N-Chloramine. J. Neurochem. 92, 302–310. doi:10.1111/j.1471-4159.2004.02868.x
Jiao, X., Li, Y., Niu, J., Xie, X., Wang, X., and Tang, B. (2018). Small-molecule Fluorescent Probes for Imaging and Detection of Reactive Oxygen, Nitrogen, and Sulfur Species in Biological Systems. Anal. Chem. 90, 533–555. doi:10.1021/acs.analchem.7b04234
Kardas, P., Devine, S., Golembesky, A., and Roberts, C. (2005). A Systematic Review and Meta-Analysis of Misuse of Antibiotic Therapies in the Community. Int. J. Antimicrob. Agents 26, 106–113. doi:10.1016/j.ijantimicag.2005.04.017
Kim, H. N., Lee, E.-H., Xu, Z., Kim, H.-E., Lee, H.-S., Lee, J.-H., et al. (2012). A Pyrene-Imidazolium Derivative that Selectively Recognizes G-Quadruplex DNA. Biomaterials 33, 2282–2288. doi:10.1016/j.biomaterials.2011.11.073
Kim, T., Zhang, Q., Li, J., Zhang, L., and Jokerst, J. V. (2018). A Gold/silver Hybrid Nanoparticle for Treatment and Photoacoustic Imaging of Bacterial Infection. ACS Nano 12, 5615–5625. doi:10.1021/acsnano.8b01362
Krasowska, A., and Konat, G. W. (2004). Vulnerability of Brain Tissue to Inflammatory Oxidant, Hypochlorous Acid. Brain Res. 997, 176–184. doi:10.1016/j.brainres.2003.09.080
Kurapati, R., Kostarelos, K., Prato, M., and Bianco, A. (2016). Biomedical Uses for 2D Materials beyond Graphene: Current Advances and Challenges Ahead. Adv. Mater. 28, 6052–6074. doi:10.1002/adma.201506306
Kwon, N., Kim, D., Swamy, K. M. K., and Yoon, J. (2021). Metal-coordinated Fluorescent and Luminescent Probes for Reactive Oxygen Species (ROS) and Reactive Nitrogen Species (RNS). Coord. Chem. Rev. 427, 213581. doi:10.1016/j.ccr.2020.213581
Ledwon, P. (2019). Recent Advances of Donor-Acceptor Type Carbazole-Based Molecules for Light Emitting Applications. Org. Elect. 75, 105422. doi:10.1016/j.orgel.2019.105422
Lee, S., Cheng, H., Chi, M., Xu, Q., Chen, X., Eom, C.-Y., et al. (2016). Sensing and Antibacterial Activity of Imidazolium-Based Conjugated Polydiacetylenes. Biosens. Bioelectron. 77, 1016–1019. doi:10.1016/j.bios.2015.10.090
Lee, S. C., Park, S., So, H., Lee, G., Kim, K.-T., and Kim, C. (2020b). An Acridine-Based Fluorescent Sensor for Monitoring ClO− in Water Samples and Zebrafish. Sensors 20, 4764. doi:10.3390/s20174764
Lee, S., Pham, T. C., Bae, C., Choi, Y., Kim, Y. K., and Yoon, J. (2020a). Nano Theranostics Platforms that Utilize Proteins. Coord. Chem. Rev. 412, 213258. doi:10.1016/j.ccr.2020.213258
Li, X., Bai, H., Yang, Y., Yoon, J., Wang, S., and Zhang, X. (2019). Supramolecular Antibacterial Materials for Combatting Antibiotic Resistance. Adv. Mater. 31, 1805092. doi:10.1002/adma.201805092
Li, X., Lee, D., Huang, J.-D., and Yoon, J. (2018a). Phthalocyanine-Assembled Nanodots as Photosensitizers for Highly Efficient Type I Photoreactions in Photodynamic Therapy. Angew. Chem. Int. Ed. 57, 9885–9890. doi:10.1002/anie.201806551
Li, X., Lee, S., and Yoon, J. (2018b). Supramolecular Photosensitizers Rejuvenate Photodynamic Therapy. Chem. Soc. Rev. 47, 1174–1188. doi:10.1039/c7cs00594f
Liu, L.-X., Wang, X.-Q., Zhou, B., Yang, L.-J., Li, Y., Zhang, H.-B., et al. (2015). Synthesis and Antitumor Activity of Novel N-Substituted Carbazole Imidazolium Salt Derivatives. Scientific Rep. 5, 1–20. doi:10.1038/srep13101
Mullen, D., and Hellner, E. (1978). A Simple Refinement of Density Distributions of Bonding Electrons. IX. Bond Electron Density Distribution in Thiourea, CS(NH2)2, at 123K. Acta Crystallogr. Sect B 34, 2789–2794. doi:10.1107/s0567740878009243
Nguyen, V.-N., Heo, S., Kim, S., Swamy, K. M. K., Ha, J., Park, S., et al. (2020). A Thiocoumarin-Based Turn-On Fluorescent Probe for Hypochlorite Detection and its Application to Live-Cell Imaging. Sensors Actuators B: Chem. 317, 128213. doi:10.1016/j.snb.2020.128213
Pak, Y. L., Park, S. J., Wu, D., Cheon, B., Kim, H. M., Bouffard, J., et al. (2018). N-heterocyclic Carbene Boranes as Reactive Oxygen Species-Responsive Materials: Application to the Two-Photon Imaging of Hypochlorous Acid in Living Cells and Tissues. Angew. Chem. 130, 1583–1587. doi:10.1002/ange.201711188
Pene, F., Courtine, E., Cariou, A., and Mira, J.-P. (2009). Toward Theragnostics. Crit. Care Med. 37, S50–S58. doi:10.1097/ccm.0b013e3181921349
Pham, T. C., Choi, Y., Bae, C., Tran, C. S., Kim, D., Jung, O.-S., et al. (2021a). A Molecular Design towards Sulfonyl Aza-BODIPY Based NIR Fluorescent and Colorimetric Probe for Selective Cysteine Detection. RSC Adv. 11, 10154–10158. doi:10.1039/d0ra10567h
Pham, T. C., Heo, S., Nguyen, V. N., Lee, M. W., Yoon, J., and Lee, S. (2021b). Molecular Design toward Heavy-atom-free Photosensitizers Based on the C═ S Bond and Their Dual Functions in Hypoxia Photodynamic Cancer Therapy and ClO–Detection. ACS Appl. Mater. Inter. 13 (12), 13949–13957. doi:10.1021/acsami.0c22174
Pham, T. C., Lee, S., Kim, D., Jung, O.-S., Lee, M. W., and Lee, S. (2020). Visual Simultaneous Detection and Real-Time Monitoring of Cadmium Ions Based on Conjugated Polydiacetylenes. ACS Omega 5, 31254–31261. doi:10.1021/acsomega.0c04636
Popescu, R., Filimon, M. N., Vlad, D. C., Verdes, D., Moatar, A., Moise, G., et al. (2021). Antiproliferative and Antibacterial Potential of Tetrahexylammonium Bromide-Based Ionic Liquids. Exp. Ther. Med. 22, 1–9. doi:10.3892/etm.2021.10104
Rehmat, N., Toffoletti, A., Mahmood, Z., Zhang, X., Zhao, J., and Barbon, A. (2020). Carbazole-perylenebisimide Electron Donor/acceptor Dyads Showing Efficient Spin Orbit Charge Transfer Intersystem Crossing (SOCT-ISC) and Photo-Driven Intermolecular Electron Transfer. J. Mater. Chem. C 8, 4701–4712. doi:10.1039/c9tc06429j
Reinhardt, A., Horn, M., Schmauck, J. P. g., Bröhl, A., Giernoth, R., Oelkrug, C., et al. (2014). Novel Imidazolium Salt-Peptide Conjugates and Their Antimicrobial Activity. Bioconjug. Chem. 25, 2166–2174. doi:10.1021/bc500510c
Riduan, S. N., and Zhang, Y. (2013). Imidazolium Salts and Their Polymeric Materials for Biological Applications. Chem. Soc. Rev. 42, 9055–9070. doi:10.1039/c3cs60169b
Salih, N., Salimon, J., and Yousif, E. (2016). Synthesis and Antimicrobial Activities of 9H-Carbazole Derivatives. Arabian J. Chem. 9, S781–S786. doi:10.1016/j.arabjc.2011.08.013
Saritha, R., Annes, S. B., Saravanan, S., and Ramesh, S. (2020). Carbazole Based Electron Donor Acceptor (EDA) Catalysis for the Synthesis of Biaryl and Aryl-Heteroaryl Compounds. Org. Biomol. Chem. 18, 2510–2515. doi:10.1039/d0ob00282h
Shen, B.-X., Qian, Y., Qi, Z.-Q., Lu, C.-G., Sun, Q., Xia, X., et al. (2017). Near-infrared BODIPY-Based Two-Photon ClO− Probe Based on Thiosemicarbazide Desulfurization Reaction: Naked-Eye Detection and Mitochondrial Imaging. J. Mater. Chem. B 5, 5854–5861. doi:10.1039/c7tb01344b
Sun, W., Li, M., Fan, J., and Peng, X. (2019). Activity-based Sensing and Theranostic Probes Based on Photoinduced Electron Transfer. Acc. Chem. Res. 52, 2818–2831. doi:10.1021/acs.accounts.9b00340
Valls, A., Andreu, J. J., Falomir, E., Luis, S. V., Atrián-Blasco, E., Mitchell, S. G., et al. (2020). Imidazole and Imidazolium Antibacterial Drugs Derived from Amino Acids. Pharmaceuticals 13, 482. doi:10.3390/ph13120482
Wang, X., Min, J., Wang, W., Wang, Y., Yin, G., and Wang, R. (2018). A Novel Porphyrin-Based Near-Infrared Fluorescent Probe for Hypochlorite Detection and its Application In Vitro and In Vivo. Analyst 143, 2641–2647. doi:10.1039/c8an00586a
Winterbourn, C. C., Vissers, M. C., and Kettle, A. J. (2000). Myeloperoxidase. Curr. Opin. Hematol. 7, 53–58. doi:10.1097/00062752-200001000-00010
Wu, D., Chen, L., Xu, Q., Chen, X., and Yoon, J. (2019). Design Principles, Sensing Mechanisms, and Applications of Highly Specific Fluorescent Probes for HOCl/OCl-. Acc. Chem. Res. 52, 2158–2168. doi:10.1021/acs.accounts.9b00307
Xiao, H., Li, J., Zhao, J., Yin, G., Quan, Y., Wang, J., et al. (2015). A Colorimetric and Ratiometric Fluorescent Probe for ClO−targeting in Mitochondria and its Application In Vivo. J. Mater. Chem. B 3, 1633–1638. doi:10.1039/c4tb02003k
Xu, Q., Heo, C. H., Kim, G., Lee, H. W., Kim, H. M., and Yoon, J. (2015). Development of Imidazoline-2-Thiones Based Two-Photon Fluorescence Probes for Imaging Hypochlorite Generation in a Co-culture System. Angew. Chem. Int. Ed. 54, 4890–4894. doi:10.1002/anie.201500537
Xu, Q., Heo, C. H., Kim, J. A., Lee, H. S., Hu, Y., Kim, D., et al. (2016). A Selective Imidazoline-2-Thione-Bearing Two-Photon Fluorescent Probe for Hypochlorous Acid in Mitochondria. Anal. Chem. 88, 6615–6620. doi:10.1021/acs.analchem.6b01738
Xu, Q., Lee, K.-A., Lee, S., Lee, K. M., Lee, W.-J., and Yoon, J. (2013). A Highly Specific Fluorescent Probe for Hypochlorous Acid and its Application in Imaging Microbe-Induced HOCl Production. J. Am. Chem. Soc. 135, 9944–9949. doi:10.1021/ja404649m
Xu, Z., Kim, S. K., and Yoon, J. (2010). Revisit to Imidazolium Receptors for the Recognition of Anions: Highlighted Research during 2006-2009. Chem. Soc. Rev. 39, 1457–1466. doi:10.1039/b918937h
Keywords: fluorescent sensor, fluorogenic probe, hypochlorite sensor, antibacterial effect, probe–killer
Citation: Pham TC, Nguyen V-N, Choi Y, Kim D, Jung O-S, Lee DJ, Kim HJ, Lee MW, Yoon J, Kim HM and Lee S (2021) Hypochlorite-Activated Fluorescence Emission and Antibacterial Activities of Imidazole Derivatives for Biological Applications. Front. Chem. 9:713078. doi: 10.3389/fchem.2021.713078
Received: 21 May 2021; Accepted: 17 June 2021;
Published: 12 July 2021.
Edited by:
Dokyoung Kim, Kyung Hee University, South KoreaReviewed by:
Ki Tae Kim, Chungbuk National University, South KoreaDaniel Gryko, Polish Academy of Sciences, Poland
Copyright © 2021 Pham, Nguyen, Choi, Kim, Jung, Lee, Kim, Lee, Yoon, Kim and Lee. This is an open-access article distributed under the terms of the Creative Commons Attribution License (CC BY). The use, distribution or reproduction in other forums is permitted, provided the original author(s) and the copyright owner(s) are credited and that the original publication in this journal is cited, in accordance with accepted academic practice. No use, distribution or reproduction is permitted which does not comply with these terms.
*Correspondence: Hwan Myung Kim, a2ltaG1AYWpvdS5hYy5rcg==; Songyi Lee, c2xlZUBwa251LmFjLmty