- 1Center of Translational Medicine, Fifth School of Medicine/Suizhou Central Hospital, Hubei University of Medicine, Suizhou, China
- 2School of Biological and Health Systems Engineering, Arizona State University, Tempe, AZ, United States
- 3Department of Radiology, University of Texas Southwestern Medical Center at Dallas, Dallas, TX, United States
- 4Biomedical Research Institute, Hubei University of Medicine, Shiyan, China
For wide applications of the lacZ gene in cellular/molecular biology, small animal investigations, and clinical assessments, the improvement of noninvasive imaging approaches to precisely assay gene expression has garnered much attention. In this study, we investigate a novel molecular platform in which alizarin 2-O-β-d-galactopyranoside AZ-1 acts as a lacZ gene/β-gal responsive 1H-MRI probe to induce significant 1H-MRI contrast changes in relaxation times T1 and T2in situ as a concerted effect for the discovery of β-gal activity with the exposure of Fe3+. We also demonstrate the capability of this strategy for detecting β-gal activity with lacZ-transfected human MCF7 breast and PC3 prostate cancer cells by reaction-enhanced 1H-MRI T1 and T2 relaxation mapping.
Introduction
Due to various advantages such as stability, high turnover rate, and ease of conjugation, the lacZ gene-encoding β-galactosidase (β-gal) has been broadly used in cellular/molecular biology, small animal studies, clinical trials with assays of clonal insertion, transcriptional activation, and protein expression and interaction (Kruger et al., 1999; Haberkorn et al., 2005; Razgulin et al., 2011; Yang et al., 2019). Moreover, overexpressed β-gal has been identified as a vital enzyme biomarker related to cell senescence and cancer progression (Chatterjee et al., 1979; Alam et al., 1990; Dimri et al., 1995; Paradis et al., 2001; Pacheco-Rivera et al., 2016; Lozano-Torres et al., 2017; Sharma and Leblanc, 2017; Kim et al., 2018; Wang et al., 2019; Li et al., 2020b; Gao et al., 2020; Qiu et al., 2020). Thus, β-gal activity detection has been exploited with diverse techniques including colorimetric assays (James et al., 2000; Browne et al., 2010; Zeng et al., 2012; Yeung et al., 2013; Chen et al., 2016; Hu Q. et al., 2017), fluorescence (Tung et al., 2004; Urano et al., 2005; Josserand et al., 2007; Kamiya et al., 2007; Feng et al., 2009; Koide et al., 2009; Kamiya et al., 2011; Oushiki et al., 2012; Han et al., 2013; Sakabe et al., 2013; Lee et al., 2014; Asanuma et al., 2015; Peng et al., 2015; Zeng et al., 2015; Doura et al., 2016; Gu et al., 2016; Zhang C. et al., 2016; Zhang X. X. et al., 2016; Huang J. et al., 2017; Hu Q. et al., 2017; Jiang et al., 2017; Kim et al., 2017; Nakamura et al., 2017; Wei et al., 2017; Zhang et al., 2017; Tang et al., 2017; Chen et al., 2018; Ito et al., 2018; Liu et al., 2018; Yang et al., 2018; Chen et al., 2019; Fu et al., 2019; Gu et al., 2019; Jiang et al., 2019; Kong et al., 2019; Lee et al., 2019; Shi et al., 2019; Singh et al., 2019; Zhang et al., 2019a; Zhang X. et al., 2019; Zhao et al., 2019; Li et al., 2020a; Li Y. et al., 2020; Li Z. et al., 2020; Pang et al., 2020; Wu et al., 2020; Zhu et al., 2020), chemiluminescence (Wehrman et al., 2006; Liu and Mason, 2010; Broome et al., 2015; Green et al., 2017; Huang Y. et al., 2017; Wang et al., 2017; Gorai and Maitra, 2018; Hananya and Shabat, 2019; Lozano-Torres et al., 2019; Zhang et al., 2019b), positron emission tomography or single-photon emission computed tomography (Celen et al., 2008; Van Dort et al., 2008; Rempel et al., 2017), magnetic resonance imaging (MRI) (Louie et al., 2000; Chang et al., 2007; Hanaoka et al., 2008; Cui et al., 2010; Bengtsson et al., 2010; Arena et al., 2011; Yu et al., 2012a; Gulaka et al., 2013; Li et al., 2013; Heffern et al., 2014; Burke et al., 2015; Hingorani et al., 2015; Fernández-Cuervo et al., 2016; Hu J. et al., 2017; Li and Meade, 2019; Xu et al., 2019; Lilley et al., 2020), and 19F-MRS/MRI approaches (Yu et al., 2005; Kodibagkar et al., 2006; Yu et al., 2006; Yu and Mason, 2006; Liu et al., 2007; Yu et al., 2008a; Yu et al., 2008b; Mizukami et al., 2011; Yu et al., 2012b; Yu et al., 2013; Yu et al., 2017). In particular, 1H-MRI molecular imaging approaches for visualization of β-gal activity attract much more attention because 1H-MRI is noninvasive and capable of soft tissue delineation with a high lateral and depth resolution (Terreno et al., 2010; Haris et al., 2015; Wahsner et al., 2019).
β-Galactosidase prompts the hydrolysis of β-d-galactopyranoside by cleavage of its β-anomeric C-O linkage between β-d-galactopyranose and aglycone; the hydrolysis reactivity of β-d-galactopyranosides to β-gal is completely dependent upon the aglycone structure. However, the structure activity relationship of the aglycones in β-d-galactopyranosides vs. β-gal is not yet clear (Juers et al., 2012; Duo et al., 2017). Therefore, further exploration is still highly needed to discover effective β-gal substrates for functional molecular imaging probes. We believe that the traditional histopathological methods of assaying β-gal activity might be the fruitful resources for developing novel imaging agents for the assessment of lacZ gene expression. In reviewing the histopathological literature, we noticed that the well-established β-gal substrate alizarin 2-O-β-d-galactopyranoside AZ-1 (Figure 1) is readily hydrolyzed by β-gal to release aglycone alizarin, which chelates with ferric iron Fe3+ to form an intense dark violet Fe complex (James et al., 2000). By comparison of the structural characteristics of the Fe3+–alizarin complex with Fe3+-based 1H-MRI contrast agents (Davies et al., 1996; Richardson et al., 1999; Schwert et al., 2002; Schwert et al., 2005; Haas and Franz, 2009; Yu et al., 2012a; Yu et al., 2012b; Gulaka et al., 2013; Li et al., 2013; Yu et al., 2013; Kuznik and Wyskocka, 2016), we speculated that the Fe3+–alizarin complex could function as an Fe3+-based 1H-MRI contrast agent. If so, the well-established β-gal substrate AZ-1 could work as a lacZ gene or β-gal 1H-MRI reporter. Upon delivery and cleavage at lacZ-transfected or β-gal–overexpressed tumor cells with the presence of Fe3+, the paramagnetic Fe complex could be spontaneously formed in situ and specifically produced the 1H MRI contrast effect while localizing and accumulating 1H-MRI signals at the β-gal activity site. Figure 1 depicts the Fe3+–alizarin complex generated in situ for the 1H-MRI detection of β-gal activity. We now demonstrate the use of exploiting AZ-1 to assess β-gal activity in vitro with lacZ-transfected human MCF7 breast and PC3 prostate cancer cells by 1H MRI T1 and T2 relaxation mapping.
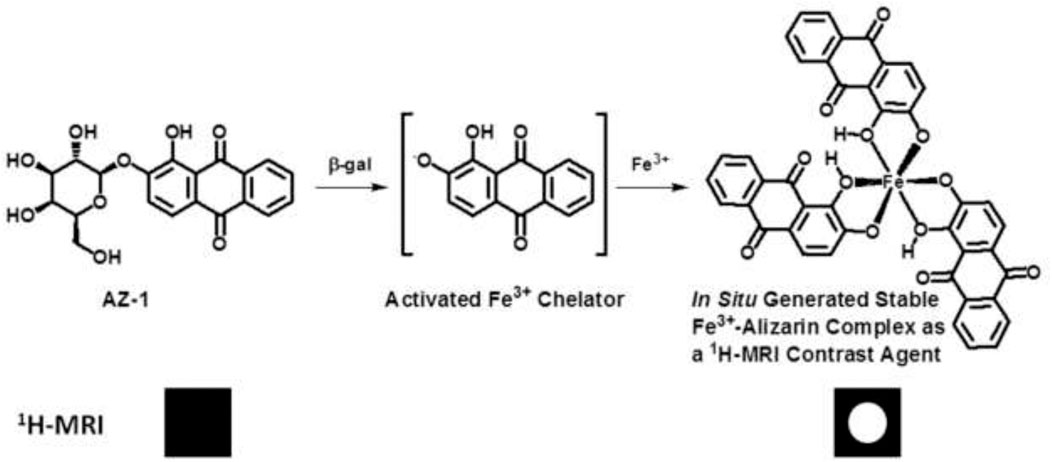
FIGURE 1. The proposed mechanism of an in situ generated stable Fe3+–alizarin complex for 1H MRI detection of β-gal activity.
Results and Discussion
Verification of the Fe3+–Alizarin Complex as an 1H-MRI Contrast Agent
Alizarin is 1,2-dihydroxy-9,10-anthraquinone with a tricyclic aromatic planar structure and chelates with Fe3+ to form a thermodynamically stable octahedral Fe3+–alizarin (1:3) complex at physiological pH conditions with the stability constant logβ = 32.21 (Das et al., 1995; Das et al., 2002). To explore the MRI signal–enhancing capability of the Fe3+–alizarin complex, the spin–lattice relaxation time T1 and spin–spin relaxation time T2 of the Fe3+–alizarin complex were measured with a 4.7 T MR scanner using a saturation recovery spin echo sequence and multi-spin echo sequence with varying repetition times (TRs) and echo times (TEs), respectively. The images were acquired using a 3-cm diameter solenoid coil (home-built) with 4 × 4 cut section of a 96-well plate containing the different concentrations of alizarin and ferric ammonium citrate (FAC) mixed solutions in 1:1 (V/V’) DMSO/PBS (0.1 M, pH = 7.4) at 37°C. Figure 2 displays the significant changes as expected on the T1 and T2 maps and relaxation time values of the Fe3+–alizarin complex at T1 = 254 ± 3, 131 ± 3, and 92 ± 8 ms, and T2 = 106 ± 1, 59 ± 1, and 48 ± 1 ms, corresponding to the concentrations of alizarin at 2.5, 6.0, and 9.0 mM, respectively. The comparison with the control FAC of T1 = 389 ± 6 ms and T2 = 143 ± 1 ms showed that the Fe3+–alizarin complex formed in situ resulted in substantial signal enhancement on either T1- or T2-weighted 1H-MRI, confirming the Fe3+–alizarin complex generated in situ to function as an 1H-MRI contrast agent. Notably, the significantly different T1 and T2 values of the Fe3+–alizarin complex suggested the potential to combine T1 and T2 data for additional information of imaging evaluation and detection reliability, specifically where there is possibility for misinterpretation in tissue heterogeneity (Zhou et al., 2017).
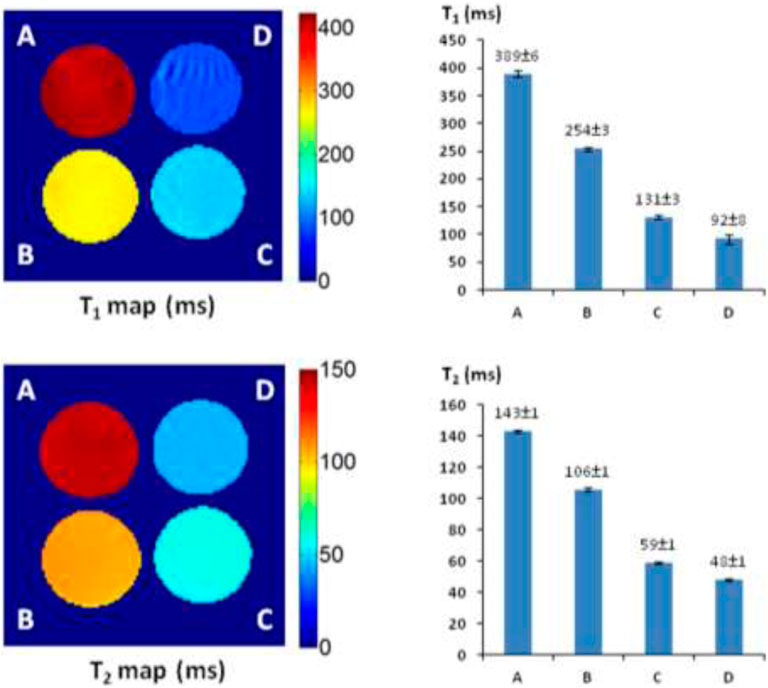
FIGURE 2. The 1H-MRI of an Fe3+–alizarin complex. MRI acquisition parameters: 1H-MRI, 200 MHz, matrix size: 128 × 128, FOV: 40 mm × 40 mm, slice thickness: 2 mm; receiver bandwidth: 20 kHz, T1-map: saturation recovery spin-echo sequence, TR = 200, 400, 600, 800, 1,000, 2000, 3,000, and 6,000 ms, respectively, TE = 15 ms; T2-map: multi-echo SE sequence, TE = 10, 20, 30, 40, 50, 60, 70, 80, 90, 100, 110, 120, 130, 140, 150, and 160 ms, respectively, TR = 2000 ms. (A) Control, FAC (15.0 mM); (B) alizarin (2.5 mM), FAC (15.0 mM); (C) alizarin (6.0 mM) and FAC (15.0 mM); (D) alizarin (9.0 mM), FAC (15.0 mM) in 1:1 (V/V′), and DMSO/PBS (0.1 M, pH = 7.4) at 37°C in 4 h.
Alizarin β-d-Galactopyranoside Synthesis
After the Fe3+–alizarin complex was shown to be an 1H-MRI contrast agent, we therefore began the β-d-galactopyranosylation with alizarin at the phenolic hydroxyl groups. Previously, James et al. (2000) reported a modified method for the synthesis of AZ-1, involving the reaction of alizarin potassium salt with acetobromo-α-D-galactose via the nucleophilic substitution procedure followed by deacetylation mediated by the aqueous NaOH solution, but the yield was low (14%). We observed that the phenolic hydroxyl groups at 1,2-positions of alizarin have excellent site-reaction selectivity due to the various electronic deficiency/sterically hindered effects (Mahal et al., 2011) and apparently different pKa values: pKa(2-OH) = 5.98 ± 0.05, whereas pKa(1-OH) = 9.88 ± 0.05 (Das et al., 1995; Das et al., 2002), which suggested that the phase-transfer catalysis method at pH = 8-9 could provide regio- and stereoselective synthesis of AZ-1, as exploited previously for β-gal 19F-MRS/MRI reporters (Yu et al., 2005; Kodibagkar et al., 2006; Yu et al., 2006; Yu and Mason, 2006; Liu et al., 2007; Yu et al., 2008a; Yu et al., 2008b; Yu et al., 2012b; Yu et al., 2013; Yu et al., 2017). To the well-stirred solution of alizarin in CH2Cl2-H2O (pH 8–9) using tetrabutylammonium bromide (TBAB) as a catalyst at 50°C, an equimolar amount of 2, 3, 4, and 6-tetra-O-acetyl-α-D-galactopyranosyl bromide was dropped under N2 atmosphere for around 1 h; alizarin 2-O-2′, 3′, 4′, and 6′-tetra-O-acetyl-β-D-galactopyranoside AZ-M1 was isolated purely at the yield of 78%. The NOESY correlation between anomeric H-1′ and H-3 in AZ-M1 (Supporting Information, Supplementary Figure S7) showed that β-d-galactopyranosylation took place at the 2-hydroxyl group of alizarin as predicted. The following deacetylation with NH3/MeOH from 0°C to room temperature produced AZ-1 at 91% yield.
The prerequisite for molecular MRI of intracellular targets is that the contrast agents must be effectively taken up by cells in vivo, which requires contrast agents to be sufficiently soluble and capable of entering cells. Carbohydrate-associated prodrugs in clinical applications have widely demonstrated the improved aqueous solubility and permeability, leading to better selectivity and efficacy for diagnosis and therapy (Dwek, 1996; Bertozzi and Kiessling, 2001; Hudak and Bertozzi, 2014; Fernández-Tejada et al., 2015). We hence thought about introducing an additional β-D-galactopyranosyl unit to form alizarin 1,2-di-O-β-d-galactopyranoside AZ-2. Similarly, a drop of 2.2 equivalent 2, 3, 4, and 6-tetra-O-acetyl-α-D-galactopyranosyl bromide CH2Cl2 solution into alizarin in CH2Cl2-H2O (pH 10–11) employing TBAB as a catalyst at 55°C under N2 atmosphere afforded alizarin 1,2-di-O-2′, 3′, 4′, 6′-tetra-O-acetyl-β-D-galactopyranoside AZ-M2 at 62% yield. Deacetylation accomplished the target molecule alizarin 1,2-di-O-β-d-galactopyranoside AZ-2 with 94% yield. Figure 3 illustrates the structures of AZ-1/AZ-M1 and AZ-2/AZ-M2. As expected, the free di-β-d-galactopyranoside AZ-2 is soluble in PBS (0.1 M, pH = 7.4) at the concentration of 15 mM; meanwhile, the free mono-β-d-galactopyranoside AZ-1 unlikely requires the addition of DMSO for the same concentration. The structures of AZ-M1/AZ-1 and AZ-M2/AZ-2 were confirmed by NMR and HRMS data. The molecular/quasimolecular ions of AZ-M1 and AZ-1, as well as AZ-M2 and AZ-2, showed the introduction of one and two galactopyranosyl units to AZ-M1/AZ-1 and AZ-M2/AZ-2, respectively, in which the β-D-galactopyranoside configuration was determined by 1H/13C NMR data of the anomeric protons at δH-1′ = δH-1′′ = 4.90–5.30 ppm and their coupling constants J1′,2′ = J1′′,2′′≅8.0 Hz while maintaining the corresponding anomeric C-1′/C-1′′ at δC-1′ = δC-1′′ = 99.5–104.0 ppm in accordance (Supporting Information, Supplementary Figures S4–S13), which are in agreement with the typical characteristics for the identification of the anomeric β-d-configuration (Yu et al., 2005; Yu et al., 2006; Yu and Mason, 2006; Kodibagkar et al., 2006; Liu et al., 2007; Yu et al., 2008a; Yu et al., 2008b; Yu et al., 2012b; Yu et al., 2013; Yu et al., 2017).
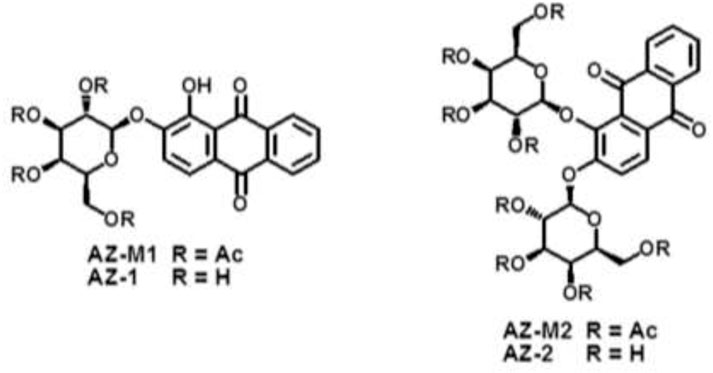
FIGURE 3. The structures of alizarin mono β-D-galactopyranosides AZ-M1/AZ-1, and alizarin di-β-D-galactopyranosides AZ-M2/AZ-2.
β-Gal Reactivity
AZ-1 has been identified as a highly sensitive substrate for the demonstration of β-gal in a range of Gram-negative bacteria under incubation at 37°C in air for 18 h (James et al., 2000). However, none of the existing data have shown the kinetics of AZ-1 vs. β-gal, which is crucial for further in vivo imaging applications. The absorption spectra of AZ-1 and AZ-2 solutions in 1:1 (V/V′) DMSO/PBS (0.1 M, pH = 7.4) with and without β-gal (E801A) at 20–22°C indicated that upon reactions of AZ-1 and AZ-2 with β-gal, a new absorption around 520 nm, corresponding to the in situ released alizarin mono-/dianions, appeared and increased gradually. Hence, the absorbance measurements at 520 nm following the enzymatic reaction of AZ-1 and AZ-2 with β-gal (E801A) at 20–22°C in different time points showed that both AZ-1 and AZ-2 are very reactive to β-gal (E801A) with varying hydrolytic rates at ν(AZ-1) = 93.3 and ν(AZ-2) = 133.3 μM/min/unit, respectively (Figure 4). Also, the absorption spectra of AZ-1 and AZ-2 by reaction with other enzymes α-galactosidase (Sigma G7163) and β-glucuronidase (Sigma G8295) at 20–22°C; showed that both AZ-1 and AZ-2 remained essentially stable over the period of 60 min, verifying their specificity for reaction to β-gal.
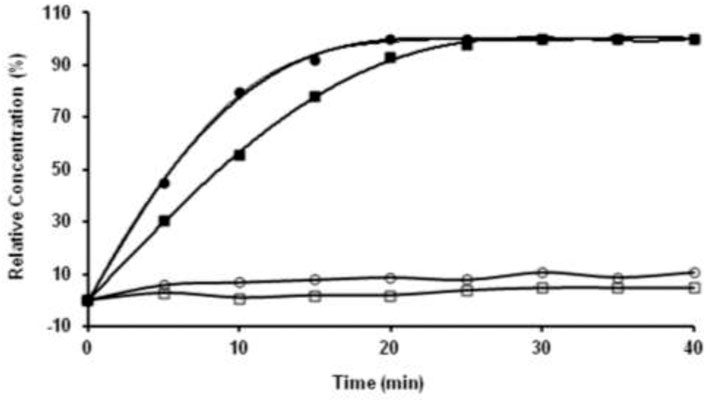
FIGURE 4. The kinetic hydrolysis time courses of alizarin β-D-galactopyranosides AZ-1 (■) and AZ-2 (●) with β-gal. Absorbance measurements at λ = 520 nm following the addition of β-gal (E801A, 3 units) to solutions of AZ-1, AZ-2 each (5.0 mM) in 1:1 (V/V′) DMSO/PBS (0.1 M, pH = 7.4) at 20–22°C in different time points; The time courses of alizarin β-D-galactopyranosides AZ-1 (□) and AZ-2 (○) each (5.0 mM) in 1:1 (V/V′) DMSO/PBS (0.1 M, pH = 7.4) at 20–22°C in different time points without β-gal (E801A).
1H-MRI Detection of β-Gal Activity
The T1 and T2 maps and relaxation time values were measured with a 4 × 4 cut section of 96-well plate containing various concentrations of AZ-1 and AZ-2 (4.0–9.0 mM) together with a fixed concentration of FAC (15.0 mM), respectively, in 1:1 (V/V′) DMSO/PBS (0.1 M, pH = 7.4) with or without β-gal (E801A). In the AZ-1/FAC solution at 37°C in 4 h in the absence of β-gal, relaxation times were determined to be T1 = 368 ± 6 and T2 = 134 ± 1 ms. In comparison, in the presence of β-gal (E801A, 5 units) in the mixture solution of AZ-1 and FAC at 37°C in 4 h, pronounced shortening in relaxation times T1 and T2 caused by the Fe3+–alizarin complex generated in situ was observed at T1 = 138 ± 3, 115 ± 4, and 84 ± 5 ms, whereas T2 = 74 ± 1, 54 ± 1, and 44 ± 5 ms, correlating with the increasing concentrations of AZ-1 from 4.0, 6.0 and 9.0 mM, respectively (Figure 5). However, the much more soluble and reactive AZ-2, exhibiting significant advantages for in vivo1H-MRI applications, produced very unexpected results under similar procedures at the same conditions. In the absence of β-gal at 37°C in 4 h, the AZ-2/FAC solution, as the control, yielded surprisingly reduced T1 = 230 ± 11 and T2 = 98 ± 1 ms (Figure 6). However, in the presence of β-gal (E801A, 5 units), the mixture solutions of AZ-2/FAC gave rise to an insignificant decrease in T1 = 220 ± 7, 198 ± 11, and 177 ± 5 ms and T2 = 95 ± 1, 78 ± 2, and 72 ± 1 ms (AZ-2 concentrations at 4.0, 6.0, and 9.0 mM, respectively, Figure 6), indicating the much less Fe3+–alizarin complex formed in situ during the β-gal hydrolysis. Comparing the interactions between AZ-1/AZ-2 and Fe3+ with their relaxivities to FAC, we attributed that the larger differences of AZ-2/FAC to FAC solution (T1: 230 ± 11 vs. 389 ± 6 ms and T2: 98 ± 1 vs. 143 ± 1 ms; alternatively, ΔR1 = 1.78 s−1 and ΔR2 = 3.21 s−1) than AZ-1/FAC solution (T1: 368 ± 6 vs. 389 ± 6 ms, T2: 134 ± 1 vs. 143 ± 1 ms; alternatively, ΔR1 = 0.15 s−1, ΔR2 = 0.47 s−1) were risen from the formation of the much stronger and more stable molecular tweezer complex AZ-2/Fe3+ due to the adjacent 3′,4′,6′-OH and 3″,4″,6″-OH located at the same side of 1,2-di-O-β-d-galactopyranosyl rings in the favored configuration for chelation of Fe3+ (Supplementary Figure S3 in the Supporting Information) (Davies et al., 1996; Richardson et al., 1999; Schwert et al., 2002; Schwert et al., 2005; Haas and Franz, 2009; Coskuner et al., 2011; Kuznik and Wyskocka, 2016) which thus simultaneously hindered the reaction with β-gal (E801A) and slowed down the release of alizarin as well as the in situ generation of the Fe3+–alizarin complex. These were confirmed by mixing solutions of AZ-2 and β-gal (E801A) first for hydrolysis, and then followed by adding FAC for complexation at 37°C in 2 h for each step with the same concentrations as in Figure 6. A significant decrease in relaxation times T1 and T2 was seen at T1 = 133 ± 1, 110 ± 2, and 78 ± 2 ms and T2 = 73 ± 2, 51 ± 3, and 41 ± 1 ms, which were very close to expectations based on AZ-1/FAC T1 and T2 data as shown in Figure 5.
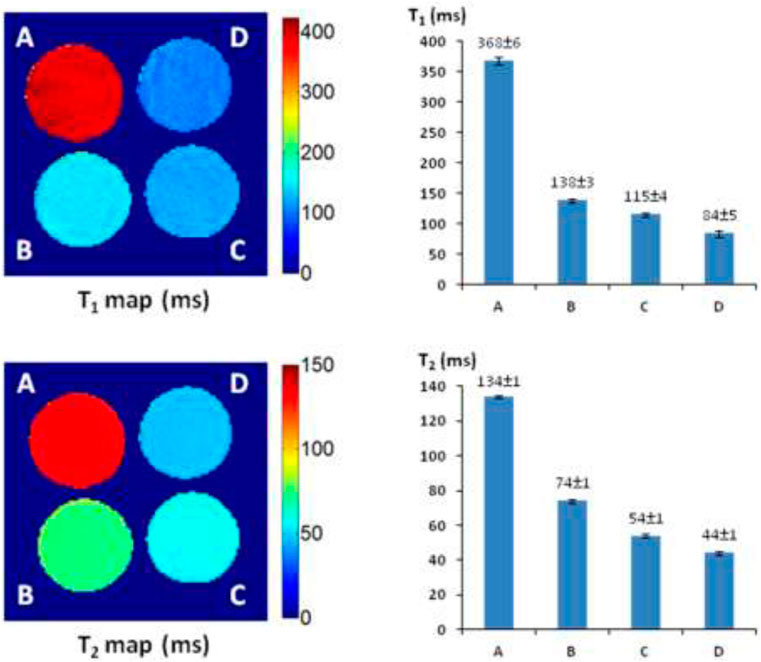
FIGURE 5. 1H-MRI detection of β-gal activity. 1H-MRI acquisition: using the same parameters as in Figure 2, (A) alizarin 2-O-β-D-galactopyranoside AZ-1 (9.0 mM) and FAC (15.0 mM); (B) alizarin 2-O-β-D-galactopyranoside AZ-1 (4.0 mM), FAC (15.0 mM), and β-gal (E801A, 5 units); (C) alizarin 2-O-β-D-galactopyranoside AZ-1 (6.0 mM), FAC (15.0 mM), and β-gal (E801A, 5 units); (D) alizarin 2-O-β-D-galactopyranoside AZ-1 (9.0 mM), FAC (15.0 mM), and β-gal (E801A, 5 units) in 1:1 (V/V′) DMSO/PBS (0.1 M, pH = 7.4) at 37°C in 4 h.
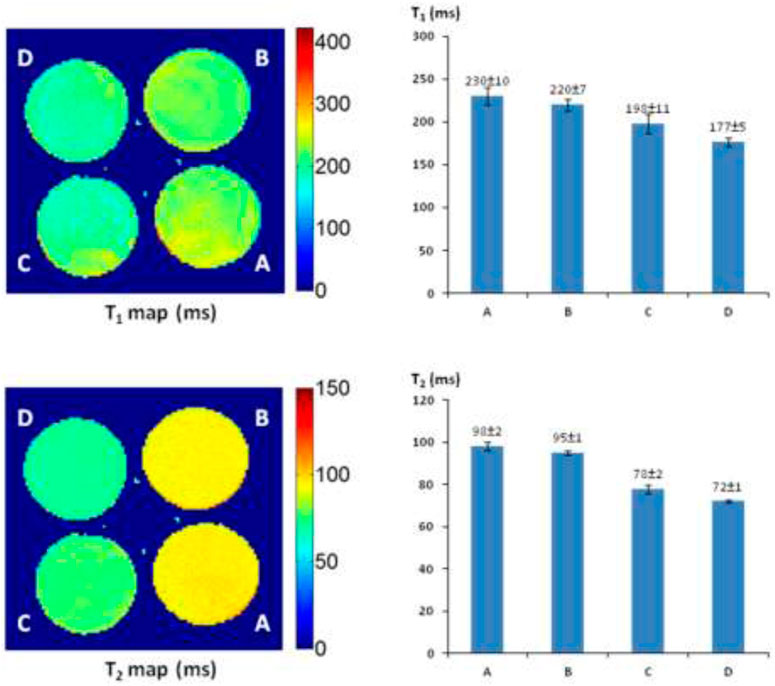
FIGURE 6. 1H-MRI detection of β-gal activity. 1H-MRI acquisition: using the same parameters as in Figure 2, (A) alizarin 1,2-di-O-β-D-galactopyranoside AZ-2 (9.0 mM) and FAC (15.0 mM); (B) alizarin 1,2-di-O-β-D-galactopyranoside AZ-2 (4.0 mM), FAC (15.0 mM), and β-gal (E801A, 5 units); (C) alizarin 1,2-di-O-β-D-galactopyranoside AZ-2 (6.0 mM), FAC (15.0 mM), and β-gal (E801A, 5 units); (D) alizarin 1,2-di-O-β-D-galactopyranoside AZ-2 (9.0 mM), FAC (15.0 mM), and β-gal (E801A, 5 units) in 1:1 (V/V′) DMSO/PBS (0.1 M, pH = 7.4) at 37°C in 4 h.
In Vitro1H-MRI Detection of lacZ Transfection in Human Tumor Cells
The recombinant vector phCMVlacZ has been successfully created and used to stably transfect human prostate cancer PC3-lacZ cells from PC3-wild-type (WT) cells (Liu et al., 2007). Accordingly, human breast cancer MCF7-lacZ cells were stably transfected from MCF7 wild-type (WT) cells: the β-gal activity and quantification in MCF7-lacZ cells were verified on the basis of Western blot, X-gal and S-gal staining, and the β-gal assay (Figure 7).
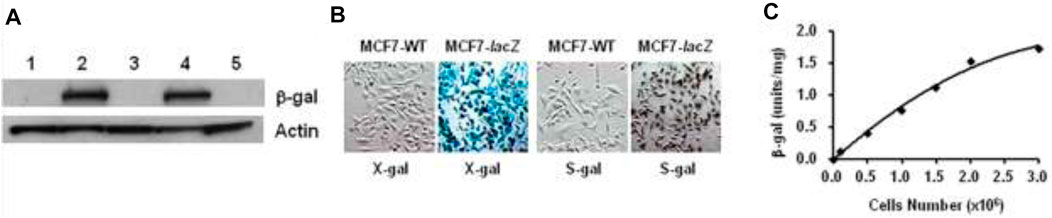
FIGURE 7. MCF7-lacZ transfection. (A) Western blot: protein extracts from two transfected MCF7-lacZ cell lines (lanes 2,4), together with MCF7-WT cells (lanes 1,3,5) showing intense bands for β-gal activity in MCF7-lacZ cells and none in MCF7-WT cells; (B) MCF7-WT/MCF7-lacZ cells staining by X-gal and S-gal: deep blue (X-gal) and black (S-gal) staining confirming the intense lacZ expression in MCF7-lacZ cells with essentially no β-gal activity in MCF7-WT cells. Regional magnification ×100; (C) β-Gal assay for activity quantification in MCF7-lacZ cells: 1.0 unit corresponding to the hydrolysis of 1.0 μmol/min O-nitrophenyl β-D-galactopyranoside, β-gal activity was increasingly associated with the number of MCF7-lacZ cells.
Given AZ-2 showed much better aqueous solubility and reactivity to β-gal, the stabilized molecular tweezer complexation AZ-2/Fe3+ obstructed its implementation spreading to effective 1H-MRI assessment of β-gal. So, AZ-1 with a significant signal loss either on T1 or T2 upon β-gal hydrolysis was prompted for the further in vitro1H-MRI evaluation. As an initial demonstration for in vitro1H-MRI detection of β-gal with lacZ-transfected human cancer cells, we first acquired T2* maps on pair mixtures of AZ-1 (10.0 mM) with PC3-WT cells (5 × 105) and PC3-lacZ cells (5 × 105), respectively, in the presence of FAC (10.0 mM) layered between agarose after incubation 4 h at 37°C under 5% CO2/air with 95% humidity. Significant differences confined within the layers were observed between PC3-WT and PC3-lacZ cells at different echo times (Figure 8A), in which there was essentially no signal loss with PC3-WT cells but a remarkable signal decrease with PC3-lacZ cells upon increasing echo times (TEs) (Figure 8B). The relaxation time T2* was determined to be T2*(AZ-1/PC3/FAC) = 96 ± 23 ms in PC3-WT cells, while T2*(AZ-1/PC3-lacZ/FAC) = 26 ± 14 ms in PC3-lacZ cells. Again, the β-gal activity was verified based on X-gal, S-gal, and AZ-1 staining (dark violet) (Figure 8C), with each staining method consistently showing intense lacZ expression in PC3-lacZ cells with essentially no β-gal activity in PC3-WT cells.
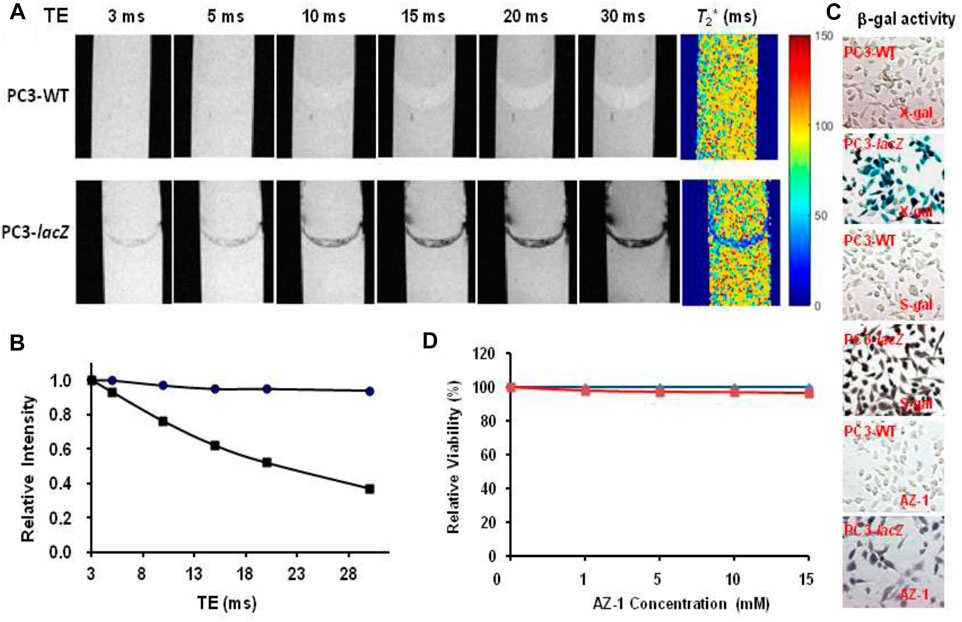
FIGURE 8. In vitro1H-MRI detection of lacZ gene expression in PC3-lacZ cells. 1H-MRI acquisition: 1H-MRI, 400 MHz, matrix size: 256 × 128, FOV: 48 mm × 24 mm, gradient echo imaging: TE = multiple values 3–30 ms, TR = 100 ms, flip angle = 10° (A)T2* maps: A mixture of alizarin 2-O-β-D-galactopyranosides AZ-1 (10.0 mM) and FAC (10.0 mM) with 5 × 105 PC3-WT or PC3-lacZ cells was placed in the interlayer between 1% low-gelling temperature agarose in a 10-mm NMR tube, and then incubated for 4 h at 37°C under 5% CO2/air with 95% humidity, T2*(AZ-1/PC3-WT/FAC) = 96 ± 23 ms (top row) and T2*(AZ-1/PC3-lacZ/FAC) = 26 ± 14 ms (bottom row), respectively; (B) relative signal intensity changes at different echo times (TEs) from (A), essentially no signal loss with PC3-WT cells (●) but a significant signal loss with PC3-lacZ cells (■); (C) PC3-WT/PC3-lacZ cells staining by X-gal, S-gal, and AZ-1: deep blue (X-gal), black (S-gal), and dark violet (AZ-1) staining confirming an intense lacZ expression in PC3-lacZ cells with essentially no β-gal activity in PC3-WT cells. Regional magnification ×100; (D) cytotoxicity: PC3-WT/PC3-lacZ cells viability vs.AZ-1 in various concentrations in 1:1 (V/V′) DMSO/PBS (0.1 M, pH = 7.4) at 37°C under 5% CO2/air with 95% humidity for 72 h, PC3-WT cells (in blue), and PC3-lacZ cells (in red)).
The cytotoxicity of AZ-1 was studied with PC3-WT and PC3-lacZ cells in PBS (0.1 M, pH = 7.4) incubated 72 h at 37°C under 5% CO2/air with 95% humidity. Cell viability assays showed that neither AZ-1 nor alizarin showed toxicity to PC3 cells, for AZ-1 viability exceeded 96% for both PC3-WT and PC3-lacZ cells at all concentrations tested (Figure 8D).
Furthermore, in vitro1H-MRI acquisition of AZ-1 (10.0 mM) with PC3-WT cells (5 × 105) and PC3-lacZ cells (5 × 105) in the presence of FAC (10.0 mM) was performed in a 1:1 (V/V′) DMSO/PBS (0.1 M, pH = 7.4) solution. A pronounced signal decrease in the relaxation time T1 was observed between PC3-WT (T1(AZ-1/PC3-WT/FAC) = 245 ± 9 ms) and PC3-lacZ cells (T1(AZ-1/PC3-lacZ/FAC) = 82 ± 7 ms) after incubation 4 h at 37°C under 5% CO2/air with 95% humidity.
Similarly, after incubation of AZ-1 (10.0 mM) with MC7-WT cells (5 × 105) and MC7-lacZ cells (5 × 105), respectively, in the same conditions as the previous study, the relaxation times were observed to be T1(AZ-1/MCF7-WT/FAC) = 223 ± 11 ms and T2(AZ-1/MCF7-WT/FAC) = 97 ± 12 ms in MC7-WT cells, whereas T1(AZ-1/MCF7-lacZ/FAC) = 75 ± 7 ms and T2(AZ-1/MCF7-lacZ/FAC) = 45 ± 9 ms made for MC7-lacZ cells (Figures 9A,B), the T1 and T2 values are shown as bars adjacent to T1 and T2 maps; both illustrated significant differences after the reaction with β-gal at ΔR1 = 8.85 s−1 and ΔR2 = 11.91 s−1. Staining by X-gal, S-gal, and AZ-1 (dark violet) (Figure 9C) all displayed an intense lacZ expression in MC7-lacZ cells but with essentially no β-gal activity in MC7-WT cells. Cell viability assays indicated that both AZ-1 and the released aglycone alizarin have no toxicity to MC7 cells upon the viability above 95% to MC7-WT and MC7-lacZ cells at all concentrations tested for 72 h (Figure 9D).
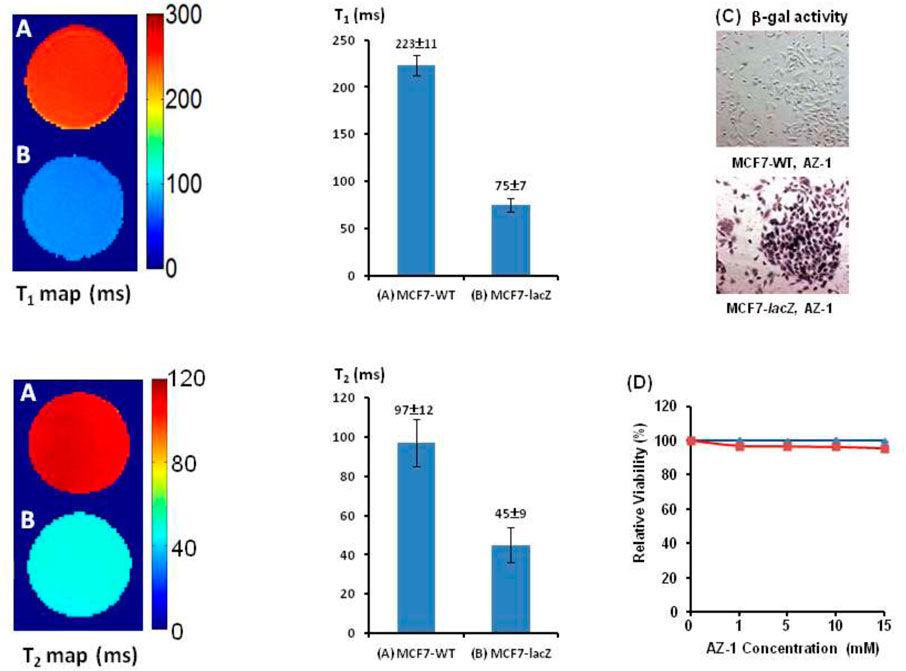
FIGURE 9. In vitro1H-MRI detection of the lacZ gene expression in MCF7-lacZ cells. 1H-MRI acquisition: using the same parameters as in Figure 2. T1 and T2 Maps: Solution of alizarin 2-O-β-D-galactopyranoside AZ-1 (10.0 mM) and FAC (10.0 mM) in 1:1 (V/V′) DMSO/PBS (0.1 M, pH = 7.4) after incubation 4 h at 37°C under 5% CO2/air with 95% humidity with (A) MCF7-WT cells [5 × 105, T1(AZ-1/MCF7-WT/FAC) = 223 ± 11 ms, T2(AZ-1/MCF7-WT/FAC) = 97 ± 12 ms]; (B) MCF7-lacZ cells [5 × 105, T1(AZ-1/MCF7-lacZ/FAC) = 75 ± 7 ms, and T2(AZ-1/MCF7-lacZ/FAC) = 45 ± 9 ms]; (C) MCF7-WT/MCF7-lacZ cells staining by AZ-1: dark violet staining confirming intense lacZ expression in MCF7-lacZ cells with essentially no β-gal activity in MCF7-WT cells. Regional magnification ×100; (D) Cytotoxicity: MCF7-WT/MCF7-lacZ cells viability vs.AZ-1 in various concentrations in 1:1 (V/V′) DMSO/PBS (0.1 M, pH = 7.4) at 37°C under 5% CO2/air with 95% humidity for 72 h, MCF7-WT cells (in blue), and MCF7-lacZ cells (in red).
Currently, a Gd-based contrast agent–enhanced 1H-MRI has been widely applied for medical diagnosis, offering a noninvasive way to generate anatomical and physiological information while maintaining high spatial and temporal resolution (Terreno et al., 2010; Haris et al., 2015; Wahsner et al., 2019). An Fe-based 1H MRI contrast agent, different from the Gd3+-based 1H MRI contrast agent with very strong relaxivity, exhibited much shorter relaxation times because of the formation of Fe complexes with the complete coordination of Fe3+, eliminating the possibility of inner-sphere to directly coordinate water, leaving outer-sphere and second-sphere coordination water molecules as the only pathways for relaxation (Davies et al., 1996; Richardson et al., 1999; Schwert et al., 2002; Schwert et al., 2005; Haas and Franz, 2009; Kuznik and Wyskocka, 2016). However, an Fe-based contrast agent enhanced 1H-MRI has now become a viable alternative because Fe3+ is extensively present in the tissues of the human body and is involved in transport, storage, compartmentalization, and excretion mechanisms, while Gd3+ is not naturally present in human biochemistry (Beutler, 2004; Weber et al., 2006; Kaplan and Kaplan, 2009; Theil and Goss, 2009). Particularly, cancer cells need a significant amount of Fe3+ for rapid replication, so endogenously abundant Fe3+ in tumors has been recognized as a molecular target for chemotherapeutic treatments through depleting cancer cellular Fe3+ to disrupt cancer cell proliferation and inhibit tumor growth (Fe3+-chelation therapy) (Buss et al., 2003; Richardson, 2005). In this study, we introduced exogenous Fe3+ with the ultimate goal of developing this approach to hunt the elevated Fe3+ level in tumors for the 1H-MRI signal generation. Indeed, alizarin has a very high thermodynamic stability constant logβ = 32.21 (Das et al., 1995; Das et al., 2002), indicating its capability of capturing Fe3+ from tumor to produce the Fe3+–alizarin complex in situ while simultaneously generating the 1H-MRI signal enhancement (Richardson et al., 1999; Davies et al., 1996; Schwert et al., 2002; Schwert et al., 2005; Haas and Franz, 2009; Kuznik and Wyskocka, 2016). Moreover, alizarin has been known to inhibit human cytomegalovirus replication, HIV-1 RT-associated RDDP, and integrase activities (Esposito et al., 2011). Furthermore, alizarin is the core part of anthraquinones, which constitute numerous antitumor drugs widely applied in the treatment of various neoplasms such as Adriamycin and daunorubicin, and their coordination with Fe3+ was shown to diminish cardiotoxicity while improving the antitumor activity in chemotherapy and maintain sound radiosensitizing properties in radiotherapy (Lown, 1993; Nowak and Tarasiuk, 2012; Malik and Müller, 2016). Therefore, this novel molecular platform also indicates the potential for cancer therapy and imaging by utilizing the β-gal responsive turn on pathway to selectively deplete tumor Fe3+, resulting in cancer cell cycle arrest and apoptosis while generating 1H-MRI contrast enhancement, thereby providing insight into the lacZ gene expression, development, location, and magnitude.
Conclusion
In this study, we present a novel responsive molecular platform for β-gal activity detection using 1H-MRI, in which the 1H-MRI signal enhancement is specifically generated, localized, and accumulated in situ at the β-gal activity site. In conjunction with this design, we have successfully produced and characterized alizarin 2-O-β-d-galactopyranoside AZ-1 and alizarin 1,2-di-O-β-d-galactopyranoside AZ-2. We have also demonstrated the feasibility of using AZ-1 by spontaneous in situ formation of paramagnetic Fe3+–alizarin complex to assess the β-gal activity in solution with Fe3+ ions existence by 1H-MRI T1 and T2/T2* relaxation mapping. 1H-MRI clearly showed the significant differences in both T1 and T2 at WT vs. lacZ gene expressing cells in culture after incubation with AZ-1, signifying the potential of integrating T1 and T2 data together to gain the additional certainty in imaging evaluation and detection reliability of β-gal activity.
Experimental
General Methods
NMR spectra were recorded on a Varian Unity INOVA 400 spectrometer (400 MHz for 1H, 100 MHz for 13C). 1H and 13C chemical shifts are referenced to TMS as an internal standard with CDCl3, or DMSO-d6 as solvents, and chemical shifts are given in ppm. All compounds were characterized by NMR at 25°C. Mass spectra were obtained by positive and negative ESI-MS using a Micromass Q-TOF hybrid quadrupole/time-of-flight instrument (Micromass UK Ltd.). Absorption spectra were taken on a UV-2700 UV-Vis Shimadzu spectrophotometer.
Solutions in organic solvents were dried with anhydrous sodium sulfate and concentrated in vacuo below 45°C. 2, 3, 4, 6-Tetra-O-acetyl-α-D-galactopyranosyl bromide was purchased from the Sigma Chemical Company. β-Gal (E801A) was purchased from the Promega (Madison, WI, United States), and enzymatic reactions were performed at 37°C in the PBS solution (0.1 M, pH = 7.4). Column chromatography was performed on silica gel (200–300 mesh), and silica gel GF254 used for analytical TLC was purchased from the Aldrich Chemical Company. The detection was affected by spraying the plates with 5% ethanolic H2SO4 (followed by heating at 110°C for 10 min) or by direct UV illumination of the plate. The purity of the final products was determined by HPLC with ≥95%.
Alizarin 2-O-2′, 3′, 4′, 6′-Tetra-O-Acetyl-β-D-Galactopyranoside AZ-M1
A solution of 2, 3, 4, 6-tetra-O-acetyl-α-D-galactopyranosyl bromide (1.23 g, 3.0 mmol) in CH2Cl2 (15 ml) was added dropwise to a vigorously stirred CH2Cl2-H2O biphasic mixture (pH 8–9) of alizarin (0.72 g, 3.0 mmol) and tetrabutylammonium bromide (TBAB) (322 mg, 1.0 mmol) in CH2Cl2-H2O (30 ml, 1:1 V/V′) around 1 h at 50°C under N2 atmosphere, with stirring continued for an additional 4–5 h until TLC showed that the reaction was completed. The product was extracted with CH2Cl2 (4 × 30 ml) and subsequently washed (H2O), dried (Na2SO4), and evaporated under reduced pressure to give a syrup, which was purified by column chromatography on silica gel to give alizarin 2-O-2′, 3′, 4′, 6′-tetra-O-acetyl-β-D-galactopyranoside AZ-M1.
Alizarin 1,2-Di-O-2′, 3′, 4′, 6′-Tetra-O-Acetyl-β-D-Galactopyranoside AZ-M2
A solution of 2, 3, 4, 6-tetra-O-acetyl-α-D-galactopyranosyl bromide (2.71 g, 6.6 mmol) in CH2Cl2 (30 ml) was added dropwise to a vigorously stirred CH2Cl2-H2O biphasic mixture (pH 10–11) of alizarin (0.72 g, 3.0 mmol) and TBAB (322 mg, 1.0 mmol) in CH2Cl2-H2O (30 ml, 1:1 V/V′) around 1 h at 55°C under N2 atmosphere, with stirring continued for an additional 4–5 h until TLC showed that the reaction was completed. The product was extracted with CH2Cl2 (4 × 40 ml) and subsequently washed (H2O), dried (Na2SO4), and evaporated under reduced pressure to give a syrup, which was purified by column chromatography on silica gel to give alizarin 1,2-di-O-2′, 3′, 4′, 6′-tetra-O-acetyl-β-D-galactopyranoside AZ-M2.
Alizarin 2-O-β-D-Galactopyranoside AZ-1 and Alizarin 1,2-Di-O-β-D-Galactopyranoside AZ-2
General procedure: A solution of alizarin 2-O-2′, 3′, 4′, 6′-tetra-O-acetyl-β-D-galactopyranoside AZ-M1 or alizarin 1,2-di-O-2′, 3′, 4′, 6′-tetra-O-acetyl-β-D-galactopyranoside AZ-M2 (1.30 g) in anhydrous MeOH (120 ml) containing 0.5 M NH3 was vigorously stirred from 0°C to room temperature overnight until TLC showed that the reaction was complete and then evaporated to dryness in vacuo. Chromatography of the crude syrup on silica gel with ethyl acetate-methanol afforded the corresponding alizarin 2-O-β-D-galactopyranoside AZ-1 and alizarin 1,2-di-O-β-D-galactopyranoside AZ-2 in high yields.
MRI
MRI studies were performed using a 4.7T horizontal bore magnet or a 9.4T vertical bore magnet equipped with a Varian INOVA Unity system (Palo Alto, CA, United States). T1 and T2 (or T2*) maps were acquired using a spin echo (or gradient echo) sequence with varying repetition times (TRs) or echo times (TEs), respectively. The raw data were acquired using a centric k-space reordering scheme, followed by the phase encoding steps with higher phase encoding gradient amplitudes. Data acquisition parameters of the FLASH readout were TR/TE/Flip angle = 10 ms/5 ms/10°. The standard multi-echo Carr–Purcell–Meiboom–Gill pulse sequence was used for measuring T2 from a single echo train. The T2 and T2* maps were obtained on a voxel-by-voxel basis using a nonlinear least-squares fit equation M = M0e−TE/T2 from the images taken at each echo time. Images were reconstructed and analyzed by using MatLab (MathWorks, Natick, MA).
lacZ Transfection in Human Tumor Cells
The E. coli lacZ gene (from pSV-β-gal vector, Promega, Madison, WI, United States) was inserted into a high expression human cytomegalovirus (CMV) immediate early enhancer/promoter vector phCMV (Gene Therapy Systems, San Diego, CA, United States), producing a recombinant vector phCMV/lacZ. This was used to transfect wild-type MCF7 (human breast cancer) and PC3 (human prostate cancer) cells (ATCC, Manassas, VA, United States) using GenePORTER2 (Gene Therapy Systems, Genlantis, Inc., San Diego, CA, United States). The highest β-gal expressing colony was selected using the antibiotic G418 disulfate (800 μg/ml, Research Products International Corp, Mt Prospect, IL, United States), and G418 (200 μg/ml) was also included for routine culture. The cells were maintained in Dulbecco’s modified Eagle’s medium (DMEM, Mediatech Inc., Herndon, VA, United States) containing 10% fetal bovine serum (FBS, 0.1 M, pH = 7.4, Atlanta Biologicals, Inc., Lawrenceville, GA, United States) with 100 units/mL of penicillin and 100 units/mL streptomycin, and cultured in a humidified 5% CO2 incubator at 37°C. The β-gal activity of lacZ-transfected tumor cells was measured using a β-Gal Assay Kit with o-nitrophenyl-β-D-galactopyranoside (Promega, Madison, WI, United States) and confirmed by X-gal or S-gal staining. Cells were fixed in PBS plus 0.5% glutaraldehyde (5 min) and rinsed in PBS prior to staining. Staining was performed using standard procedures for 2 h at 37°C in PBS plus 1 mg/ml X-gal (Sigma, St. Louis, MO, United States), 1 mM MgCl2, 5 mM K3Fe(CN)6, and 5 mM K4Fe(CN)6 or with 1.5 mg/mL S-gal (Sigma, St. Louis, MO, United States) and 2.5 mg/ml FAC.
Western Blot
The protein extracted from the wild-type and lacZ-expressing MCF7 and PC3 cancer cells was quantified using the Bradford method by a protein assay (Bio-Rad, Hercules, CA, United States). Protein (30 μg) was added to each well, separated by 10% sodium dodecyl sulfate-polyacrylamide gel electrophoresis, and transferred to a polyvinylidene fluoride membrane. A primary monoclonal anti–β-gal antibody (Promega, Madison, WI, United States) and anti-actin antibody (Sigma, St. Louis, MO, United States) were used as probes at a dilution of 1:5,000, with the reacting protein detected using a horseradish peroxidase–conjugated secondary antibody and ECL detection (Amersham, Piscataway, NJ, United States).
Cytotoxicity
The cytotoxicity for the free β-D-galactopyranoside AZ-1 and the released aglycone alizarine was assessed in both wild-type and lacZ- expressing MCF7 and PC3 cells using a colorimetric CellTiter 96 Aqueous Non-Radioactive Cell Proliferation Assay (MTS) (Promega, Madison, WI, United States). Assays were performed in triplicate using 24-well plates seeded with 103 cells per well in 500 μL of RPMI-1640 without phenol red and supplemented with 10% FCS and 2 mM glutamine (Urano et al., 2005; Kamiya et al., 2007).
Data Availability Statement
The datasets presented in this study can be found in online repositories. The names of the repository/repositories and accession number(s) can be found in the article/Supplementary Material.
Author Contributions
J-XY: conceived and designed the study, analyzed the data, and wrote the manuscript. SG, LZ, and ZF: synthesized, purified the compounds, and performed most part of NMR experiments. VK: conducted 1H-MRI experiments with PC3-lacZ cells and demonstrated the feasibility for detection of the lacZ gene expression. LL: conducted lacZ transfection in tumor cells and validated the β-gal activity. HW: assisted in toxicity evaluation. HX: helped with structural characterization. MT, BH, CC, and ZZ: assisted in processing data.
Funding
This research was supported in part by the Research/Development Grants (2016QDJZR01 and HBMUPI201808) from the Biomedical Research Institute, fifth School of Medicine/Suizhou Central Hospital, Hubei University of Medicine, and the Natural Science Foundation of China (21877028).
Conflict of Interest
The authors declare that the research was conducted in the absence of any commercial or financial relationships that could be construed as a potential conflict of interest.
Supplementary Material
The Supplementary Material for this article can be found online at: https://www.frontiersin.org/articles/10.3389/fchem.2021.709581/full#supplementary-material
Abbreviations
NMR, nuclear magnetic resonance; MRS, magnetic resonance spectroscopy; MRI, magnetic resonance imaging; TR, repetition time; TE, echo time; β-gal, β-galactosidase; FAC, ferric ammonium citrate, TBAB, tetrabutylammonium bromide; CH2Cl2, dichloromethane; HRMS, high-resolution mass spectrometry; PBS, phosphate-buffered saline; X-Gal, 5-bromo-4-chloro-3-indolyl-β-D-galactopyranoside; S-Gal, 3,4-cyclohexenoesculetin β-D-galactopyranoside; DMSO, dimethyl sulfoxide; TLC, thin-layer chromatography.
References
Alam, J., and Cook, J. L. (1990). Reporter Genes: Application to the Study of Mammalian Gene Transcription. Anal. Biochem. 188, 245–254. doi:10.1016/0003-2697(90)90601-5
Arena, F., Singh, J. B., Gianolio, E., Stefanìa, R., and Aime, S. (2011). β-Gal Gene Expression MRI Reporter in Melanoma Tumor Cells. Design, Synthesis, Andin Vitroandin VivoTesting of a Gd(III) Containing Probe Forming a High Relaxivity, Melanin-like Structure upon β-Gal Enzymatic Activation. Bioconjug. Chem. 22, 2625–2635. doi:10.1021/bc200486j
Asanuma, D., Sakabe, M., Kamiya, M., Yamamoto, K., Hiratake, J., Ogawa, M., et al. (2015). Sensitive β-galactosidase-targeting Fluorescence Probe for Visualizing Small Peritoneal Metastatic Tumours In Vivo. Nat. Commun. 6, 6463–6470. doi:10.1038/ncomms7463
Bengtsson, N. E., Brown, G., Scott, E. W., and Walter, G. A. (2010). LacZ as a Genetic Reporter for Real-Time MRI. Magn. Reson. Med. 63, 745–753. doi:10.1002/mrm.22235
Bertozzi, C. R., and Kiessling, L. L. (2001). Chemical Glycobiology. Science 291, 2357–2364. doi:10.1126/science.1059820
Beutler, E. (2004). CELL BIOLOGY: " Pumping" Iron: The Proteins. Science 306, 2051–2053. doi:10.1126/science.1107224
Broome, A.-M., Ramamurthy, G., Lavik, K., Liggett, A., Kinstlinger, I., and Basilion, J. (2015). Optical Imaging of Targeted β-Galactosidase in Brain Tumors to Detect EGFR Levels. Bioconjug. Chem. 26, 660–668. doi:10.1021/bc500597y
Browne, N. K., Huang, Z., Dockrell, M., Hashmi, P., and Price, R. G. (2010). Evaluation of New Chromogenic Substrates for the Detection of Coliforms. J. Appl. Microbiol. 108, 1828–1838. doi:10.1111/j.1365-2672.2009.04588.x
Burke, H. M., Gunnlaugsson, T., and Scanlan, E. M. (2015). Recent Advances in the Development of Synthetic Chemical Probes for Glycosidase Enzymes. Chem. Commun. 51, 10576–10588. doi:10.1039/c5cc02793d
Buss, J., Torti, F., and Torti, S. (2003). The Role of Iron Chelation in Cancer Therapy. Cmc 10, 1021–1034. doi:10.2174/0929867033457638
Celen, S., Deroose, C., Groot, T. d., Chitneni, S. K., Gijsbers, R., Debyser, Z., et al. (2008). Synthesis and Evaluation of 18F- and 11C-Labeled Phenyl-Galactopyranosides as Potential Probes for In Vivo Visualization of LacZ Gene Expression Using Positron Emission Tomography. Bioconjug. Chem. 19, 441–449. doi:10.1021/bc700216d
Chang, Y.-T., Cheng, C.-M., Su, Y.-Z., Lee, W.-T., Hsu, J.-S., Liu, G.-C., et al. (2007). Synthesis and Characterization of a New Bioactivated Paramagnetic Gadolinium(III) Complex [Gd(DOTA-FPG)(H2O)] for Tracing Gene Expression. Bioconjug. Chem. 18, 1716–1727. doi:10.1021/bc070019s
Chatterjee, S. K., Bhattacharya, M., and Barlow, J. J. (1979). Glycosyltransferase and Glycosidase Activities in Ovarian Cancer Patients. Cancer Res. 39, 1943–1951.
Chen, J., Jackson, A. A., Rotello, V. M., and Nugen, S. R. (2016). Colorimetric Detection of Escherichia coli Based on the Enzyme-Induced Metallization of Gold Nanorods Based on the Enzyme-Induced Metallization of Gold Nanorods. Small 12, 2469–2475. doi:10.1002/smll.201503682Escherichia Coli
Chen, X., Ma, X., Zhang, Y., Gao, G., Liu, J., Zhang, X., et al. (2018). Ratiometric Fluorescent Probes with a Self-Immolative Spacer for Real-Time Detection of β-galactosidase and Imaging in Living Cells. Analytica Chim. Acta 1033, 193–198. doi:10.1016/j.aca.2018.05.071
Chen, X., Zhang, X., Ma, X., Zhang, Y., Gao, G., Liu, J., et al. (2019). Novel Fluorescent Probe for Rapid and Ratiometric Detection of β-galactosidase and Live Cell Imaging. Talanta 192, 308–313. doi:10.1016/j.talanta.2018.09.061
Coskuner, O., and Gonzalez, C. A. (2011). in Metallic Systems: A Quantum Chemists Perspective, Chapter 3, Carbohydrate and Trivalent Iron Ion Interactions. Editors T. C. Allison, and O. Coskunner (Gonzalez CA: CRC Press, Taylor & Francis Group), 83–106. 1420060775. 9781420060775.
Cui, W., Liu, L., Kodibagkar, V. D., and Mason, R. P. (2010). S-Gal, A Novel 1H MRI Reporter for β-galactosidase. Magn. Reson. Med. 64, 66–71. doi:10.1002/mrm.22400
Das, S., Bhattacharya, A., Mandal, P. C., Rath, M. C., and Mukherjee, T. (2002). One-electron Reduction of 1,2-Dihydroxy-9,10-Anthraquinone and Some of its Transition Metal Complexes in Aqueous Solution and in Aqueous Isopropanol-Acetone-Mixed Solvent: A Steady-State and Pulse Radiolysis Study. Radiat. Phys. Chem. 65, 93–100. doi:10.1016/s0969-806x(01)00451-0
Das, S., Saha, A., and Mandal, P. C. (1995). Radiosensitization of Thymine by Fe(III)-1,2 Dihydroxyanthraquinone Complex in Dilute Aqueous Solution. J. Radioanal. Nucl. Chem. Articles 196, 57–63. doi:10.1007/bf02036289
Davies, J. A., Dutremez, S. G., Hockensmith, C. M., Keck, R., Richardson, N., Selman, S., et al. (1996). Iron-based Second-Sphere Contrast Agents for Magnetic Resonance Imaging: Development of a Model System and Evaluation of Iron (III) Tris (Tironate) Complex in Rats. Acad. Radiol. 3, 936–945. doi:10.1016/s1076-6332(96)80305-9
Dimri, G. P., Lee, X., Basile, G., Acosta, M., Scott, G., Roskelley, C., et al. (1995). A Biomarker that Identifies Senescent Human Cells in Culture and in Aging Skin In Vivo. Proc. Natl. Acad. Sci. 92, 9363–9367. doi:10.1073/pnas.92.20.9363
Doura, T., Kamiya, M., Obata, F., Yamaguchi, Y., Hiyama, T. Y., Matsuda, T., et al. (2016). Detection ofLacZ-Positive Cells in Living Tissue with Single-Cell Resolution. Angew. Chem. Int. Ed. 55, 9620–9624. doi:10.1002/anie.201603328
Duo, T., Robinson, K., Greig, I. R., Chen, H.-M., Patrick, B. O., and Withers, S. G. (2017). Remarkable Reactivity Differences between Glucosides with Identical Leaving Groups. J. Am. Chem. Soc. 139, 15994–15999. doi:10.1021/jacs.7b09645
Dwek, R. A. (1996). Glycobiology: Toward Understanding the Function of Sugars. Chem. Rev. 96, 683–720. doi:10.1021/cr940283b
Esposito, F., Kharlamova, T., Distinto, S., Zinzula, L., Cheng, Y. C., Dutschman, G., et al. (2011). Alizarin Derivatives as New Dual Inhibitors of the HIV-1 Reverse Transcriptase-Associated DNA Polymerase and RNase H Activities Effective Also on the RNase H Activity of Non-nucleoside Resistant Reverse Transcriptases. FEBS J. 278, 1441–1457. doi:10.1111/j.1742-4658.2011.08057.x
Feng, F., Liu, L., and Wang, S. (2009). Water-Soluble Conjugated Polyelectrolyte-Based Fluorescence Enzyme Coupling Protocol for Continuous and Sensitiveβ-Galactosidase Detection. Macromol. Chem. Phys. 210, 1188–1193. doi:10.1002/macp.200900264
Fernández-Cuervo, G., Tucker, K. A., Malm, S. W., Jones, K. M., and Pagel, M. D. (2016). Diamagnetic Imaging Agents with a Modular Chemical Design for Quantitative Detection of β-Galactosidase and β-Glucuronidase Activities with CatalyCEST MRI. Bioconjug. Chem. 27, 2549–2557. doi:10.1021/acs.bioconjchem.6b00482
Fernández-Tejada, A., Cañada, F. J., and Jiménez-Barbero, J. (2015). Recent Developments in Synthetic Carbohydrate-Based Diagnostics, Vaccines, and Therapeutics. Chem. Eur. J. 21, 10616–10628. doi:10.1002/chem.201500831
Fu, W., Yan, C., Zhang, Y., Ma, Y., Guo, Z., and Zhu, W.-H. (2019). Near-Infrared Aggregation-Induced Emission-Active Probe Enables In Situ and Long-Term Tracking of Endogenous β-Galactosidase Activity. Front. Chem. 7, 291. doi:10.3389/fchem.2019.00291
Gao, Z., Gao, H., Zheng, D., Xu, T., Chen, Y., Liang, C., et al. (2020). β-Galactosidase Responsive AIE Fluorogene for Identification and Removal of Senescent Cancer Cells. Sci. China Chem. 63, 398–403. doi:10.1007/s11426-019-9659-2
Gorai, T., and Maitra, U. (2018). Eu/Tb Luminescence for Alkaline Phosphatase and β-galactosidase Assay in Hydrogels and on Paper Devices. J. Mater. Chem. B 6, 2143–2150. doi:10.1039/c7tb02657a
Green, O., Gnaim, S., Blau, R., Eldar-Boock, A., Satchi-Fainaro, R., and Shabat, D. (2017). Near-Infrared Dioxetane Luminophores with Direct Chemiluminescence Emission Mode. J. Am. Chem. Soc. 139, 13243–13248. doi:10.1021/jacs.7b08446
Gu, K., Qiu, W., Guo, Z., Yan, C., Zhu, S., Yao, D., et al. (2019). An Enzyme-Activatable Probe Liberating AIEgens: On-Site Sensing and Long-Term Tracking of β-galactosidase in Ovarian Cancer Cells. Chem. Sci. 10, 398–405. doi:10.1039/c8sc04266g
Gu, K., Xu, Y., Li, H., Guo, Z., Zhu, S., Zhu, S., et al. (2016). Real-Time Tracking and In Vivo Visualization of β-Galactosidase Activity in Colorectal Tumor with a Ratiometric Near-Infrared Fluorescent Probe. J. Am. Chem. Soc. 138, 5334–5340. doi:10.1021/jacs.6b01705
Gulaka, P. K., Yu, J.-X., Liu, L., Mason, R. P., and Kodibagkar, V. D. (2013). Novel S-Gal Analogs as 1H MRI Reporters for In Vivo Detection of β-galactosidase. Magn. Reson. Imaging 31, 1006–1011. doi:10.1016/j.mri.2013.03.001
Haas, K. L., and Franz, K. J. (2009). Application of Metal Coordination Chemistry to Explore and Manipulate Cell Biology. Chem. Rev. 109, 4921–4960. doi:10.1021/cr900134a
Haberkorn, U., Mier, W., and Eisenhut, M. (2005). Scintigraphic Imaging of Gene Expression and Gene Transfer. Cmc 12, 779–794. doi:10.2174/0929867053507351
Han, J., Han, M. S., and Tung, C.-H. (2013). A Fluorogenic Probe for β-galactosidase Activity Imaging in Living Cells. Mol. Biosyst. 9, 3001–3008. doi:10.1039/c3mb70269c
Hananya, N., and Shabat, D. (2019). Recent Advances and Challenges in Luminescent Imaging: Bright Outlook for Chemiluminescence of Dioxetanes in Water. ACS Cent. Sci. 5, 949–959. doi:10.1021/acscentsci.9b00372
Hanaoka, K., Kikuchi, K., Terai, T., Komatsu, T., and Nagano, T. (2008). A Gd3+-Based Magnetic Resonance Imaging Contrast Agent Sensitive to β-Galactosidase Activity Utilizing a Receptor-Induced Magnetization Enhancement (RIME) Phenomenon. Chem. Eur. J. 14, 987–995. doi:10.1002/chem.200700785
Haris, M., Yadav, S. K., Rizwan, A., Singh, A., Wang, E., Hariharan, H., et al. (2015). Molecular Magnetic Resonance Imaging in Cancer. J. Transl. Med. 13, 313–329. doi:10.1186/s12967-015-0659-x
Heffern, M. C., Matosziuk, L. M., and Meade, T. J. (2014). Lanthanide Probes for Bioresponsive Imaging. Chem. Rev. 114, 4496–4539. doi:10.1021/cr400477t
Hingorani, D. V., Bernstein, A. S., and Pagel, M. D. (2015). A Review of Responsive MRI Contrast Agents: 2005-2014. Contrast Media Mol. Imaging 10, 245–265. doi:10.1002/cmmi.1629
Hu, J., Wu, Q., Cheng, K., Xie, Y., Li, C., and Li, Z. (2017). A 19F NMR Probe for the Detection of β-galactosidase: Simple Structure with Low Molecular Weight of 274.2, "Turn-On" Signal without the Background, and Good Performance Applicable in Cancer Cell Line. J. Mater. Chem. B 5, 4673–4678. doi:10.1039/c7tb00616k
Hu, Q., Ma, K., Mei, Y., He, M., Kong, J., and Zhang, X. (2017). Metal-to-ligand Charge-Transfer: Applications to Visual Detection of β-galactosidase Activity and sandwich Immunoassay. Talanta 167, 253–259. doi:10.1016/j.talanta.2017.02.027
Huang, J., Li, N., Wang, Q., Gu, Y., and Wang, P. (2017). A Lysosome-Targetable and Two-Photon Fluorescent Probe for Imaging Endogenous β-galactosidase in Living Ovarian Cancer Cells. Sensors Actuators B: Chem. 246, 833–839. doi:10.1016/j.snb.2017.02.158
Huang, Y., Feng, H., Liu, W., Zhang, S., Tang, C., Chen, J., et al. (2017). Cation-driven Luminescent Self-Assembled Dots of Copper Nanoclusters with Aggregation-Induced Emission for β-galactosidase Activity Monitoring. J. Mater. Chem. B 5, 5120–5127. doi:10.1039/c7tb00901a
Hudak, J. E., and Bertozzi, C. R. (2014). Glycotherapy: New Advances Inspire a Reemergence of Glycans in Medicine. Chem. Biol. 21, 16–37. doi:10.1016/j.chembiol.2013.09.010
Ito, H., Kawamata, Y., Kamiya, M., Tsuda‐Sakurai, K., Tanaka, S., Ueno, T., et al. (2018). Red‐Shifted Fluorogenic Substrate for Detection of Lac Z‐Positive Cells in Living Tissue with Single‐Cell Resolution. Angew. Chem. Int. Ed. 57, 15702–15706. doi:10.1002/anie.201808670
James, A. L., Perry, J. D., Chilvers, K., Robson, I. S., Armstrong, L., and Orr, K. E. (2000). Alizarin-beta- D-Galactoside: a New Substrate for the Detection of Bacterial Beta-Galactosidase. Lett. Appl. Microbiol. 30, 336–340. doi:10.1046/j.1472-765x.2000.00669.x
Jiang, G., Zeng, G., Zhu, W., Li, Y., Dong, X., Zhang, G., et al. (2017). A Selective and Light-Up Fluorescent Probe for β-galactosidase Activity Detection and Imaging in Living Cells Based on an AIE Tetraphenylethylene Derivative. Chem. Commun. 53, 4505–4508. doi:10.1039/c7cc00249a
Jiang, J., Tan, Q., Zhao, S., Song, H., Hu, L., and Xie, H. (2019). Late-stage Difluoromethylation Leading to a Self-Immobilizing Fluorogenic Probe for the Visualization of Enzyme Activities in Live Cells. Chem. Commun. 55, 15000–15003. doi:10.1039/c9cc07903c
Josserand, V., Texier-Nogues, I., Huber, P., Favrot, M.-C., and Coll, J.-L. (2007). Non-invasive In Vivo Optical Imaging of the lacZ and Luc Gene Expression in Mice. Gene Ther. 14, 1587–1593. doi:10.1038/sj.gt.3303028
Juers, D. H., Matthews, B. W., and Huber, R. E. (2012). LacZβ-galactosidase: Structure and Function of an Enzyme of Historical and Molecular Biological Importance. Protein Sci. 21, 1792–1807. doi:10.1002/pro.2165
Kamiya, M., Asanuma, D., Kuranaga, E., Takeishi, A., Sakabe, M., Miura, M., et al. (2011). β-Galactosidase Fluorescence Probe with Improved Cellular Accumulation Based on a Spirocyclized Rhodol Scaffold. J. Am. Chem. Soc. 133, 12960–12963. doi:10.1021/ja204781t
Kamiya, M., Kobayashi, H., Hama, Y., Koyama, Y., Bernardo, M., Nagano, T., et al. (2007). An Enzymatically Activated Fluorescence Probe for Targeted Tumor Imaging. J. Am. Chem. Soc. 129, 3918–3929. doi:10.1021/ja067710a
Kaplan, C. D., and Kaplan, J. (2009). Iron Acquisition and Transcriptional Regulation. Chem. Rev. 109, 4536–4552. doi:10.1021/cr9001676
Kim, E.-J., Kumar, R., Sharma, A., Yoon, B., Kim, H. M., Lee, H., et al. (2017). In Vivo imaging of β-galactosidase Stimulated Activity in Hepatocellular Carcinoma Using Ligand-Targeted Fluorescent Probe. Biomaterials 122, 83–90. doi:10.1016/j.biomaterials.2017.01.009,
Kim, E.-J., Podder, A., Maiti, M., Lee, J. M., Chung, B. G., and Bhuniya, S. (2018). Selective Monitoring of Vascular Cell Senescence via β-Galactosidase Detection with a Fluorescent Chemosensor. Sensors Actuators B: Chem. 274, 194–200. doi:10.1016/j.snb.2018.07.171
Kodibagkar, V. D., Yu, J., Liu, L., Hetherington, H. P., and Mason, R. P. (2006). Imaging β-galactosidase Activity Using 19F Chemical Shift Imaging of LacZ Gene-Reporter Molecule 2-Fluoro-4-Nitrophenol-β-D-Galactopyranoside. Magn. Reson. Imaging 24, 959–962. doi:10.1016/j.mri.2006.04.003
Koide, Y., Urano, Y., Yatsushige, A., Hanaoka, K., Terai, T., and Nagano, T. (2009). Design and Development of Enzymatically Activatable Photosensitizer Based on Unique Characteristics of Thiazole orange. J. Am. Chem. Soc. 131, 6058–6059. doi:10.1021/ja900443b
Kong, X., Li, M., Dong, B., Yin, Y., Song, W., and Lin, W. (2019). An Ultrasensitivity Fluorescent Probe Based on the ICT-FRET Dual Mechanisms for Imaging β-Galactosidase In Vitro and Ex Vivo. Anal. Chem. 91, 15591–15598. doi:10.1021/acs.analchem.9b03639
Kruger, A., Schirrmacher, V., and Khokha, R. (1999). The Bacterial lacZ Gene: An Important Tool for Metastasis Research and Evaluation of New Cancer Therapies. Cancer Metastasis Rev. 17, 285–294.
Kuznik, N., and Wyskocka, M. (2016). Iron(III) Contrast Agent Candidates for MRI: A Survey of the Structure-Effect Relationship in the Last 15 Years of Studies. Eur. J. Inorg. Chem., 445–458. doi:10.1002/ejic.201501166
Lee, H. W., Heo, C. H., Sen, D., Byun, H.-O., Kwak, I. H., Yoon, G., et al. (2014). Ratiometric Two-Photon Fluorescent Probe for Quantitative Detection of β-Galactosidase Activity in Senescent Cells. Anal. Chem. 86, 10001–10005. doi:10.1021/ac5031013
Lee, H. W., Lim, C. S., Choi, H., Cho, M. K., Noh, C.-K., Lee, K., et al. (2019). Discrimination between Human Colorectal Neoplasms with a Dual-Recognitive Two-Photon Probe. Anal. Chem. 91, 14705–14711. doi:10.1021/acs.analchem.9b03951
Li, H., and Meade, T. J. (2019). Molecular Magnetic Resonance Imaging with Gd(III)-based Contrast Agents: Challenges and Key Advances. J. Am. Chem. Soc. 141, 17025–17041. doi:10.1021/jacs.9b09149
Li, X., Pan, Y., Chen, H., Duan, Y., Zhou, S., Wu, W., et al. (2020a). Specific Near-Infrared Probe for Ultrafast Imaging of Lysosomal β-Galactosidase in Ovarian Cancer Cells. Anal. Chem. 92, 5772–5779. doi:10.1021/acs.analchem.9b05121
Li, X., Qiu, W., Li, J., Chen, X., Hu, Y., Gao, Y., et al. (2020b). First-generation Species-Selective Chemical Probes for Fluorescence Imaging of Human Senescence-Associated β-galactosidase. Chem. Sci. 11, 7292–7301. doi:10.1039/d0sc01234c
Li, X., Zhang, Z., Yu, Z., Magnusson, J., and Yu, J.-X. (2013). Novel Molecular Platform Integrated Iron Chelation Therapy for 1H-MRI Detection of β-Galactosidase Activity. Mol. Pharmaceutics 10, 1360–1367. doi:10.1021/mp300627t
Li, Y., Ning, L., Yuan, F., Zhang, T., Zhang, J., Xu, Z., et al. (2020). Activatable Formation of Emissive Excimers for Highly Selective Detection of β-Galactosidase. Anal. Chem. 92, 5733–5740. doi:10.1021/acs.analchem.9b04806
Li, Z., Ren, M., Wang, L., Dai, L., and Lin, W. (2020). Development of a Red-Emissive Two-Photon Fluorescent Probe for Sensitive Detection of Beta-Galactosidase In Vitro and In Vivo. Sensors Actuators B: Chem. 307, 127643. doi:10.1016/j.snb.2019.127643
Lilley, L. M., Kamper, S., Caldwell, M., Chia, Z. K., Ballweg, D., Vistain, L., et al. (2020). Self‐Immolative Activation of β‐Galactosidase‐Responsive Probes for In Vivo MR Imaging in Mouse Models. Angew. Chem. Int. Ed. 59, 388–394. doi:10.1002/anie.201909933
Liu, H.-W., Chen, L., Xu, C., Li, Z., Zhang, H., Zhang, X.-B., et al. (2018). Recent Progresses in Small-Molecule Enzymatic Fluorescent Probes for Cancer Imaging. Chem. Soc. Rev. 47, 7140–7180. doi:10.1039/c7cs00862g
Liu, L., Kodibagkar, V. D., Yu, J.-X., and Mason, R. P. (2007). 19 F‐NMR Detection of lacZ Gene Expression via the Enzymic Hydrolysis of 2‐fluoro‐4‐nitrophenyl β‐D‐galactopyranoside In Vivo in PC3 Prostate Tumor Xenografts in the Mouse 1. FASEB j. 21, 2014–2019. doi:10.1096/fj.06-7366lsf
Liu, L., and Mason, R. P. (2010). Imaging β-Galactosidase Activity in Human Tumor Xenografts and Transgenic Mice Using a Chemiluminescent Substrate. PloS ONE 5, e12024–31. doi:10.1371/journal.pone.0012024
Louie, A. Y., Hüber, M. M., Ahrens, E. T., Rothbächer, U., Moats, R., Jacobs, R. E., et al. (2000). In Vivo visualization of Gene Expression Using Magnetic Resonance Imaging. Nat. Biotechnol. 18, 321–325. doi:10.1038/73780
Lown, J. W. (1993). Anthracycline and Anthraquinone Anticancer Agents: Current Status and Recent Developments. Pharmacol. Ther. 60, 185–214. doi:10.1016/0163-7258(93)90006-y
Lozano-Torres, B., Estepa-Fernández, A., Rovira, M., Orzáez, M., Serrano, M., Martínez-Máñez, R., et al. (2019). The Chemistry of Senescence. Nat. Rev. Chem. 3, 426–441. doi:10.1038/s41570-019-0108-0
Lozano-Torres, B., Galiana, I., Rovira, M., Garrido, E., Chaib, S., Bernardos, A., et al. (2017). An Off-On Two-Photon Fluorescent Probe for Tracking Cell Senescence In Vivo. J. Am. Chem. Soc. 139, 8808–8811. doi:10.1021/jacs.7b04985
Mahal, A., Villinger, A., and Langer, P. (2011). Site-selective Arylation of Alizarin and Purpurin Based on Suzuki-Miyaura Cross-Coupling Reactions. Eur. J. Org. Chem. 2011, 2075–2087. doi:10.1002/ejoc.201001497
Malik, E. M., and Müller, C. E. (2016). Anthraquinones as Pharmacological Tools and Drugs. Med. Res. Rev. 36, 705–748. doi:10.1002/med.21391
Mizukami, S., Matsushita, H., Takikawa, R., Sugihara, F., Shirakawa, M., and Kikuchi, K. (2011). 19F MRI Detection of β-galactosidase Activity for Imaging of Gene Expression. Chem. Sci. 2, 1151–1155.
Nakamura, Y., Mochida, A., Nagaya, T., Okuyama, S., Ogata, F., Choyke, P. L., et al. (2017). A Topically-Sprayable, Activatable Fluorescent and Retaining Probe, SPiDER-βGal for Detecting Cancer: Advantages of Anchoring to Cellular Proteins after Activation. Oncotarget 8, 39512–39521. doi:10.18632/oncotarget.17080
Nowak, R., and Tarasiuk, J. (2012). Anthraquinone Antitumour Agents, Doxorubicin, Pirarubicin and Benzoperimidine BP1, Trigger Caspase-3/caspase-8-dependent Apoptosis of Leukaemia Sensitive HL60 and Resistant HL60/VINC and HL60/DOX Cells. Anticancer Drugs 23, 380–392. doi:10.1097/cad.0b013e32834f8ab4
Oushiki, D., Kojima, H., Takahashi, Y., Komatsu, T., Terai, T., Hanaoka, K., et al. (2012). Near-infrared Fluorescence Probes for Enzymes Based on Binding Affinity Modulation of Squarylium Dye Scaffold. Anal. Chem. 84, 4404–4410. doi:10.1021/ac300061a
Pacheco-Rivera, R., Fattel-Fazenda, S., Arellanes-Robledo, J., Silva-Olivares, A., Alemán-Lazarini, L., Rodríguez-Segura, M., et al. (2016). Double Staining of β-galactosidase with Fibrosis and Cancer Markers Reveals the Chronological Appearance of Senescence in Liver Carcinogenesis Induced by Diethylnitrosamine. Toxicol. Lett. 241, 19–31. doi:10.1016/j.toxlet.2015.11.011
Pang, X., Li, Y., Zhou, Z., Lu, Q., Xie, R., Wu, C., et al. (2020). Visualization of Endogenous β-galactosidase Activity in Living Cells and Zebrafish with a Turn-On Near-Infrared Fluorescent Probe. Talanta 217, 121098. doi:10.1016/j.talanta.2020.121098
Paradis, V., Youssef, N., Dargère, D., Bâ, N., Bonvoust, F., Deschatrette, J., et al. (2001). Replicative Senescence in normal Liver, Chronic Hepatitis C, and Hepatocellular Carcinomas. Hum. Pathol. 32, 327–332. doi:10.1053/hupa.2001.22747
Peng, L., Gao, M., Cai, X., Zhang, R., Li, K., Feng, G., et al. (2015). A Fluorescent Light-Up Probe Based on AIE and ESIPT Processes for β-galactosidase Activity Detection and Visualization in Living Cells. J. Mater. Chem. B 3, 9168–9172. doi:10.1039/c5tb01938a
Qiu, W., Li, X., Shi, D., Li, X., Gao, Y., Li, J., et al. (2020). A Rapid-Response Near-Infrared Fluorescent Probe with a Large Stokes Shift for Senescence-Associated β-galactosidase Activity Detection and Imaging of Senescent Cells. Dyes Pigm. 182, 108657. doi:10.1016/j.dyepig.2020.108657
Razgulin, A., Ma, N., and Rao, J. (2011). Strategies for In Vivo Imaging of Enzyme Activity: An Overview and Recent Advances. Chem. Soc. Rev. 40, 4186–4216. doi:10.1039/c1cs15035a
Rempel, B. P., Price, E. W., and Phenix, C. P. (2017). Molecular Imaging of Hydrolytic Enzymes Using PET and SPECT. Mol. Imaging 16, 1536012117717852–30. doi:10.1177/1536012117717852
Richardson, D. R. (2005). Molecular Mechanisms of Iron Uptake by Cells and the Use of Iron Chelators for the Treatment of Cancer. Cmc 12, 2711–2729. doi:10.2174/092986705774462996
Richardson, N., Davies, J. A., and Radüchel, B. (1999). Iron(III)-based Contrast Agents for Magnetic Resonance Imaging. Polyhedron 18, 2457–2482. doi:10.1016/s0277-5387(99)00151-5
Sakabe, M., Asanuma, D., Kamiya, M., Iwatate, R. J., Hanaoka, K., Terai, T., et al. (2013). Rational Design of Highly Sensitive Fluorescence Probes for Protease and Glycosidase Based on Precisely Controlled Spirocyclization. J. Am. Chem. Soc. 135, 409–414. doi:10.1021/ja309688m
Schwert, D. D., Davies, J. A., and Richardson, N. (2002). Non-gadolinium-based MRI Contrast Agents. Top. Curr. Chem. 221, 165–199. doi:10.1007/3-540-45733-x_6
Schwert, D. D., Richardson, N., Ji, G., Radüchel, B., Ebert, W., Heffner, P. E., et al. (2005). Synthesis of Two 3,5-disubstituted Sulfonamide Catechol Ligands and Evaluation of Their Iron(III) Complexes for Use as MRI Contrast Agents. J. Med. Chem. 48, 7482–7485. doi:10.1021/jm0501984
Sharma, S. K., and Leblanc, R. M. (2017). Biosensors Based on β-galactosidase Enzyme: Recent Advances and Perspectives. Anal. Biochem. 535, 1–11. doi:10.1016/j.ab.2017.07.019
Shi, L., Yan, C., Ma, Y., Wang, T., Guo, Z., and Zhu, W.-H. (2019). In Vivo ratiometric Tracking of Endogenous β-galactosidase Activity Using an Activatable Near-Infrared Fluorescent Probe. Chem. Commun. 55, 12308–12311. doi:10.1039/c9cc06869d
Singh, H., Tiwari, K., Tiwari, R., Pramanik, S. K., and Das, A. (2019). Small Molecule as Fluorescent Probes for Monitoring Intracellular Enzymatic Transformations. Chem. Rev. 119, 11718–11760. doi:10.1021/acs.chemrev.9b00379
Tang, C., Zhou, J., Qian, Z., Ma, Y., Huang, Y., and Feng, H. (2017). A Universal Fluorometric Assay Strategy for Glycosidases Based on Functional Carbon Quantum Dots: β-galactosidase Activity Detection In Vitro and in Living Cells. J. Mater. Chem. B 5, 1971–1979. doi:10.1039/c6tb03361j
Terreno, E., Castelli, D. D., Viale, A., and Aime, S. (2010). Challenges for Molecular Magnetic Resonance Imaging. Chem. Rev. 110, 3019–3042. doi:10.1021/cr100025t
Theil, E. C., and Goss, D. J. (2009). Living with Iron (And Oxygen): Questions and Answers about Iron Homeostasis. Chem. Rev. 109, 4568–4579. doi:10.1021/cr900052g
Tung, C.-H., Zeng, Q., Shah, K., Kim, D.-E., Schellingerhout, D., and Weissleder, R. (2004). In Vivo Imaging of β-Galactosidase Activity Using Far Red Fluorescent Switch. Cancer Res. 64, 1579–1583. doi:10.1158/0008-5472.can-03-3226
Urano, Y., Kamiya, M., Kanda, K., Ueno, T., Hirose, K., and Nagano, T. (2005). Evolution of Fluorescein as a Platform for Finely Tunable Fluorescence Probes. J. Am. Chem. Soc. 127, 4888–4894. doi:10.1021/ja043919h
Van Dort, M. E., Lee, K. C., Hamilton, C. A., Rebemtulla, A., and Ross, B. D. (2008). Radiosynthesis and Evaluation of 5-[125I]iodoindol-3-Yl-β-D-Galactopyranoside as a β-galactosidase Imaging Radioligand. Mol. Imaging 7, 187–197. doi:10.2310/7290.2008.00020
Wahsner, J., Gale, E. M., Rodríguez-Rodríguez, A., and Caravan, P. (2019). Chemistry of MRI Contrast Agents: Current Challenges and New Frontiers. Chem. Rev. 119, 957–1057. doi:10.1021/acs.chemrev.8b00363
Wang, W., Vellaisamy, K., Li, G., Wu, C., Ko, C.-N., Leung, C.-H., et al. (2017). Development of a Long-Lived Luminescence Probe for Visualizing β-Galactosidase in Ovarian Carcinoma Cells. Anal. Chem. 89, 11679–11684. doi:10.1021/acs.analchem.7b03114
Wang, Y., Liu, J., Ma, X., Cui, C., Deenik, P. R., Henderson, P. K. P., et al. (2019). Real-time Imaging of Senescence in Tumors with DNA Damage. Sci. Rep. 9, 2102. doi:10.1038/s41598-019-38511-z
Weber, K. A., Achenbach, L. A., and Coates, J. D. (2006). Microorganisms Pumping Iron: Anaerobic Microbial Iron Oxidation and Reduction. Nat. Rev. Microbiol. 4, 752–764. doi:10.1038/nrmicro1490
Wehrman, T. S., von Degenfeld, G., Krutzik, P. O., Nolan, G. P., and Blau, H. M. (2006). Luminescent Imaging of β-galactosidase Activity in Living Subjects Using Sequential Reporter-Enzyme Luminescence. Nat. Methods 3, 295–301. doi:10.1038/nmeth868
Wei, X., Wu, Q., Zhang, J., Zhang, Y., Guo, W., Chen, M., et al. (2017). Synthesis of Precipitating Chromogenic/fluorogenic β-glucosidase/β-galactosidase Substrates by a New Method and Their Application in the Visual Detection of Foodborne Pathogenic Bacteria. Chem. Commun. 53, 103–106. doi:10.1039/c6cc07522c
Wu, C., Ni, Z., Li, P., Li, Y., Pang, X., Xie, R., et al. (2020). A Near-Infrared Fluorescent Probe for Monitoring and Imaging of β-galactosidase in Living Cells. Talanta 219, 121307. doi:10.1016/j.talanta.2020.121307
Xu, Z., Liu, C., Zhao, S., Chen, S., and Zhao, Y. (2019). Molecular Sensors for NMR-Based Detection. Chem. Rev. 119, 195–230. doi:10.1021/acs.chemrev.8b00202
Yang, C., Wang, Q., and Ding, W. (2019). Recent Progress in the Imaging Detection of Enzyme Activitiesin Vivo. RSC Adv. 9, 25285–25302. doi:10.1039/c9ra04508b
Yang, W., Zhao, X., Zhang, J., Zhou, Y., Fan, S., Sheng, H., et al. (2018). Hydroxyphenylquinazolinone-based Turn-On Fluorescent Probe for β-galactosidase Activity Detection and Application in Living Cells. Dyes Pigm. 156, 100–107. doi:10.1016/j.dyepig.2018.04.003
Yeung, K., Schmid, K. M., and Phillips, S. T. (2013). A Thermally-Stable Enzyme Detection Assay that Amplifies Signal Autonomously in Water without Assistance from Biological Reagents. Chem. Commun. 49, 394–396. doi:10.1039/c2cc36861g
Yu, J-X., Kodibagkar, V. D., Liu, L., Zhang, Z., Liu, L., Magnusson, J., et al. (2013). MRS/1H-MRI Dual-Function Probe for Detection of β-galactosidase Activity. Chem. Sci. 4, 2132–2142.
Yu, J.-X., Gulaka, P. K., Liu, L., Kodibagkar, V. D., and Mason, R. P. (2012a). Novel Fe3+-Based 1H MRI β-Galactosidase Reporter Molecules. ChemPlusChem 77, 370–378. doi:10.1002/cplu.201100072
Yu, J.-x., Kodibagkar, V., Cui, W., Mason, R., and F, (2005). 19F: A Versatile Reporter for Non-invasive Physiology and Pharmacology Using Magnetic Resonance. Cmc 12, 819–848. doi:10.2174/0929867053507342
Yu, J.-X., Kodibagkar, V. D., Hallac, R. R., Liu, L., and Mason, R. P. (2012b). Dual19F/1H MR Gene Reporter Molecules Forin VivoDetection of β-Galactosidase. Bioconjug. Chem. 23, 596–603. doi:10.1021/bc200647q
Yu, J.-X., Kodibagkar, V. D., Liu, L., and Mason, R. P. (2008a). A19F-NMR Approach Using Reporter Molecule Pairs to Assessβ-Galactosidase in Human Xenograft Tumorsin Vivo. NMR Biomed. 21, 704–712. doi:10.1002/nbm.1244
Yu, J., Liu, L., Kodibagkar, V. D., Cui, W., and Mason, R. P. (2006). Synthesis and Evaluation of Novel Enhanced Gene Reporter Molecules: Detection of β-galactosidase Activity Using 19F NMR of Trifluoromethylated Aryl β-d-galactopyranosides. Bioorg. Med. Chem. 14, 326–333. doi:10.1016/j.bmc.2005.08.021
Yu, J., and Mason, R. P. (2006). Synthesis and Characterization of Novel lacZ Gene Reporter Molecules: Detection of β-Galactosidase Activity by 19F Nuclear Magnetic Resonance of Polyglycosylated Fluorinated Vitamin B6. J. Med. Chem. 49, 1991–1999. doi:10.1021/jm051049o
Yu, J. X., Cui, W., Zhao, D., and Mason, R. P. (2008b). Non-invasive Physiology and Pharmacology Using 19F Magnetic Resonance, Fluorine & Health - Molecular Imaging. Biomedical Materials and Pharmaceuticals. (Amsterdam, Netherlands: Elsevier), 197–276. doi:10.1016/b978-0-444-53086-8.00005-9
Yu, Z., Zhao, J., Hua, Z., Wang, X., Wang, X., Wang, H., et al. (2017). Novel19F-MRS β-galactosidase Reporter Molecules Incorporated Nitrogen Mustard Analogues. Chem. Biol. Drug Des. 90, 719–729. doi:10.1111/cbdd.12992
Zeng, Z., Mizukami, S., Fujita, K., and Kikuchi, K. (2015). An Enzyme-Responsive Metal-Enhanced Near-Infrared Fluorescence Sensor Based on Functionalized Gold Nanoparticles. Chem. Sci. 6, 4934–4939. doi:10.1039/c5sc01850a
Zeng, Z., Mizukami, S., and Kikuchi, K. (2012). Simple and Real-Time Colorimetric Assay for Glycosidases Activity Using Functionalized Gold Nanoparticles and its Application for Inhibitor Screening. Anal. Chem. 84, 9089–9095. doi:10.1021/ac301677v
Zhang, C., Wang, D., Zhang, L., Guo, J.-F., and Ren, A.-M. (2016). Theoretical Investigation and Design of Two-Photon Fluorescent Probes for Visualizing β-galactosidase Activity in Living Cells. RSC Adv. 6, 70960–70971. doi:10.1039/c6ra11712k
Zhang, J., Chai, X., He, X.-P., Kim, H.-J., Yoon, J., and Tian, H. (2019a). Fluorogenic Probes for Disease-Relevant Enzymes. Chem. Soc. Rev. 48, 683–722. doi:10.1039/c7cs00907k
Zhang, J., Cheng, P., and Pu, K. (2019b). Recent Advances of Molecular Optical Probes in Imaging of β-Galactosidase. Bioconjug. Chem. 30, 2089–2101. doi:10.1021/acs.bioconjchem.9b00391
Zhang, J., Li, C., Dutta, C., Fang, M., Zhang, S., Tiwari, A., et al. (2017). A Novel Near-Infrared Fluorescent Probe for Sensitive Detection of β-galactosidase in Living Cells. Analytica Chim. Acta 968, 97–104. doi:10.1016/j.aca.2017.02.039
Zhang, X.-X., Wu, H., Li, P., Qu, Z.-J., Tan, M.-Q., and Han, K.-L. (2016). A Versatile Two-Photon Fluorescent Probe for Ratiometric Imaging E. coli β-galactosidase in Live Cells and In Vivo. Chem. Commun. 52, 8283–8286. doi:10.1039/c6cc04373a
Zhang, X., Chen, X., Zhang, Y., Liu, K., Shen, H., Zheng, E., et al. (2019). A Near-Infrared Fluorescent Probe for the Ratiometric Detection and Living Cell Imaging of β-galactosidase. Anal. Bioanal. Chem. 411, 7957–7966. doi:10.1007/s00216-019-02181-7
Zhao, X., Yang, W., Fan, S., Zhou, Y., Sheng, H., Cao, Y., et al. (2019). A Hemicyanine-Based Colorimetric Turn-On Fluorescent Probe for β-galactosidase Activity Detection and Application in Living Cells. J. Lumin. 205, 310–317. doi:10.1016/j.jlumin.2018.09.036
Zhou, Z., Bai, R., Munasinghe, J., Shen, Z., Nie, L., and Chen, X. (2017). T1-T2 Dual-Modal Magnetic Resonance Imaging: From Molecular Basis to Contrast Agents. ACS Nano 11, 5227–5232. doi:10.1021/acsnano.7b03075
Keywords: β-galactosidase detection, responsive Fe-based 1H-MRI agent, T1 and T2 relaxation mapping, in vitro1H-MRI imaging, lacZ gene reporter, synthesis
Citation: Gao S, Zhao L, Fan Z, Kodibagkar VD, Liu L, Wang H, Xu H, Tu M, Hu B, Cao C, Zhang Z and Yu J-X (2021) In Situ Generated Novel 1H MRI Reporter for β-Galactosidase Activity Detection and Visualization in Living Tumor Cells. Front. Chem. 9:709581. doi: 10.3389/fchem.2021.709581
Received: 14 May 2021; Accepted: 16 June 2021;
Published: 15 July 2021.
Edited by:
Jinwu Yan, South China University of Technology, ChinaReviewed by:
Chuqiao Tu, University of California, Davis, United StatesWei Chen, University of Texas at Arlington, United States
Copyright © 2021 Gao, Zhao, Fan, Kodibagkar, Liu, Wang, Xu, Tu, Hu, Cao, Zhang and Yu. This is an open-access article distributed under the terms of the Creative Commons Attribution License (CC BY). The use, distribution or reproduction in other forums is permitted, provided the original author(s) and the copyright owner(s) are credited and that the original publication in this journal is cited, in accordance with accepted academic practice. No use, distribution or reproduction is permitted which does not comply with these terms.
*Correspondence: Jian-Xin Yu, amlhbnhpbnl1NzA3QGdtYWlsLmNvbQ==
†These authors have contributed equally to this work