- 1Guizhou Provincial Key Laboratory for Rare Animal and Economic Insects of the Mountainous Region, College of Biology and Environmental Engineering, Guiyang University, Guiyang, China
- 2School of Chemistry and Chemical Engineering, Anshun University, Anshun, China
- 3State-Local Joint Laboratory for Comprehensive Utilization of Biomass, Guizhou University, Guiyang, China
Biomass, the only globally available, renewable feedstock of organic carbon, is considered a viable alternative to fossil fuels. It can be efficiently utilized to produce various building blocks in accordance with green and sustainable chemistry principles. In this review, recent progress, such as the transformation of carbohydrates (C5 or C6 sugar, inulin, and cellulose) and their derivatives (furfural, hydroxymethylfurfural) into significant platform chemicals over polyoxometalates, zeolites, non-noble metals, and ionic liquids in single or multiphase, is evaluated.
Introduction
Considerable effort must be devoted in transforming alternative and renewable resources to a form that would satisfy market requirements, industrial development, and remission environmental pressure given the increased consumption of nonrenewable resources and the environmental crises (such as global warming and chemical rain) (Tang et al., 2017). Biomass is the only globally available, abundant, and sustainable feedstock, and is considered a viable alternative to fossil fuels, which can be efficiently utilized for the preparation of various building blocks in accordance with green and sustainable chemistry. Soluble acids, which were previously used as catalysts for the conversion of biomass and its derivatives, are being discontinued because the complicated corrosion in the reaction contradicts green and sustainable principles. Nevertheless, fast mass transport, effective conversion of substrates, uniform texture, and good dispersion of the catalyst must be provided. This review summarizes the latest progress, such as the conversion of carbohydrates (C5 or C6 sugar, inulin, cellulose) and their derivatives [furfural, hydroxymethylfurfural (HMF) via hydrolysis, dehydration, hydrogenation, oxidation, hydrodeoxygenation, etc.] (Chheda et al., 2007; Fernandes et al., 2012; Gallezot, 2012) into significant platform chemicals (as shown in Table 1) over polyoxometalates, zeolites, non-noble metals, and ionic liquids in single or multiphase/s. The progress includes developments, such as catalysts affording tunable acidity and active sites, stable and uniform structure, and the excellent dispersion of catalytic centers to cater to sustainable requirements.
Sustainable Catalysts for Furan Derivatives
Upgrading of Furan Derivatives Over Polyoxometalate-Based Catalysts
Polyoxometalates (POMs) are a special class of anionic polynuclear metal-oxo clusters with structural multiplicity and countercations balanced with a negative charge. Owing to their adjustable Brønsted or Lewis acidic nature and reducibility, POMs and POM-based materials have been widely used for heterogeneous catalysis (Wang and Yang, 2015; Enferadi-Kerenkan et al., 2018; Weinstock et al., 2018). For biomass valorization, POMs have been used as green acids or oxidation agents (Wang et al., 2015; Weinstock et al., 2018) to produce renewable chemicals and platform molecules, including industrial chemicals (Hu et al., 2015; Wang et al., 2015), organic acids, furans, (Bulushev and Ross, 2018; Wang et al., 2018; Zhang and Huber, 2018), and biodiesels (Narkhede et al., 2015). Similarly, metal–POM complexes have been applied to upgrade biomass into building block chemicals and fuels (Li et al., 2016). However, POMs are generally soluble in water and polar organic solvents; inorganic cations (e.g., K+, Sn+, Cs+, Ag+, etc.) usually serve as countercations to increase the insolubility of POMs. In addition, multiple heterogeneous uniform supports, including metal oxides, zeolites, porous silica, MOFs, and hybrids have been employed to immobilize POMs.
It is well known that HMF, furfuryl alcohol (FAL), and lactic acid are listed as significant platform chemicals in the “Top 10 + 4” list provided by the US Department of Energy (DOE) (Bozell and Petersen, 2010). A few compounds from the list will be discussed in this review. To date, various POM-based materials have been used as catalysts in HMF, LA, EMF, and ethyl levulinate (EL) production.
The LA esterification reaction for alkyl levulinate production has been examined over numerous heterogeneous solid acid catalysts that exhibit comparatively high efficiency (Pileidis et al., 2016; Cirujano et al., 2015). A plausible reaction mechanism for LA esterification with alcohols has also been proposed (Figure 1). A heteropolyacid (HPA) contains metal atoms such as W, Mo, and V, and p-block elements such as P, Si, etc.; the oxygen atoms connecting the metal atoms and H+ ions for charge balancing are generally used for recycling acidic catalysts for the synthesis of fine chemicals (Yan et al., 2013; Wu et al., 2016a; Wu et al., 2016b; Wu et al., 2016c; Zhou et al., 2016; Manikandan et al., 2017; Ramli et al., 2017; Zhang et al., 2017; Luan et al., 2018; Vilanculo et al., 2018; Lucas et al., 2019). Numerous structural analogs can be synthesized by either replacing the metal or nonmetal atoms. Furthermore, these HPAs possess ordered metal oxide nanoclusters, strong Brønsted (or Lewis) acidity and can be applied almost entirely as homogenous or heterogeneous catalysts, or reaction solvents (Zhou et al., 2016; Wu et al., 2016a; Ramli et al., 2017; Zheng et al., 2017).
Zhao et al. (2011) reported excellent catalytic activity and thermal stability for HMF production from pristine lignocellulosic biomass catalyzed by a Brønsted–Lewis-surfactant-combined HPA catalyst, Cr [(DS)H2PW12O40]3 (DS represents dodecyl sulfate, OSO3C12H25). The result comprising 77.1% conversion of cellulose and 52.7% HMF yield is mainly ascribed to the synergistic acidities and micellar structure of the multihydrophobic groups. The stability and simple separation of the recycling catalyst demonstrate that micellar (HPA) Cr [(DS)H2PW12O40]3 satisfied green and sustainable principles. The aforementioned group synthesized a similar series of [HOCH2CH2N(CH3)3]xH3−xPW12O40 (ChxH3−xPW12O40, x = 1, 2, and 3) with choline chloride and HPW, and achieved a high yield of up to 75% by ChH2PW12O40 at 140°C for 8 h (Zhang et al., 2016). The hydrophilic head of ChxH3−xPW12O40 assembles the reactant cellulose, while the hydrophobic terminal suppresses the hydration side reaction of HMF into byproducts, thus, increasing the HMF yield.
Similarly, Sun et al. (2016) reported the original temperature-sensing HPA nanohybrid ChnH5−nAlW12O40 catalysts that could be dissolved at high temperatures to afford a homogeneous reaction medium and removed from the catalytic system at room temperature. Among the explored catalysts, ChnH5−nAlW12O40 (n = 0–5) and ChH4AlW12O40 exhibited an excellent LA yield of 74.8% and 98.9% conversion of cellulose with green methyl isobutyl ketone (MIBK) as a co-solvent in a one-pot scheme, respectively; the results were attributed to a combination of temperature stimulus and Lewis–Brønsted double acidity. Additionally, these HPA catalysts can be easily reused without structural breakdown or loss of weight.
EMF is a potential alternative biofuel derived from biomass due to its high energy density (30.3 MJ·L−1), similar to that of regular gasoline (31.9 MJ·L−1) and comparable to that of diesel (33.6 MJ·L−1); EMF exhibits lower emissions of soot, NOx, and SOx and, therefore, decreased contamination. Thus, EMF preparation from HMF or C5/C6 monose has drawn considerable attention (Liu and Wang, 2018). ALs has shown potential for use in fragrance and flavoring manufacturing, and as a mixture in biodiesel. EL is a novel diesel-blended biofuel.
Wang et al. (2016) developed MOF-based POMs (Cu-BTC)(HPM) (NENU-5) [benzene-1,3,5-tricarboxylate (BTC) and phosphomolybdic acid hydrate (HPM)] to produce EMF and EL based on HMF (Figure 2). (Cu-BTC) (HPM) is composed of guest molecules of HPM that support the micropores of the stable and regular Cu-BTC host and exhibit notable catalytic performance and stability. The highest EMF and EL yields of 68.4% and 20.2% were observed at 140°C over 12 h, respectively.
Su et al. (2013) reported on ZrO2-based organic–inorganic hybrid materials functionalized by hydrophilic Keggin-type HPA and hydrophobic alkyl groups (i.e., benzene terminally bonded, and ethane-/benzene-bridged organosilica moieties), H3PW12O40/ZrO2-Si(Et/Ph)Si, and H3PW12O40/ZrO2-Si(Ph). The prepared catalysts exhibited good catalytic performance and stability for the esterification of LA with alcohols. The authors surmised that the excellent catalytic activity was due to the following factors: the strong Brønsted and Lewis collaborative acidity, unique textural properties, and hydrophobic groups that suppress the strong adsorption of the hydrophilic byproducts. In particular, the unique 2D hexagonal mesostructured H3PW12O40/ZrO2-Si(Ph)Si exhibited superior activity compared with the 3D wormhole-like H3PW12O40/ZrO2-Si(Et)Si and H3PW12O40/ZrO2-Si(Ph) because of the efficient diffusion in the regular mesoporous structure. The same authors examined HPA and ZrO2 bifunctionalized organosilica nanohybrid materials, e.g., PW12/ZrO2-Si(Et)Si with a 1D hollow tubular nanostructure, a 2D hexagonal periodic mesostructure, and a 3D interconnected mesostructure (Song et al., 2016). Among the materials examined, HPW and ZrO2-bifunctionalized organosilica nanotubes PW12/ZrO2-Si(Et)Si-NTs, exhibited the optimal performance for alkyl levulinates by LA esterification.
Furthermore, impressive metal/Si-pillared montmorillonite-supported heterophosphotungstate catalysts (SG-HPW-XSiMt-y, where X = La3+, Ce3+, Er3+, Cu2+, Al3+, Ti4+, Zr4+) were prepared for the synthesis of methyl levulinate (ML) from glucose (Lai et al., 2021). The study demonstrated that the appropriate Lewis acidity of the metal ions facilitated the key isomerization steps of glucose and methylglucoside. The strong Brønsted acidity of HPW suppressed the side reactions, and the mesoporous structure enhanced the catalytic activity, which allowed the surface and inside active sites to take effect. The optimum catalyst (SG-HPW-ZrSiMt-20) achieved the highest activity (65.8% yield of ML from glucose) at 170°C for 4 h. The SG-HPW-ZrSiMt-20 can be reused eight times without any obvious loss of catalytic activity owing to the uniform dispersion and stable bonding of HPW with the ZrSiMt support.
Various methods have been developed to transform POMs into reusable heterogeneous catalysts. However, leaching of these catalysts to the environment occurs, and further efforts are required to devise appropriate support materials. Furthermore, other unexplored POM structures (e.g., Lindqvist type) should be considered for application in biomass valorization.
Zeolites as Catalysts for Upgrading Furan Derivatives
Zeolites with ordered micropores between 5 and 13 Å are usually highly structured crystalline aluminosilicates that exhibit superior thermal and chemical stability; most demonstrate Brønsted acidity. They assist the conversion of biomass substrates into platform molecules. Compared with microporous zeolites, mesoporous zeolites can afford excellent carrier materials with freedom from kinetic diffusion limitations in terms of larger reactants. Owing to their excellent structural features, high surface area, and relatively large pore size, mesoporous zeolites have been used to upgrade biomass for platform chemicals.
The design and preparation of zeolite catalysts that enhance product yield and afford good hydrothermal stability is a difficult and urgent problem that needs to be solved. This is evident from the reported literature on the conversion of biomass or biomass-derived platform chemicals.
It has been proven that zeolites are efficient for the dehydration of glucose to produce HMF. Mercedes explored a 57% conversion of glucose and a 1.6% yield of HMF in water at 195°C for 30 min; they employed H-ZSM-5 zeolites with a Lewis/Brønsted molar ratio of 0.25 as a catalyst. However, the application of a biphasic NaCl (20 wt%) aqueous solution/MIBK system by Moreno-Recio et al. (2016) achieved an enhanced conversion of 80% and an HMF yield of up to 42%. The group demonstrated that the catalytic activity was enhanced with the H-ZSM-5 zeolite by introducing NaCl in the biphasic reaction solvent, which is accredited with the inhibition of side reactions.
Based on the aforementioned research, Jiang et al. (2018) designed and prepared a zirconium-doped mesoporous KIT-6 catalyst (Zr-KIT-6) to transform glucose into HMF via dehydration. The highest glucose conversion (54.8%) and HMF yield (19.5%) were achieved at 170°C for 3 h in an aqueous phase. Similarly, the biphasic MIBK–water system increased the conversion of glucose to 79.0% and HMF yield to 34.5% under optimized conditions. This excellent catalytic activity is mainly ascribed to the high dispersion of ZrO2 nanoparticles and the existence of multicoordinated Zr4+ species in the Zr-KIT-6 materials.
Mesoporous SBA-15 materials are considered good candidates as supports for the dispersion of active sites because of their unique surface, pore texture with adjustable uniform hexagonal channels, and high hydrothermal stability (Verma et al., 2020). The group of Wu (Shi et al., 2011; Hua et al., 2013) constructed novel SBA-15-SO3H and AAO/SBA-15-SO3H catalysts processing active centers of sulfonic, carrier SBA-15, and substrates of porous alumina membranes (AAO). The xylose conversion and furfural selectivity were 90% and 70%, respectively, at 160°C for 4 h in a water/toluene two-phase reaction medium. The high reaction rate, mass transfer, and uniform distribution of the active sites in the developed AAO/SBA-15-SO3H catalyst contributed to its performance (Hua et al., 2013).
Ya’aini et al. (2013) assessed the LA yield of glucose valorization using a CrCl3/H-Y catalyst (1:1, w:w of CrCl3 and HY zeolite) composed mainly of hierarchical pores (i.e., mesopores) affected by the reaction temperature. The glucose conversion improved from 30% to 85% when the reaction temperature increased from 100°C to a maximum of 200°C for 3 h. Beyond the appropriate temperature of 140°C, decompositions of LA increased in the presence of HMF and formic acid (FA) (Antonyraj et al., 2014; Karwa et al., 2016; Liu et al., 2017; Piola et al., 2017; Wu et al., 2017); this explained the decrease in yield (Rackemann et al., 2014; Chen et al., 2018; Khan et al., 2018; Emel’yanenko et al., 2018). The results demonstrated that the type, quantity, and strength of the acid sites (Lewis acid), SBET, and hierarchical porous texture strongly influenced the catalytic activities during LA production. Further investigation revealed that the hybrid 1:1 catalyst afforded optimal catalytic activity with 62% LA yield at 160°C in 3 h.
Recently, the influence of the Sn/H-BEA catalyst on the glucose transformation of fructose and mannose was examined (Zhang et al., 2017). The conversion of the glucose substrate is generally enhanced by increasing the reaction temperature, and the maximum value of the conversion (up to 100%) may be attained because of the endothermic nature of the reaction (Saravanamurugan et al., 2013). The synthesis of glycosides was favored at higher reaction temperatures, whereas the production of fructose and methyl mannose declined markedly. The influence of temperature on the reaction performance can be attributed to the redistribution of the Sn species in the catalyst. A similar research was performed by Rajabbeigi et al. (2014), who studied the Sn/HBEA catalyst for the glucose upgrading isomerization of fructose at temperatures in the range 70–130°C and reached an analogous conclusion.
Further investigations could be performed involving the introduction of F−, P, and silane compounds to enhance the stability of zeolites (Jon et al., 2006; Louwen et al., 2020; Simancas et al., 2021). Additionally, research into extending the zeolite scope to contain natural materials with synthetic properties to accelerate the sustainability process should be considered.
Upgrading of Furan Derivatives Catalyzed by Non-Noble Metals
The high price of noble metal catalysts limits their industrial application, and some non-noble metals that have similar chemical properties and catalytic activity, including Fe, Co, Ni, or Cu, have been appraised for biomass valorization. Non-noble metal catalysts can reduce the cost of catalysts; however, they have the problem of metal leaching.
Cerium exhibited fair catalytic activity in the hydrogenation reaction. Feng et al. (2019) developed a (CePO4)0.16/Co2P catalyst that achieved an LA conversion of 98.2% and GVL yield of 97.1% at 90°C, 4.0 MPa H2 for 1.5 h. Furthermore, the catalyst maintained excellent performance in five successive runs, which confirmed that it had long-term catalytic activity and stability under strongly acidic conditions.
Non-noble materials have been used more frequently for the hydrogenation of furfural. Gong et al. (2018) investigated two N-doped carbon nanotube-encapsulated metal nanoparticles, denoted as Ni@NCNTs-600–800 and Co@NCNTs-600–800, which displayed superior catalytic activity for furfural hydrogenation. The production of furfuryl alcohol was as high as 100% at 80°C, and active performance was maintained for six cycles. The excellent yield of further hydrogenation compounds containing tetrahydrofurfuryl alcohol or cyclopentanone suggested that the catalyst has excellent catalytic potential at higher temperatures and pressures.
In addition, non-noble metal oxides (Mn, Fe, Co, Ni, etc.) (Jiang et al., 2016; You et al., 2016, 2017; Schade et al., 2018) have been reported to synthesize carbon 1,4-diacids (FDCA). A high FDCA yield of up to 90% and robust stability were observed over NiCo2O4 nanowires (Gao et al., 2018). Non-noble metals (Fe–Zr–O) were introduced as a catalyst in (Bmim)Cl to realize the conversion of fructose to HMF. HMF was further oxidized to FDCA, and a yield of 46.4% was obtained with full fructose conversion under one-pot reaction conditions. Similarly, a potassium ferrate (K2FeO4) catalyst was developed, and a comparable optimal FDCA yield (48.3%) was obtained under the optimized reaction conditions (Zhang et al., 2015).
Yu et al. (2015) explored a carbon nanotube-loaded bimetallic Ni–Fe [(Ni–Fe/CNT, carbon nanotube (CNT)] catalyst for the preparation of bis(hydroxymethyl)furan (BHMF) and DMF derived from the selective hydrogenation and hydrogenolysis of HMF. The results indicated that Ni-Fe/CNT with a Ni/Fe atomic ratio of 2:1 exhibited high catalytic activity (TON 48) with enhanced production of BHMF (96%) at 110°C (91% DMF at 200°C), which is higher than that of monometallic Fe/CNTs. Unlike monometallic Fe/CNT, monometallic Ni/CNT exhibited high transformation (TON 34.7) at 110°C with low selectivity for target hydrogenated molecules, BHMF (76% at 110°C) and DMF (46% at 200°C). The results demonstrated that a combination of Ni and Fe in Ni–Fe/CNT displayed benefits for selective C–O bond cleavage.
In addition, Sheng and Lobo (2016) investigated the performance of Fe in the catalytic activity of Cu/SiO2 for furfural-2-MF (2-methylfuran) conversion, where they revealed that the Fe chemical state in the bimetallic Fe–Cu was significant in determining the selectivity; the uniform dispersion of the Cu particles was the primary reason for the excellent catalytic activity. Notably, the partial reduction of the Fe species in the pre-reduced Cu–Fe/SiO2 catalysts altered the selectivity of the target molecule from furfuryl alcohol to 2-MF based on furfural. Furthermore, the Cu species exhibited weak affinity toward the C=C bond when compared with the C=O bond; thus, Cu-based metallic catalysts showed high selectivity for the preparation of furfuryl alcohol (Sitthisa et al., 2011).
In addition, Guo et al., 2018 investigated the catalytic performance of NiCo–B active centers supported on acid-activated attapulgite for the synthesis of furfuryl alcohol via furfural hydrogenation (Guo et al., 2017; Guo et al., 2018). Owing to the strong synergistic effect between the Ni and Co active centers, the NiCo–B exhibited strong interactions with the acid-activated attapulgite (H+-ATP) carrier via the electronic charge effect between the bimetallic NiCo–B amorphous alloy and H+-ATP support. The excellent catalytic performance can be ascribed to the role of ATP in the dispersion of NiCo–B particles, adjustment of the partial charge distribution, and the strong synergistic effect between Co and Ni.
Ionic Liquid-Catalyzed Conversion for Furan Derivatives
Ionic liquids (ILs) used in biomass valorization can be broadly divided into two categories, namely, 1) neutral ionic liquids and 2) functionalized ionic liquids. Neutral ionic liquids are normally used as solvents in biomass upgrading, whereas functionalized ionic liquids, generally including one or more acidic active groups, are used as solvents and catalysts. The meaningful conversion of C5 and C6 sugars to multipurpose green, renewable feedstocks, and fuel precursors illustrated the potential of ILs in biomass processing. Nevertheless, the efficient and convenient synthesis and recovery of ILs remain to be improved.
Acidic functionalized ILs catalyze the dehydration of carbohydrates to HMF and furfural in aqueous or alcohol media. The rehydration of HMF to afford LA or its derivatives is well known. Frequently used ILs, e.g., dimethylimidazolium hydrogensulfate (Kotadia and Soni, 2013; Eminov et al., 2014), 1-(4-butylsulfonic)-3-methylimidazolium hydrogensulfate (Matsagar et al., 2015; Wang et al., 2015), 1-(4-butylsulfonic)-3-allyl imidazolium hydrogensulfate, trifluoromethane sulfonate (Bao et al., 2008; Eminov et al., 2016), 1-carboxymethyl-3-methyl imidazolium chloride (Ma et al., 2015), and Cr3+ containing –SO3H functionalized polymeric BAILs (Li et al., 2013) have been extensively investigated as catalysts in the dehydration of monosaccharides to HMF under relatively mild conditions.
In addition to the dehydration of monosaccharides, ILs have been applied to the more complex multistep reactions of converting biomass to value-added platform molecules and feedstocks. For instance, a one-pot transformation of lignocellulosic and algal biomass into DMF was performed using a multicomponent catalytic system composed of N,N-dimethylacetamide methanesulfonate, Ru/C, and FA. (De et al., 2012).
LA or 4-oxopentanoic acid is a renewable generation platform molecule that can be obtained from C5 and C6 carbohydrates during dehydration and hydration reactions. This C5 keto-acid is generally used for the production of reproducible polymers and fuel precursors. The neutral and functionalized ILs acted as solvents or catalysts for the synthesis of LA and related derivatives (Tiong et al., 2018).
With 1-(3-propylsulfonic)-3-methylimidazolium chloride acting as the catalyst, a mixture of EL and LA was obtained from cellulose in aqueous ethanol via a one-pot successive process under mild conditions (Amarasekara and Wiredu, 2014). The optimal EL yield of 19.0% was achieved at 170°C, for 12 h, in a water–ethanol mixture medium containing 38.5% water. Furthermore, the highest LA yield (23.7%) was obtained for aqueous ethanol with a water content of 54% at 150°C for 48 h. The IL catalyst employed could be efficiently reused (96%) from the reaction medium (water) with negligible contamination.
ILs have also been employed as reaction media and catalysts for the conversion of biomass-derived derivatives. For instance, 90%–92% yield of succinic anhydride was obtained from HMF by oxidation with 30% H2O2 using 10 mol% 1-(alkylsulfonic)-3-methylimidazolium chloride acidic ILs as catalysts at 60°C for 14 h. The IL catalyst was recovered four times, with negligible loss in the catalytic performance. In addition, oxidation of furfural under similar reaction conditions afforded a mixture of three chemicals: 2(5H)-furanone, succinic anhydride, and maleic acid in 68%, 8%, and 12% yields, respectively (Amarasekara and Okorie, 2018).
Upgrading of Furan Derivatives in Sustainable Reaction Media
Considering that the single reaction phase systems required the separation of the prepared derivatives, an energy intensive process and afforded low selectivity values for the target molecule, the development of an alternative multiphasic medium was necessary (Figure 3).
Tetrahydrofuran (THF) has been widely employed as the extraction phase (EP) solvent. At the appropriate reaction temperature of 140°C, glucose converted almost completely using phosphates as catalysts; CrPO4 exhibited a significantly higher selectivity of 63% to HMF (Xu et al., 2016) than that of FePO4 (23%) (Yang et al., 2015). Meanwhile, the reaction catalyzed by Cr-β zeolite at 150°C achieved a slightly lower conversion rate of 87%, but an increased selectivity of 83% (Xu et al., 2019). Published reports indicate that NaCl has been extensively utilized as a modifier for the reaction phase (RP). For example, hafnyl phosphates (HPs) have demonstrated high selectivity of up to 91% under the optimal reaction conditions of 175°C and 150 min, and the concentration of NaCl in the solvent was 4 wt% (Cao et al., 2019).
Methyl tetrahydrofuran (MeTHF) was selected to investigate the influence of a biphasic medium on the dehydration of glucose at 175°C for 80 min using phosphate TiO2 as a catalyst. Comparing the biphasic system effect with the catalytic performance using MeTHF-N-methyl pyrrolidone (NMP) (v:v, 6:1), a notable enhancement of glucose conversion from 89.4% to 97.9% and HMF yield from 58.8% to 90.7% was observed (Atanda et al., 2016). The Atanda group explored using dioxane as EP, comparing the catalytic performance of other solvents containing THF, 1-BuOH, MIBK, and 1-propanol, all of which had a consistent NaCl content (20 wt%). With the hybrid catalysts composed of TiO2–ZrO2 and Amberlyst 70, almost full conversion of glucose and 86% product yield was achieved at 175°C for 3 h (Atanda et al., 2015).
In the interim, a combination of H2O-DMSO (3:1, w/w)/MIBK–1-BuOH (7:3, w/w) was employed in a reaction medium, in which a modified Cr(III)-containing polydivinylbenzene polymeric material with bifunctional Brønsted–Lewis acidity was utilized as the catalyst, affording 95% glucose conversion and 59% HMF yield (Wang et al., 2016). In addition, the same glucose–HMF reaction system was researched using zeolite β as a catalyst in the H2O–DMSO (9:1 v/v)/THF biphasic system at 180°C for 30 min, and a relatively high conversion of 80% and selectivity of 75% were obtained (Otomo et al., 2015).
In contrast to biphasic media, some researchers have concentrated on the use of deep eutectic solvents (DESs) as reaction media. An interesting composition was constructed using tetraalkyl ammonium chloride and alkylamine hydrochloride salts for fructose transformation (Figure 4). The salts were used as the catalyst, RP with NaHSO4 acted as the co-catalyst, and THF was utilized as the EP; these reaction conditions provided the maximum HMF yield of 83% at 120°C (Cao et al., 2011). Recently, a similar DES system was reported; it was composed of choline chloride (ChCl) as a hydrogen bond acceptor (HBA) and the reactant fructose as a hydrogen bond donor (HBD) (molar ratio 5:1), with acetonitrile as the EP. The results showed that complete conversion of fructose was achieved with 90% HMF yield using HCl as a catalyst after 4 h at 100°C (Zuo et al., 2017).
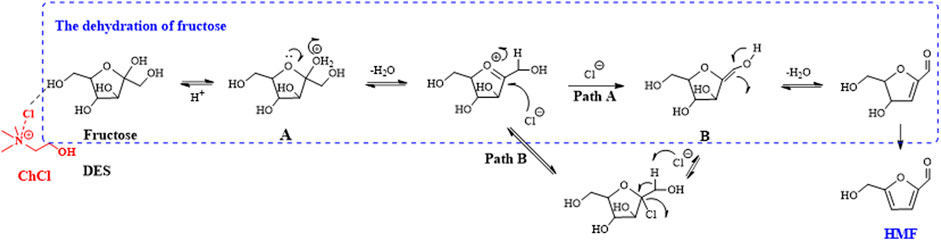
FIGURE 4. Possible synthesis mechanism of HMF from fructose in the deep eutectic solvents (DES)/acetonitrile biphasic system.
A biphasic reaction medium was also studied for polysaccharide conversion to HMF. For example, the mixed reaction media H2O/2-BuOH was utilized in situ for the inulin transformation with a catalyst dosage of 0.5 wt% of pretreated niobium pentoxide in the RP and gave a 54% yield in 140 min (Yang et al., 2011). The catalytic conversion reaction was also tested in the ChCl:oxalic acid DES reaction medium, simultaneously, with EtOAc as EP (v:v, 1:10), obtaining 64% conversion of inulin with complete selectivity for the synthesis of HMF (Hu et al., 2009). Based on the previously described work, the different species of RP and EP for the same reaction with inulin as a reactant, the optimal result with H2O–NMP (4:6, w/w)/MIBK, offered a 69% yield. In addition, sucrose was explored to produce an HMF-verifying solvent system, affording a maximum yield of 43%, although the conversion of sucrose was relatively low at only 58% (Chheda and Dumesic, 2007).
A more interesting alternative for the preparation of furans in EP–RP systems could be the production of CMF. The systematic research of the synthesis of CMF from microcrystalline cellulose, corn stover, straw, or birch wood was performed with an H2O/dichloroethane solvent pair modified with LiCl (Mascal and Nikitin, 2008; Mascal and Nikitin, 2009).
In addition, in situ extraction for the target chemical furfural was attempted with supercritical CO2, for which the conditions of 20 MPa, 150°C, and CO2 flow rate of 3.77 g min−1 were needed to achieve 88% conversion of xylose degradation and 52% yield with a 10-wt% dosage of Amberlyst 70 as a catalyst (Sato et al., 2019).
Summary and Outlook
Recent advances in the chem-catalytic valorization of biomass and derivatives into chemicals and fuels using green and sustainable approaches have been summarized. The heterogeneous solid catalysts, POM, zeolites, non-noble metals, and ILs are environmentally friendly materials. Additionally, the multiphasic extract affords efficient sustainable utilization of the reaction medium and catalyst and reduces resource requirements, which are needed for the separation of products.
Despite the aforementioned developments, there are still considerable challenges and opportunities for biomass upgrading using inexpensive, green, and efficient heterogeneous catalysts. First, leaching still exists, thus, compromising catalyst stability. Future efforts should be devoted to the investigation of substrate–catalyst interactions and the kinetics and thermodynamics of various catalytic materials. Second, a deeper understanding of the relationship between the catalytic performance and the chemical/electronic/structural properties of catalytic materials will be important for biomass valorization. Furthermore, the choice of the catalytic system is the most challenging problem, and determines catalyst reusability and the potential for industrial applications.
Author Contributions
XL and DY performed critical reviews and wrote the manuscript. HW and CL designed the structure of the manuscript and were responsible for this work. WY and QZ assisted XL and DY in preparing and completing the review. All authors discussed the results, wrote, and commented on the manuscript.
Funding
This work was financially supported by the Discipline and Master’s Site Construction Project of Guiyang University, Guiyang City Financial Support Guiyang University (2021-xk06), the Basic Research Program of Guizhou Province ((2019)1009), the Guizhou Provincial Key Laboratory for Rare Animal and Economic Insects of the Mountainous Region ((2018)5102), the National Natural Science Foundation of China (22065004), and the Guizhou Science and Technology Foundation ((2020)1Y054).
Conflict of Interest
The authors declare that the research was conducted in the absence of any commercial or financial relationships that could be construed as a potential conflict of interest.
Publisher’s Note
All claims expressed in this article are solely those of the authors and do not necessarily represent those of their affiliated organizations, or those of the publisher, the editors, and the reviewers. Any product that may be evaluated in this article, or claim that may be made by its manufacturer, is not guaranteed or endorsed by the publisher.
Abbreviations
DES, deep eutectic solvents; DMF, 2,5-dimethylfuran; EL, ethyl levulinate; EMF, 5-ethoxymethylfurfural; EP, extraction phase; FA, formic acid; FDCA, furan dicarboxylic acid; HBA, hydrogen bond acceptor; HBD, hydrogen bond donor; HMF, hydroxymethylfurfural; HP, hafnyl phosphates; HPA, heteropolyacid; HPM, phosphomolybdic acid hydrate; IL, ionic liquid; LA, levulinic acid; MeTHF, methyl tetrahydrofuran; MIBK, methyl isobutyl ketone; ML, methyl levulinate; POM, polyoxometalate; RP, reaction phase.
References
Amarasekara, A. S., and Okorie, N. C. (2018). 1-(Alkylsulfonic)-3-methylimidazolium Chloride Brönsted Acidic Ionic Liquid Catalyzed Hydrogen Peroxide Oxidations of Biomass Derived Furan Aldehydes. Catal. Commun. 108, 108–112. doi:10.1016/j.catcom.2018.01.032
Amarasekara, A. S., and Wiredu, B. (2014). Acidic Ionic Liquid Catalyzed One-Pot Conversion of Cellulose to Ethyl Levulinate and Levulinic Acid in Ethanol-Water Solvent System. Bioenerg. Res. 7, 1237–1243. doi:10.1007/s12155-014-9459-z
Antonyraj, C. A., Kim, B., Kim, Y., Shin, S., Lee, K.-Y., Kim, I., et al. (2014). Heterogeneous Selective Oxidation of 5-Hydroxymethyl-2-Furfural (HMF) into 2,5-diformylfuran Catalyzed by Vanadium Supported Activated Carbon in MIBK, Extracting Solvent for HMF. Catal. Commun. 57, 64–68. doi:10.1016/j.catcom.2014.08.008
Atanda, L., Konarova, M., Ma, Q., Mukundan, S., Shrotri, A., and Beltramini, J. (2016). High Yield Conversion of Cellulosic Biomass into 5-hydroxymethylfurfural and a Study of the Reaction Kinetics of Cellulose to HMF Conversion in a Biphasic System. Catal. Sci. Technol. 6, 6257–6266. doi:10.1039/C6CY00820H
Atanda, L., Silahua, A., Mukundan, S., Shrotri, A., Torres-Torres, G., and Beltramini, J. (2015). Catalytic Behaviour of TiO2-ZrO2 Binary Oxide Synthesized by Sol-Gel Process for Glucose Conversion to 5-hydroxymethylfurfural. RSC Adv. 5, 80346–80352. doi:10.1039/C5RA15739K
Bao, Q., Qiao, K., Tomida, D., and Yokoyama, C. (2008). Preparation of 5-hydroymethylfurfural by Dehydration of Fructose in the Presence of Acidic Ionic Liquid. Catal. Commun. 9, 1383–1388. doi:10.1016/j.catcom.2007.12.002
Bozell, J. J., and Petersen, G. R. (2010). Technology Development for the Production of Biobased Products from Biorefinery Carbohydrates-The US Department of Energy's "Top 10" Revisited. Green. Chem. 12, 539–554. doi:10.1039/B922014C
Bulushev, D. A., and Ross, J. R. H. (2018). Towards Sustainable Production of Formic Acid. ChemSusChem 11, 821–836. doi:10.1002/cssc.201702075
Cao, Q., Guo, X., Guan, J., Mu, X., and Zhang, D. (2011). A Process for Efficient Conversion of Fructose into 5-hydroxymethylfurfural in Ammonium Salts. Appl. Catal. A: Gen. 403, 98–103. doi:10.1016/j.apcata.2011.06.018
Cao, Z., Fan, Z., Chen, Y., Li, M., Shen, T., Zhu, C., et al. (2019). Efficient Preparation of 5-hydroxymethylfurfural from Cellulose in a Biphasic System over Hafnyl Phosphates. Appl. Catal. B: Environ. 244, 170–177. doi:10.1016/j.apcatb.2018.11.019
Chen, S. S., Wang, L., Yu, I. K. M., Tsang, D. C. W., Hunt, A. J., Jérôme, F., et al. (2018). Valorization of Lignocellulosic Fibres of Paper Waste into Levulinic Acid Using Solid and Aqueous Brønsted Acid. Bioresour. Tech. 247, 387–394. doi:10.1016/j.biortech.2017.09.110
Chheda, J. N., and Dumesic, J. A. (2007). An Overview of Dehydration, Aldol-Condensation and Hydrogenation Processes for Production of Liquid Alkanes from Biomass-Derived Carbohydrates. Catal. Today 123, 59–70. doi:10.1016/j.cattod.2006.12.006
Chheda, J. N., Román-Leshkov, Y., and Dumesic, J. A. (2007). Production of 5-hydroxymethylfurfural and Furfural by Dehydration of Biomass-Derived Mono- and Poly-Saccharides. Green. Chem. 9, 342–350. doi:10.1039/B611568C
Cirujano, F. G., Corma, A., and Llabrés i Xamena, F. X. (2015). Conversion of Levulinic Acid into Chemicals: Synthesis of Biomass Derived Levulinate Esters over Zr-Containing MOFs. Chem. Eng. Sci. 124, 52–60. doi:10.1016/j.ces.2014.09.047
De, S., Dutta, S., and Saha, B. (2012). One-pot Conversions of Lignocellulosic and Algal Biomass into Liquid Fuels. ChemSusChem 5, 1826–1833. doi:10.1002/cssc.201200031
Emel’yanenko, V. N., Altuntepe, E., Held, C., Pimerzin, A. A., and Verevkin, S. P. (2018). Renewable Platform Chemicals: Thermochemical Study of Levulinic Acid Esters. Thermochim. Acta 659, 213–221. doi:10.1016/j.tca.2017.12.006
Eminov, S., Brandt, A., Wilton-Ely, J. D. E. T., and Hallett, J. P. (2016). The Highly Selective and Near-Quantitative Conversion of Glucose to 5-hydroxymethylfurfural Using Ionic Liquids. PLoS One 11, e0163835. doi:10.1371/journal.pone.0163835
Eminov, S., Wilton-Ely, J. D. E. T., and Hallett, J. P. (2014). Highly Selective and Near-Quantitative Conversion of Fructose to 5-hydroxymethylfurfural Using Mildly Acidic Ionic Liquids. ACS Sust. Chem. Eng. 2, 978–981. doi:10.1021/sc400553q
Enferadi-Kerenkan, A., Do, T. O., and Kaliaguine, S. (2018). Heterogeneous Catalysis by Tungsten-Based Heteropoly Compounds. Catal. Sci. Technol. 8, 2257–2284. doi:10.1039/C8CY00281A
Feng, H.-J., Li, X.-C., Qian, H., Zhang, Y.-F., Zhang, D.-H., Zhao, D., et al. (2019). Efficient and Sustainable Hydrogenation of Levulinic-Acid to Gamma-Valerolactone in Aqueous Solution over Acid-Resistant CePO4/Co2P Catalysts. Green. Chem. 21, 1743–1756. doi:10.1039/C9GC00482C
Fernandes, D. R., Rocha, A. S., Mai, E. F., Mota, C. J. A., and Teixeira da Silva, V. (2012). Levulinic Acid Esterification with Ethanol to Ethyl Levulinate Production over Solid Acid Catalysts. Appl. Catal. A: Gen. 425-426, 199–204. doi:10.1016/j.apcata.2012.03.020
Gallezot, P. (2012). Conversion of Biomass to Selected Chemical Products. Chem. Soc. Rev. 41, 1538–1558. doi:10.1039/C1CS15147A
Gao, L., Bao, Y., Gan, S., Sun, Z., Song, Z., Han, D., et al. (2018). Hierarchical Nickel-Cobalt‐Based Transition Metal Oxide Catalysts for the Electrochemical Conversion of Biomass into Valuable Chemicals. ChemSusChem 11, 2547–2553. doi:10.1002/cssc.201800695
Gong, W., Chen, C., Zhang, H., Wang, G., and Zhao, H. (2018). Highly Dispersed Co and Ni Nanoparticles Encapsulated in N-Doped Carbon Nanotubes as Efficient Catalysts for the Reduction of Unsaturated Oxygen Compounds in Aqueous Phase. Catal. Sci. Technol. 8, 5506–5514. doi:10.1039/C8CY01488D
Guo, H., Zhang, H., Tang, W., Wang, C., Chen, P., Chen, X., et al. (2017). Furfural Hydrogenation over Amorphous alloy Catalysts Prepared by Different Reducing Agents. BioRes 12, 8755–8774. doi:10.15376/biores.12.4.8755-8774
Guo, H., Zhang, H., Zhang, L., Wang, C., Peng, F., Huang, Q., et al. (2018). Selective Hydrogenation of Furfural to Furfuryl Alcohol over Acid-Activated Attapulgite-Supported NiCoB Amorphous alloy Catalyst. Ind. Eng. Chem. Res. 57, 498–511. doi:10.1021/acs.iecr.7b03699
Hu, L., Lin, L., Wu, Z., Zhou, S., and Liu, S. (2015). Chemocatalytic Hydrolysis of Cellulose into Glucose over Solid Acid Catalysts. Appl. Catal. B: Environ. 174-175, 225–243. doi:10.1016/j.apcatb.2015.03.003
Hu, S., Zhang, Z., Zhou, Y., Song, J., Fan, H., and Han, B. (2009). Direct Conversion of Inulin to 5-hydroxymethylfurfural in Biorenewable Ionic Liquids. Green. Chem. 11, 873–877. doi:10.1039/B822328A
Hua, D., Li, P., Wu, Y., Chen, Y., Yang, M., Dang, J., et al. (2013). Preparation of Solid Acid Catalyst Packing AAO/SBA-15-SO3H and Application for Dehydration of Xylose to Furfural. J. Ind. Eng. Chem. 19, 1395–1399. doi:10.1016/j.jiec.2013.01.002
Jiang, C., Zhu, J., Wang, B., Li, L., and Zhong, H. (2018). One-pot Synthesis of 5-hydroxymethylfurfural from Glucose over Zirconium Doped Mesoporous KIT-6. Chin. J. Chem. Eng. 26, 1270–1277. doi:10.1016/j.cjche.2018.02.031
Jiang, N., You, B., Boonstra, R., Terrero Rodriguez, I. M., and Sun, Y. (2016). Integrating Electrocatalytic 5-hydroxymethylfurfural Oxidation and Hydrogen Production via Co-P-derived Electrocatalysts. ACS Energ. Lett. 1, 386–390. doi:10.1021/acsenergylett.6b00214
Jon, H., Lu, B., Oumi, Y., Itabashi, K., and Sano, T. (2006). Synthesis and thermal Stability of Beta Zeolite Using Ammonium Fluoride. Microporous Mesoporous Mater. 89, 88–95. doi:10.1016/j.micromeso.2005.10.016
Karwa, S., Gajiwala, V. M., Heltzel, J., Patil, S. K. R., and Lund, C. R. F. (2016). Reactivity of Levulinic Acid during Aqueous, Acid-Catalyzed HMF Hydration. Catal. Today 263, 16–21. doi:10.1016/j.cattod.2015.06.020
Khan, A. S., Man, Z., Bustam, M. A., Kait, C. F., Nasrullah, A., Ullah, Z., et al. (2018). Dicationic Ionic Liquids as Sustainable Approach for Direct Conversion of Cellulose to Levulinic Acid. J. Clean. Prod. 170, 591–600. doi:10.1016/j.jclepro.2017.09.103
Kotadia, D. A., and Soni, S. S. (2013). Symmetrical and Unsymmetrical Brønsted Acidic Ionic Liquids for the Effective Conversion of Fructose to 5-hydroxymethyl Furfural. Catal. Sci. Technol. 3, 469–474. doi:10.1039/C2CY20493B
Lai, F., Yan, F., Wang, Y., Li, C., Cai, J., and Zhang, Z. (2021). Tungstophosphoric Acid Supported on metal/Si-Pillared Montmorillonite for Conversion of Biomass-Derived Carbohydrates into Methyl Levulinate. J. Clean. Prod. 314, 128072. doi:10.1016/j.jclepro.2021.128072
Li, H., Fang, Z., Smith, R. L., and Yang, S. (2016). Efficient Valorization of Biomass to Biofuels with Bifunctional Solid Catalytic Materials. Prog. Energ. Combustion Sci. 55, 98–194. doi:10.1016/j.pecs.2016.04.004
Li, H., Zhang, Q., Liu, X., Chang, F., Zhang, Y., Xue, W., et al. (2013). Immobilizing Cr3+ with SO3H-Functionalized Solid Polymeric Ionic Liquids as Efficient and Reusable Catalysts for Selective Transformation of Carbohydrates into 5-hydroxymethylfurfural. Bioresour. Tech. 144, 21–27. doi:10.1016/j.biortech.2013.06.063
Liu, D., Gao, Z. Y., Wang, X. C., Zeng, J., and Li, Y. M. (2017). DFT Study of Hydrogen Production from Formic Acid Decomposition on Pd-Au alloy Nanoclusters. Appl. Surf. Sci. 426, 194–205. doi:10.1016/j.apsusc.2017.07.165
Liu, X., and Wang, R. (2018). Upgrading of Carbohydrates to the Biofuel Candidate 5-ethoxymethylfurfural (EMF). Int. J. Chem. Eng. 2018, 1–10. doi:10.1155/2018/2316939
Louwen, J. N., van Eijck, L., Vogt, C., and Vogt, E. T. C. (2020). Understanding the Activation of ZSM-5 by Phosphorus: Localizing Phosphate Groups in the Pores of Phosphate-Stabilized ZSM-5. Chem. Mater. 32 (21), 9390–9403. doi:10.1021/acs.chemmater.0c03411
Luan, Q. J., Liu, L. J., Gong, S. W., Lu, J., Wang, X., and Lv, D. M. (2018). Clean and Efficient Conversion of Renewable Levulinic Acid to Levulinate Esters Catalyzed by an Organic-Salt of H4SiW12O40. Process Saf. Environ. Prot. 117, 341–349. doi:10.1016/j.psep.2018.05.015
Lucas, N., Gurrala, L., and Athawale, A. (2019). Heteropolyacids Supported on Mesoporous Alsba-15 as Efficient Catalysts for Esterification of Levulinic Acid. J. Porous Mater. 26, 1335–1343. doi:10.1007/s10934-019-00734-w
Ma, Y., Qing, S., Wang, L., Islam, N., Guan, S., Gao, Z., et al. (2015). Production of 5-hydroxymethylfurfural from Fructose by a Thermo-Regulated and Recyclable Brønsted Acidic Ionic Liquid Catalyst. RSC Adv. 5, 47377–47383. doi:10.1039/C5RA08107F
Manikandan, K., and Cheralathan, K. K. (2017). Heteropoly Acid Supported on Silicalite-1 Possesing Intracrystalline Nanovoids Prepared Using Biomass—an Efficient and Recyclable Catalyst for Esterification of Levulinic Acid. Appl. Catal. A Gen. 547, 237–247. doi:10.1016/j.apcata.2017.09.007
Mascal, M., and Nikitin, E. B. (2008). Direct, High-Yield Conversion of Cellulose into Biofuel. Angew. Chem. Int. Ed. 47, 7924–7926. doi:10.1002/anie.200801594
Mascal, M., and Nikitin, E. B. (2009). Towards the Efficient, Total Glycan Utilization of Biomass. ChemSusChem 2, 423–426. doi:10.1002/cssc.200900071
Matsagar, B. M., Munshi, M. K., Kelkar, A. A., and Dhepe, P. L. (2015). Conversion of Concentrated Sugar Solutions into 5-hydroxymethyl Furfural and Furfural Using Brönsted Acidic Ionic Liquids. Catal. Sci. Technol. 5, 5086–5090. doi:10.1039/c5cy00858a
Moreno-Recio, M., Santamaría-González, J., and Maireles-Torres, P. (2016). Brönsted and Lewis Acid ZSM-5 Zeolites for the Catalytic Dehydration of Glucose into 5-hydroxymethylfurfural. Chem. Eng. J. 303, 22–30. doi:10.1016/j.cej.2016.05.120
Narkhede, N., Singh, S., and Patel, A. (2015). Recent Progress on Supported Polyoxometalates for Biodiesel Synthesis via Esterification and Transesterification. Green. Chem. 17, 89–107. doi:10.1039/c4gc01743a
Otomo, R., Yokoi, T., and Tatsumi, T. (2015). Osda-free Zeolite Beta with High Aluminum Content Efficiently Catalyzes a Tandem Reaction for Conversion of Glucose to 5-hydroxymethylfurfural. ChemCatChem 7, 4180–4187. doi:10.1002/cctc.201500837
Piola, L., Fernández-Salas, J. A., Nahra, F., Poater, A., Cavallo, L., and Nolan, S. P. (2017). Ruthenium-catalysed Decomposition of Formic Acid: Fuel Cell and Catalytic Applications. Mol. Catal. 440, 184–189. doi:10.1016/j.mcat.2017.06.021
Rackemann, D. W., Bartley, J. P., and Doherty, W. O. S. (2014). Methanesulfonic Acid-Catalyzed Conversion of Glucose and Xylose Mixtures to Levulinic Acid and Furfural. Ind. Crops Prod. 52, 46–57. doi:10.1016/j.indcrop.2013.10.026
Rajabbeigi, N., Torres, A. I., Lew, C. M., Elyassi, B., Ren, L., Wang, Z., et al. (2014). On the Kinetics of the Isomerization of Glucose to Fructose Using Sn-Beta. Chem. Eng. Sci. 116, 235–242. doi:10.1016/j.ces.2014.04.031
Ramli, N. A. S., Sivasubramaniam, D., and Amin, N. A. S. (2017). Esterification of Levulinic Acid Using ZrO-Supported Phosphotungstic Acid Catalyst for Ethyl Levulinate Production. Bioenerg. Res. 10, 1–12. doi:10.1007/s12155-017-9872-1
Saravanamurugan, S., Paniagua, M., Melero, J. A., and Riisager, A. (2013). Efficient Isomerization of Glucose to Fructose over Zeolites in Consecutive Reactions in Alcohol and Aqueous media. J. Am. Chem. Soc. 135, 5246–5249. doi:10.1021/ja400097f
Sato, O., Mimura, N., Masuda, Y., Shirai, M., and Yamaguchi, A. (2019). Effect of Extraction on Furfural Production by Solid Acid-Catalyzed Xylose Dehydration in Water. J. Supercrit. Fluids 144, 14–18. doi:10.1016/j.supflu.2018.10.004
Schade, O. R., Kalz, K. F., Neukum, D., Kleist, W., and Grunwaldt, J.-D. (2018). Supported Gold- and Silver-Based Catalysts for the Selective Aerobic Oxidation of 5-(hydroxymethyl)furfural to 2,5-furandicarboxylic Acid and 5-Hydroxymethyl-2-Furancarboxylic Acid. Green. Chem. 20, 3530–3541. doi:10.1039/c8gc01340c
Sheng, H., and Lobo, R. F. (2016). Iron-promotion of Silica-Supported Copper Catalysts for Furfural Hydrodeoxygenation. ChemCatChem 8, 3402–3408. doi:10.1002/cctc.201600540
Shi, X., Wu, Y., Yi, H., Rui, G., Li, P., Yang, M., et al. (2011). Selective Preparation of Furfural from Xylose over Sulfonic Acid Functionalized Mesoporous SBA-15 Materials. Energies 4, 669–684. doi:10.3390/en4040669
Simancas, R., Chokkalingam, A., Elangovan, S. P., Liu, Z. D., Sano, T., and Iyoki, K. (2021). Recent Progress in the Improvement of Hydrothermal Stability of Zeolites. Chem. Sci. 12, 7677–7695. doi:10.1039/D1SC01179K
Sitthisa, S., Sooknoi, T., Ma, Y., Balbuena, P. B., and Resasco, D. E. (2011). Kinetics and Mechanism of Hydrogenation of Furfural on Cu/SiO2 Catalysts. J. Catal. 277, 1–13. doi:10.1016/j.jcat.2010.10.005
Song, D., An, S., Sun, Y., and Guo, Y. (2016). Efficient Conversion of Levulinic Acid or Furfuryl Alcohol into Alkyl Levulinates Catalyzed by Heteropoly Acid and ZrO2 Bifunctionalized Organosilica Nanotubes. J. Catal. 333, 184–199. doi:10.1016/j.jcat.2015.10.018
Su, F., Wu, Q., Song, D., Zhang, X., Wang, M., and Guo, Y. (2013). Pore Morphology-Controlled Preparation of ZrO2-Based Hybrid Catalysts Functionalized by Both Organosilica Moieties and Keggin-type Heteropoly Acid for the Synthesis of Levulinate Esters. J. Mater. Chem. A. 1, 13209–13221. doi:10.1039/c3ta12412f
Sun, Z., Xue, L., Wang, S., Wang, X., and Shi, J. (2016). Single Step Conversion of Cellulose to Levulinic Acid Using Temperature-Responsive Dodeca-Aluminotungstic Acid Catalysts. Green. Chem. 18, 742–752. doi:10.1039/c5gc01730k
Tang, X., Wei, J., Ding, N., Sun, Y., Zeng, X., Hu, L., et al. (2017). Chemoselective Hydrogenation of Biomass Derived 5-hydroxymethylfurfural to Diols: Key Intermediates for Sustainable Chemicals, Materials and Fuels. Renew. Sust. Energ. Rev. 77, 287–296. doi:10.1016/j.rser.2017.04.013
Tiong, Y. W., Yap, C. L., Gan, S., and Yap, W. S. P. (2018). Conversion of Biomass and its Derivatives to Levulinic Acid and Levulinate Esters via Ionic Liquids. Ind. Eng. Chem. Res. 57, 4749–4766. doi:10.1021/acs.iecr.8b00273
Verma, P., Kuwahara, Y., Mori, K., Raja, R., and Yamashita, H. (2020). Functionalized Mesoporous SBA-15 Silica: Recent Trends and Catalytic Applications. Nanoscale 12, 11333–11363. doi:10.1039/d0nr00732c
Vilanculo, C. B., Leles, L. C. A, and Silva, M. J. (2018). H4SiW12O40-Catalyzed Levulinic Acid Esterification at Room Temperature for Production of Fuel Bioadditives. Waste Biomass Valor. 11, 1895–1904. doi:10.1007/s12649-018-00549-x
Wang, J., Xi, J., and Wang, Y. (2015). Recent Advances in the Catalytic Production of Glucose from Lignocellulosic Biomass. Green. Chem. 17, 737–751. doi:10.1039/c4gc02034k
Wang, M., Ma, J., Liu, H., Luo, N., Zhao, Z., and Wang, F. (2018). Sustainable Productions of Organic Acids and Their Derivatives from Biomass via Selective Oxidative Cleavage of C-C Bond. ACS Catal. 8, 2129–2165. doi:10.1021/acscatal.7b03790
Wang, P., Ren, L., and Yu, H. (2015). Production of 5-hydroxymethylfurfural from Glucose Catalyzed by Acidic Ionic Liquid. Energy Sourc. A: Recovery, Utilization, Environ. Effects. 37, 1729–1735. doi:10.1080/15567036.2011.634887
Wang, S.-S., and Yang, G.-Y. (2015). Recent Advances in Polyoxometalate-Catalyzed Reactions. Chem. Rev. 115, 4893–4962. doi:10.1021/cr500390v
Wang, X., Zhang, H., Ma, J., and Ma, Z.-H. (2016). Bifunctional Brønsted-Lewis Solid Acid as a Recyclable Catalyst for Conversion of Glucose to 5-hydroxymethylfurfural and its Hydrophobicity Effect. RSC Adv. 6, 43152–43158. doi:10.1039/c6ra03565e
Wang, Z., and Chen, Q. (2016). Conversion of 5-hydroxymethylfurfural into 5-ethoxymethylfurfural and Ethyl Levulinate Catalyzed by MOF-Based Heteropolyacid Materials. Green. Chem. 18, 5884–5889. doi:10.1039/c6gc01206j
Weinstock, I. A., Schreiber, R. E., and Neumann, R. (2018). Dioxygen in Polyoxometalate Mediated Reactions. Chem. Rev. 118, 2680–2717. doi:10.1021/acs.chemrev.7b00444
Wu, H., Huang, T., Cao, F., Zou, Q., Wei, P., and Ouyang, P. (2017). Co-production of HMF and Gluconic Acid from Sucrose by Chemo-Enzymatic Method. Chem. Eng. J. 327, 228–234. doi:10.1016/j.cej.2017.06.107
Wu, M., Zhao, Q.-Q., Li, J., Su, X.-L., Wu, H.-Y., Guan, X.-X., et al. (2016a). Tungstophosphoric Acid-Based Mesoporous Materials Anchored to MCM-41: Characterization and Catalytic Performance in Esterification of Levulinic Acid with Ethanol. J. Porous. Mater. 23, 1329–1338. doi:10.1007/s10934-016-0192-1
Wu, M., Zhang, X., Su, X., Li, X., Zheng, X., Guan, X., et al. (2016b). 3D Graphene Aerogel Anchored Tungstophosphoric Acid Catalysts: Characterization and Catalytic Performance for Levulinic Acid Esterification with Ethanol. Catal. Commun. 85, 66–69. doi:10.1016/j.catcom.2016.07.023
Wu, M., Zhao, Q.-Q., Li, J., Wu, H.-Y., Zheng, X.-C., Guan, X.-X., et al. (2016c). Esterification of Levulinic Acid into Hexyl Levulinate over Dodecatungstophosphoric Acid Anchored to Al-MCM-41. J. Exp. Nanoscience 11, 1331–1347. doi:10.1080/17458080.2016.1214985
Xu, S., Pan, D., Hu, F., Wu, Y., Wang, H., Chen, Y., et al. (2019). Highly Efficient Cr/β Zeolite Catalyst for Conversion of Carbohydrates into 5-hydroxymethylfurfural: Characterization and P-erformance. Fuel Process. Tech. 190, 38–46. doi:10.1016/j.fuproc.2019.03.012
Xu, S., Yan, X., Bu, Q., and Xia, H. (2016). Highly Efficient Conversion of Carbohydrates into 5-hydroxymethylfurfural Using the Bi-functional CrPO4 Catalyst. RSC Adv. 6, 8048–8052. doi:10.1039/c5ra23716e
Ya’aini, N., Amin, N. A. S., and Endud, S. (2013). Characterization and Performance of Hybrid Catalysts for Levulinic Acid Production from Glucose. Microporous Mesoporous Mater. 171, 14–23. doi:10.1016/j.micromeso.2013.01.002
Yan, K., Wu, G., Wen, J., and Chen, A. (2013). One-step Synthesis of Mesoporous H4SiW12O40-SiO2 Catalysts for the Production of Methyl and Ethyl Levulinate Biodiesel. Catal. Commun. 34, 58–63. doi:10.1016/j.catcom.2013.01.010
Yang, F., Liu, Q., Bai, X., and Du, Y. (2011). Conversion of Biomass into 5-hydroxymethylfurfural Using Solid Acid Catalyst. Bioresour. Tech. 102, 3424–3429. doi:10.1016/j.biortech.2010.10.023
Yang, L., Yan, X., Xu, S., Chen, H., Xia, H., and Zuo, S. (2015). One-pot Synthesis of 5-hydroxymethylfurfural from Carbohydrates Using an Inexpensive FePO4 Catalyst. RSC Adv. 5, 19900–19906. doi:10.1039/c4ra16145a
You, B., Jiang, N., Liu, X., and Sun, Y. (2016). Simultaneous H2Generation and Biomass Upgrading in Water by an Efficient Noble-Metal-Free Bifunctional Electrocatalyst. Angew. Chem. Int. Ed. 55, 9913–9917. doi:10.1002/anie.201603798
You, B., Liu, X., Liu, X., and Sun, Y. (2017). Efficient H2 Evolution Coupled with Oxidative Refining of Alcohols via A Hierarchically Porous Nickel Bifunctional Electrocatalyst. ACS Catal. 7, 4564–4570. doi:10.1021/acscatal.7b00876
Yu, L., He, L., Chen, J., Zheng, J., Ye, L., Lin, H., et al. (2015). Robust and Recyclable Nonprecious Bimetallic Nanoparticles on Carbon Nanotubes for the Hydrogenation and Hydrogenolysis of 5-hydroxymethylfurfural. ChemCatChem 7, 1701–1707. doi:10.1002/cctc.201500097
Zhang, G., Feng, P., Zhang, W., Liu, H., Wang, C., Ma, H., et al. (2017). Single Isomerization Selectivity of Glucose in Methanol over Sn-BEC Zeolite of Homogenous Sn Distribution. Microporous Mesoporous Mater. 247, 158–165. doi:10.1016/j.micromeso.2017.03.052
Zhang, J., Li, J., Tang, Y., Lin, L., Long, M., and Yang, F. (2015). Selective Conversion of Biomass-Derived Precursor 5-hydroxymethylfurfural to 2,5-furandicarboxylic Acid by Ferrate (VI) Oxidation. J. Biobased Mat Bioenergy 9, 502–508. doi:10.1166/jbmb.2015.1547
Zhang, X., Zhang, D., Sun, Z., Xue, L., Wang, X., and Jiang, Z. (2016). Highly Efficient Preparation of HMF from Cellulose Using Temperature-Responsive Heteropolyacid Catalysts in cascade Reaction. Appl. Catal. B: Environ. 196, 50–56. doi:10.1016/j.apcatb.2016.05.019
Zhang, Z., and Huber, G. W. (2018). Catalytic Oxidation of Carbohydrates into Organic Acids and Furan Chemicals. Chem. Soc. Rev. 47, 1351–1390. doi:10.1039/c7cs00213k
Zhao, S., Cheng, M., Li, J., Tian, J., and Wang, X. (2011). One Pot Production of 5-hydroxymethylfurfural with High Yield from Cellulose by a Brønsted-Lewis-surfactant-combined Heteropolyacid Catalyst. Chem. Commun. 47, 2176–2178. doi:10.1039/c0cc04444j
Zheng, X.-C., Li, N., Wu, M., Guan, X.-X., and Zhang, X.-L. (2017). Synthesis of Biofuel via Levulinic Acid Esterification over Porous Solid Acid Consisting of Tungstophosphoric Acid and Reduced Graphene Oxide. Res. Chem. Intermed. 43, 6651–6664. doi:10.1007/s11164-017-3012-6
Zhou, X., Li, Z. X., Zhang, C., Gao, X. P., Dai, Y. Z., and Wang, G. Y. (2016). Efficient Conversion of Renewable Levulinic Acid to N-Butyl Levulinate Catalyzed by Ammonium and Silver Co-doped Phosphotungstic Acid. J. Mol. Catal. A: Chem. 417, 71–75. doi:10.1016/j.molcata.2016.03.006
Keywords: polyoxometalates (POMs), zeolites, non-noble metal, ionic Liquids, furan derivatives
Citation: Liu X, Yu D, Yang W, Zhang Q, Wu H and Li C (2021) Development of Sustainable Catalytic Pathways for Furan Derivatives. Front. Chem. 9:707908. doi: 10.3389/fchem.2021.707908
Received: 10 May 2021; Accepted: 06 October 2021;
Published: 22 November 2021.
Edited by:
Naveen Kulkarni, Amrita Vishwa Vidyapeetham (Amritapuri Campus), IndiaReviewed by:
Putla Sudarsanam, National Chemical Laboratory (CSIR), IndiaQineng Xia, Jiaxing University, China
Yamuna R., Amrita Vishwa Vidyapeetham University, India
Copyright © 2021 Liu, Yu, Yang, Zhang, Wu and Li. This is an open-access article distributed under the terms of the Creative Commons Attribution License (CC BY). The use, distribution or reproduction in other forums is permitted, provided the original author(s) and the copyright owner(s) are credited and that the original publication in this journal is cited, in accordance with accepted academic practice. No use, distribution or reproduction is permitted which does not comply with these terms.
*Correspondence: Hongguo Wu, d2hnMDQwOEAxMjYuY29t; Can Li, bGljYW43OTAxMDhAMTYzLmNvbQ==
†These authors have contributed equally to this work and share first authorship