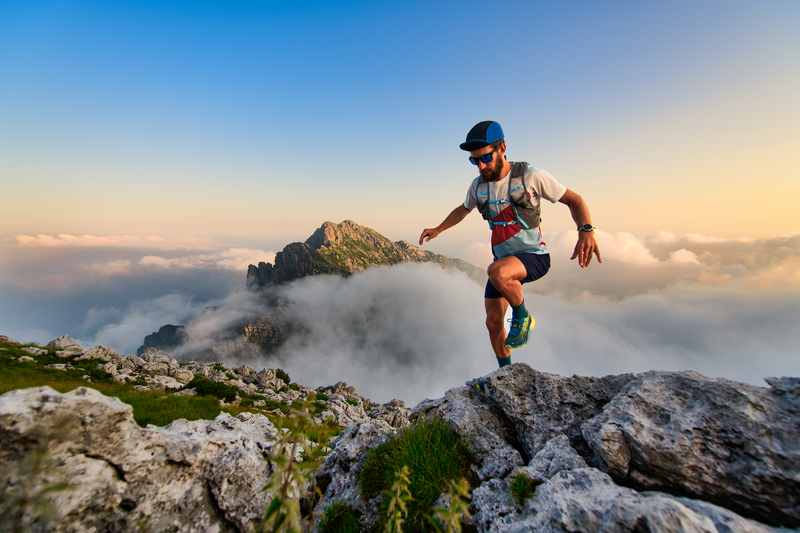
95% of researchers rate our articles as excellent or good
Learn more about the work of our research integrity team to safeguard the quality of each article we publish.
Find out more
ORIGINAL RESEARCH article
Front. Chem. , 01 July 2021
Sec. Solid State Chemistry
Volume 9 - 2021 | https://doi.org/10.3389/fchem.2021.706269
This article is part of the Research Topic Ordered and Disordered Cubic Systems: Pyrochlore to Fluorite, Now and the Horizon View all 12 articles
The structure of lead-technetium pyrochlore has been refined in space group
Technetium is unique amongst the transition metals in that no stable isotope exists. Technetium-99 is a major fission product of uranium-235 and is found in radioactive waste from nuclear fuel and, in certain jurisdictions, from the production of plutonium. Due to its long half-life (t1/2 = 2.1 × 105 y) and high mobility through geological formations, the migration of 99Tc is a significant challenge in nuclear waste management and it is a major contributor to the amount of radiation in the biosphere. Other isotopes of Tc are also of considerable importance, for example 99mTc is the most commonly employed isotope in nuclear medicine (Jurisson et al., 1993) and 95mTc is used as a radioisotope tracer (Conversi, 1985).
In comparison to the extensive literature on the nuclear medical applications of soluble technetium species, there is a dearth of information on the solid-state chemistry of Tc. Indeed, the work by Muller, White and Roy (Muller et al., 1964) in 1964 remains one of the most comprehensive reported studies in this area. Over the ensuring decades a small number of experimental studies have appeared including our studies of some Tc perovskites (Avdeev et al., 2011; Rodriguez et al., 2011; Thorogood et al., 2011a; Mravlje et al., 2012; Reynolds et al., 2017a), TcO2 (Rodriguez et al., 2007; Reynolds et al., 2017b; Childs et al., 2018), inverse spinel (Thorogood et al., 2011b) and ATcO4 scheelites (Kennedy et al., 2019) together with the study of some Bi-Tc oxides by Rodriguez and co-workers (Rodriguez et al., 2008) and lanthanoid pyrochlores by Hartman et al. (2011). Theoretical studies of the lanthanoid technetate pyrochlores have also been reported (Weck et al., 2010). The extraordinary magnetic properties of SrTcO3 and CaTcO3 have ignited interest in Tc oxides.
Rodriguez et al. have reported the synthesis and crystal structure of the bismuth technetium pyrochlore Bi2Tc2O7-d d ∼ 0.14 (Rodriguez et al., 2008). As observed for a number of other Bi pyrochlores, including Bi2Ru2O7-d (Avdeev et al., 2002), this structure is characterised by static disorder of the bismuth cations, an effect that is enhanced by the 6s (Conversi, 1985) lone pair electrons. Muller and co-workers (Muller et al., 1964) reported that the ternary lead-technetium oxide also forms a pyrochlore structure, which they suggested may be non-stoichiometric. Beyerlein and co-workers (Beyerlein et al., 1984) reported that the analogous lead-ruthenium pyrochlore is non-stoichiometric and displays oxygen vacancy ordering, the stoichiometry actually being Pb2Ru2O6.5 and the resulting space group being
Given the prevalence of oxygen non-stoichiometry in pyrochlores it is probable that, as suggested by Muller et al., Pb2Tc2O7-d will be non-stoichiometric, however it is unclear if oxygen vacancy ordering will occur. The aim of the present work was to determine the structure of the lead-technetium pyrochlore, and in particular to establish the nature of any non-stoichiometry. This has been accomplished using a combination of synchrotron X-ray and neutron powder diffraction methods. As we show here the target oxide is indeed non-stoichiometric, however we find no evidence for oxygen-vacancy ordering. X-ray absorption near-edge structure (XANES) at the Tc K-edge and Raman spectroscopy measurements are also reported.
Caution!99Tc is a β-emitter (Emax= 0.29 MeV). Appropriate shielding was employed during the synthesis and all manipulations. The polycrystalline sample of Pb2Tc2O7-d was prepared by the addition of 2.55 g of NH4TcO4 to 2.34 g of Pb(NO3)2, this mixture was then dry rolled in a polyethylene vial for 2 h to ensure complete mixing, the powder was then calcined in Ar for 1 h at 650°C. The Ar used had 12 ppm of O2, this percentage of O2 needs to be taken into account when calcining or sintering these samples as extended exposure to even small amounts of O2 will cause the Tc to oxidise and sample inhomogeneity will occur. The resultant calcine melted and so was ground in a mortar and pestle, wet ball milled in cyclohexane for 16 h and then tray dried. Note that milling in any other types of fluids may result in loss of Tc. Two one-gram pellets were pressed and sintered in Ar for 4 h at 800°C.
The synchrotron X-ray diffraction data were collected using the MYTHEN microstrip detector on the powder diffractometer at BL-10 of the Australian Synchrotron, Melbourne Australia (Wallwork et al., 2007). The sample (ca 1 mg) was loaded into a 0.3 mm diameter glass capillary inside a glovebox. The sealed capillary was rotated during the collection of the X-ray diffraction data. The data were recorded at room temperature in the angular range 5 < 2θ < 85°, using X-rays of wavelength 0.82523 Å as estimated using NIST LaB6. For high temperature measurements the sample was loaded into a 0.3 mm quartz capillary inside a glovebox and heated via a Cyberstar hot-air blower to up to 1,000°C. Neutron powder diffraction data were measured at room temperature using the high-resolution powder diffractometer Echidna at ANSTO’s OPAL facility at Lucas Heights (Avdeev and Hester, 2018). These measurements were taken at λ = 1.540 Å, with the sample ( ∼1 g) contained in a cylindrical vanadium can. The structure refinement used a combination of the synchrotron and neutron diffraction data sets using the program RIETICA (Liss et al., 2006).
X-ray absorption near-edge structure (XANES) spectra were collected from the sample as well as the (NH4)TcO4 and SrTcO3 standards at the Tc K-edge on beamline BL-12 (Glover et al., 2007) at the Australian Synchrotron in transmission mode using argon-filled ionization chambers (Blanchard et al., 2014). A total of 2 mg of each Tc-containing powder sample was first mixed with an appropriate amount of BN, and the mixture was then loaded into a 3.5-mm-diameter hole at the center of a 1-mm-thick aluminum plate. The samples were sealed using Kapton tapes on both sides of the aluminum plate. The energy calibration was carried out using the Mo K-edge at 20,000 eV, steps of 0.2 eV were used across the edge. The software package Athena was used for background subtraction and normalization (Ravel and Newville, 2005).
Electron diffraction patterns and microanalyses were obtained at ANSTO using a JEOL 2000FXII TEM operated at 200 kV and equipped with a Link ISIS ultra-thin window solid-state Si(Li) detector and microanalysis system. The TEM was calibrated for electron diffraction work over a range of objective lens settings using a polycrystalline gold standard. The sample was ground and loaded onto a copper grid for analysis.
Raman spectra were obtained using a Renishaw inVia Qontor confocal Raman microscope (Renishaw plc., Wotton-under-Edge, United Kingdom) with a 532 nm continuous wave, diode-pumped solid-state laser (Renishaw plc., Wotton-under-Edge, United Kingdom). The measurements were carried out with 0.1 mW of laser power on the sample and a x50/0.5NA long working distance objective, giving rise to a focused spot of approximately 1.3 µm diameter. The scattered light was analysed in backscattering geometry using holographic notch filters, 2,400 lines/mm grating and an air-cooled CCD detector. The spectra were collected at temperatures 298 K (25°C), 273 K (0°C) and 100 K (−173°C) using a FTIR600 variable-temperature stage (Linkam Scientific Instruments Ltd, Surrey, United Kingdom). Data collection was performed over a spectral range of 89–1,348 cm-1 with 100 accumulations/data set of 5 s exposure time per accumulation and an equilibration time of 10 min at each temperature. To avoid potential contamination of the equipment while maximizing temperature conductivity from the variable-temperature plate to the sample, the powder sample (ca 1 mg) was loaded into a 0.3 mm diameter quartz capillary inside a glovebox and this was sealed between two copper plates using thermal paste. An aperture in the top plate enabled the laser to reach the sample.
The structure of Pb2Tc2O7-d was initially refined as a cubic pyrochlore in space group
Previous high resolution structural studies of pyrochlores containing Bi cations on the A-site including Bi2Tc2O7-d (Rodriguez et al., 2008), have revealed static disorder of the Bi cations (Vanderah et al., 2005; Somphon et al., 2006). It should be noted that this is in contrast to the study of Ln2Tc2O7 compounds performed by Hartman and co-workers (Hartmann et al., 2011), however such disorder has also been seen in La2Zr2O7 (Tabira et al., 2001); although it is amplified by the presence of the Bi 6s2 lone pair electrons (Vanderah et al., 2005). Given that Pb2+ is isoelectronic with Bi3+, the possibility of static disorder of the Pb cations was then considered. Two models, where the Pb was displaced from the 16d site to either a 96g or 96h site, were explored. Both models resulted in a small improvement in the quality of the fit, however the data did not allow us to distinguish the best model between these. These two models are effectively equivalent, and we present here the results obtained with the Pb disordered on the 96h site. The possibility that the O(2) atom was also disordered on the 32e site was considered and the inclusion of such disorder resulted in a noticeable reduction in the displacement parameter for this atom to 0.71(23) Å2. There is a noticeable difference in the values of the Biso between the Pb and the Tc, this is due to the Biso for the 8-coordinate site in the pyrochlores being larger than that of the 6-coordinate site reflecting its more irregular environment. Interestingly the electron diffraction images of Pb2Re2O7-d published by Abakumov and co-workers (Abakumov et al., 1998) did not show any diffuse features characteristic of cation disorder suggesting there may be subtle differences between the Re and Tc oxides. Diffuse features have been observed in electron diffraction studies of numerous Bi containing pyrochlores including Bi2Ru2O7 (Goodwin et al., 2007).
The results of the refinements are summarised in Table 1 and are illustrated in Figure 1.
TABLE 1. Refined atomic coordinates and atomic displacement parameters for Pb2Tc2O7-d. These parameters were obtained by refinement against a combined neutron and synchrotron X-ray diffraction data set. a = 10.36581(2)Å RP = 2.50 RWP = 3.46% χ2 = 12.53. A comparably good fit could be obtained if the Pb was placed on the 96h site.
FIGURE 1. Observed, calculated and difference (A) neutron and (B) synchrotron X-ray diffraction profiles (second half of the pattern has a scale increase of 8.3 to allow the difference and observed profiles to align) for Pb2Tc2O7-d. The change in scale near 2θ = 45° in the SXRD pattern highlights both the quality of the data and fit.
The wide-spread prevalence of oxygen vacancies in pyrochlore oxides is rationalised by viewing the structure as based on two weakly interacting, but interpenetrating networks of formula Pb2O(2) and Tc2O(1)6 as illustrated in Figure 2.
FIGURE 2. Interconnecting network and the two separate sub lattices, Pb sites are shown in grey, Tc sites in purple, O(1) as fully coloured (red) spheres, and O(2) are red and white spheres, indicating the four-fold disorder of these.
The Pb cations in Pb2Tc2O7-d are in a compressed scalenohedral environment. The displacement of the Pb cations within the puckered hexagon of the PbO(1) group reduces two Pb-O(1) distances but increases the remaining four. The average Pb-O distance of 2.625(8) Å is not significantly changed from that seen in the ideal structure and is much longer than the two Pb-O(2) bonds along the
Selected area diffraction patterns (SAD) were indexed with the SingleCrystal™ software (CrystalMaker, 2021) via the coordinates obtained from X-ray and neutron diffraction analysis. The experimental patterns were in good agreement with the predicted patterns as shown in Figure 3, the zone axis for the experimental and simulated SAD patterns is [110]. An overlay of the simulated SAD patterns on the experimental one shows crosses for the forbidden reflections that are not shown in experimental image except {002} due to double diffraction. For the space group
FIGURE 3. SAD pattern with experimental data shown as white dots, red dots indicate the simulated pattern, reflections are marked, lattice absences are shown with a red cross and space group absences are shown with a red square.
The X-ray absorption spectrum of Pb2Tc2O7-d in the region of the Tc K-edge is dominated by a pronounced 1s - 5p dipole allowed transition at about 21,058 eV. This is illustrated in Figure 4, together with the spectra of SrTcO3 and (NH4)TcO4. It is immediately apparent from this figure that the energy of the Tc K-edge in Pb2Tc2O7-d is intermediate between that of the Tc4+ (SrTcO3) and Tc7+ ((NH4)TcO4) standards. This is consistent with formal valence of Tc being greater than four as indicated by the BVS calculations. Using the stoichiometry derived from the neutron diffraction measurement, Pb2Tc2O7-d, and assuming the lead remains divalent, Tc has a formal charge of +4.86.
FIGURE 4. Normalised X-ray absorption spectra of Pb2Tc2O7-d, SrTc4+O4 and (NH4)Tc7+O4. The Tc has six-fold coordination in Pb2Tc2O7-d and SrTcO3 and is in a tetrahedral environment in (NH4)TcO4.
The presence of the strong pre-edge feature in the spectrum of Pb2Tc2O7-d is somewhat unexpected. Such a feature is not apparent in the published spectra of Bi2Tc2O7-d (Rodriguez et al., 2008). The intensity of the pre-edge feature, seen in many K-edge spectra, is known to be sensitive to the site symmetry of the absorber. The transition may be assigned to a, formally dipole forbidden in centrosymmetric species, 1s → 4d transition. This transition is weakly quadrupole-allowed and gains intensity by mixing of the metal p-orbitals in a non-centrosymmetric absorber (Laplaza et al., 1996). This is clearly evident in the spectrum of tetrahedral (NH4)TcO4 where the 1s → 4d transition at 21047.7 eV is observed to be relatively intense. Studies of various Mo and Ru compounds demonstrate that well resolved pre-edge features can be observed for six-coordinate complexes where the symmetry is not strictly octahedral (Laplaza et al., 1996; Resseler et al., 2000; Sikora et al., 2007) and it is possible that this is the case here. An alternate possibility, that the sample has partially decomposed to produce a lower-symmetry material during the measurements, cannot be discounted. Irrespective of the origin of this feature in the Tc K-edge the XAS demonstrates the Tc oxidation state to be greater than 4+.
Raman spectra of the Pb2Tc2O7-δ sample were collected at 298 K (25°C), 273 K (0°C) and 100 K (−173°C). To correct for the Bose-Einstein occupation factor (Loudon, 1964), the raw data was divided by n(ω)+1, where n(ω) is the Bose-Einstein distribution given by:
Here,
where
The corrected intensities were normalized by the maximum value and fitted over the range of 100–1,000 cm-1 using a sum of Lorentzian functions. The obtained spectra at 298, 273, and 100 K are shown in Figure 5. The spectra comprise a large number of overlapping and convoluted Raman modes, and several Lorentzian peaks (>23 peaks) were needed in order to obtain a satisfactory fit of the data (see Supplementary Material for details of the fits). The most significant fitted bands (i.e., those describing clear, “sharp” peaks in the data) are shown in blue in Figure 5, whereas broader peaks describing background features are shown in light grey. The same general features are observed in the spectra measured at each of the three temperatures, although the observed sharpening of the peaks at lower temperatures emphasises the doublet nature of some peaks (see grey insert of Figure 5).
FIGURE 5. Normalized Raman spectra corrected for the Bose-Einstein population factor (black dots) and cumulative fit (red line) of a sum of Lorentzian functions (blue and grey) to the Raman spectra collected at 298 K (25°C), 273 K (0°C) and 100 K (−173°C). The broader Lorentzian peaks, which do not describe a defined peak but contribute to the description of the background are shown in grey, whereas peaks describing clearly visible peaks are shown in blue. An enhancement of the region between 825 and 1,000 cm-1 is given for each temperature, emphasizing the narrower nature of the peaks at lower temperatures.
The ideal pyrochlore structure in space group
The data unequivocally shows the presence of more than the six Raman bands expected for the ideal pyrochlore structure. In disordered structures, additional bands may emerge due to a change in local symmetry or a breakdown of the selection rules leading to silent and IR-active modes to appear in the Raman spectrum (Glerup et al., 2001). In the case of the disordered Pb2Tc2O7-d studied here, with the Pb and O(2) anions occupying the 96h and 32e Wyckoff positions, respectively, group theory predicts a total of 17 Raman-active modes, given by (Kroumova et al., 2003):
The number of predicted Raman-active modes agrees well with the number of significant peaks needed to obtain a satisfactory fit to the data (i.e., 17 significant fitted peaks in the 100 K Raman spectrum and 16 in the other two spectra), however, given the complex nature of the spectra we cannot confidently assign the fitted peaks to correspond to the aforementioned 17 Raman-active bands predicted for a disordered pyrochlore. Nevertheless, the data clearly shows the presence of several additional modes than that of the archetypical pyrochlore structure, corroborating the displacive disorder of the Pb and O(2) sites in Pb2Tc2O7-d. A similar observation was made by Arenas et al. (2010) in their Raman study of substituted bismuth pyrochlores, where displacive disorder gave rise to additional Raman bands.
The structure of lead-technetium pyrochlore has been refined using a combined synchrotron X-ray and neutron powder diffraction data set. The oxide is found to be oxygen deficient with a stoichiometry of Pb2Tc2O7-d with disordered oxygen vacancies. In this regards the structure is similar to Pb2Re2O7-d rather than to the analogous ruthenate, which displays ordering of the oxygen vacancies. Displacive disorder of the Pb cations and O(2) anions is observed, as evident from the refinements. X-ray absorption spectroscopic measurements at the Tc K-edge demonstrate the valence of the Tc is greater than 4+. Taking into account the BVS results from diffraction analysis, the most likely conclusion is that Tc is in a 5+ oxidation sate. Raman spectroscopy confirmed a change in the local structure and coordination of the ions compared to the ideal, non-disordered pyrochlore structure, supporting the displacive disorder present in Pb2Tc2O7-d.
The original contributions presented in the study are included in the article/Supplementary Material, further inquiries can be directed to the corresponding author.
BK, initial draft of manuscript, co conceived the research topic and performed synchrotron and neutron diffraction experiments and subsequent data analysis. TA, performed Raman data collection, manuscript production and literature review. MA, neutron diffraction and method of analyzing Technetium sample in neutron diffractometer. MC, sample synthesis. LL, data collection, manuscript production and literature review, MS, Raman data collection and analysis, manuscript production. KT, developed method for active Raman spectroscopy and literature review. JT, synchrotron data collection. KW, synchrotron data collection setup. ZZ, XANES data collection and analysis. HZ TEM data analysis. GT, project driver, final manuscript production, all scattering experiments, TEM data acquisition, Raman data collection.
The authors declare that the research was conducted in the absence of any commercial or financial relationships that could be construed as a potential conflict of interest.
The authors acknowledge the financial support from the Australian Government under SIA grant SHCC000002, the use of ACNS ANSTO facilities supported by NCRIS funding as well as Mike Jovanovic with assistance in the production of the samples.
The Supplementary Material for this article can be found online at: https://www.frontiersin.org/articles/10.3389/fchem.2021.706269/full#supplementary-material
Abakumov, A. M., Shpanchenko, R. V., Antipov, E. V., Kopnin, E. M., Capponi, J. J., Marezio, M., et al. (1998). Synthesis and Structural Study of Pb2Re2O7−xPyrochlores. J. Solid State. Chem. 138, 220–225. doi:10.1006/jssc.1998.7778
Arenas, D. J., Gasparov, V., Charles, H. P., and Tanner, D. B. (2010). Raman Study of Phonon Modes in Bismuth Pyrochlores. Phys. Rev. B. 82, 214302. doi:10.1103/physrevb.82.214302
CrystalMaker (2021). Generated Using SingleCrystalTM: A Single-crystal Diffraction Program for Mac and Windows. Oxford, England: CrystalMaker Software Ltd.
Avdeev, M., Haas, M. K., Jorgensen, J. D., and Cava, R. J. (2002). Static Disorder from Lone-Pair Electrons in Pyrochlores. J. Solid State. Chem. 169, 24–34. doi:10.1016/s0022-4596(02)00007-5
Avdeev, M., Thorogood, G. J., Carter, M. L., Kennedy, B. J., Ting, J., Singh, D. J., et al. (2011). Antiferromagnetism in a Technetium Oxide. Structure of Catco3. J. Am. Chem. Soc. 133, 1654–1657. doi:10.1021/ja109431t
Avdeev, M., and Hester, J. R. (2018). ECHIDNA: a Decade of High-Resolution Neutron Powder Diffraction at OPAL. J. Appl. Cryst. 51, 1597–1604. doi:10.1107/s1600576718014048
Beyerlein, R. A., Horowitz, H. S., Longo, J. M., Leonowicz, M. E., Jorgensen, J. D., and Rotella, F. J. (1984). Neutron Diffraction Investigation of Ordered Oxygen Vacancies in the Defect Pyrochlores, Pb2Ru2O6.5 and PbT1Nb2O6.5. J. Solid State. Chem. 51, 253–265. doi:10.1016/0022-4596(84)90341-4
Blanchard, P. E. R., Reynolds, E., Kennedy, B. J., Ling, C. D., Zhang, Z., Thorogood, G., et al. (2014). An Unconventional Method for Measuring the TcL3-Edge of Technetium Compounds. J. Synchrotron Radiat. 21, 1275–1281. doi:10.1107/s1600577514014891
Childs, B. C., Lawler, K. V., Braband, H., Mast, D. S., Bigler, L., Stalder, U., et al. (2018). The Nature of the Technetium Species Formed during the Oxidation of Technetium Dioxide with Oxygen and Water. Eur. J. Inorg. Chem. 2018, 1137–1144. doi:10.1002/ejic.201701199
Conversi, A. (1985). Uptake and Loss of Technetium-95m in the Crab Pachygrapsus Marmoratus. J. Environ. Radioactivity. 2, 161–170. doi:10.1016/0265-931x(85)90005-0
Facer, G., Elcombe, M., and Kennedy, B. (1993). Bismuth Ruthenium Oxides. Neutron Diffraction and Photoelectron Spectroscopic Study of Bi2Ru2O7 and Bi3Ru3O11. Aust. J. Chem. 46, 1897–1907. doi:10.1071/ch9931897
Glerup, M., Nielsen, O. F., and Poulsen, F. W. (2001). The Structural Transformation from the Pyrochlore Structure, A2B2O7, to the Fluorite Structure, AO2, Studied by Raman Spectroscopy and Defect Chemistry Modeling. J. Solid State. Chem. 160, 25–32. doi:10.1006/jssc.2000.9142
Glover, C., McKinlay, J., Clift, M., Barg, B., and Boldeman, J. (2007). Status of the X‐Ray Absorption Spectroscopy (XAS) Beamline at the Australian Synchrotron. AIP Conf. Proc. 882, 884–886. doi:10.1063/1.2644692
Goodwin, A. L., Withers, R. L., and Nguyen, H.-B. (2007). Real-space Refinement of Single-crystal Electron Diffuse Scattering and its Application to Bi2Ru2O7−δ. J. Phys. Condens. Matter 19, 335216. doi:10.1088/0953-8984/19/33/335216
Hartmann, T., Alaniz, A., Poineau, F., Weck, P. F., Valdez, J. A., Tang, M., et al. (2011). Structure Studies on Lanthanide Technetium Pyrochlores as Prospective Host Phases to Immobilize 99technetium and Fission Lanthanides from Effluents of Reprocessed Used Nuclear Fuels. J. Nucl. Mater. 411, 60–71. doi:10.1016/j.jnucmat.2011.01.033
Jurisson, S., Berning, D., Jia, W., and Ma, D. (1993). Coordination Compounds in Nuclear Medicine. Chem. Rev. 93, 1137–1156. doi:10.1021/cr00019a013
Kennedy, B. J., Injac, S., Thorogood, G. J., Brand, H. E. A., and Poineau, F. (2019). Structures and Phase Transitions in Pertechnetates. Inorg. Chem. 58, 10119–10128. doi:10.1021/acs.inorgchem.9b01257
Kennedy, B. J. (1996). Oxygen Vacancies in Pyrochlore Oxides: Powder Neutron Diffraction Study of Pb2Ir2O6.5and Bi2Ir2O7−y. J. Solid State. Chem. 123, 14–20. doi:10.1006/jssc.1996.0146
Kennedy, B. J., and Vogt, T. (1996). Structural and Bonding Trends in Ruthenium Pyrochlores. J. Solid State. Chem. 126, 261–270. doi:10.1006/jssc.1996.0337
Kroumova, E., Aroyo, M. I., Perez-Mato, J. M., Kirov, A., Capillas, C., Ivantchev, S., et al. (2003). Bilbao Crystallographic Server : Useful Databases and Tools for Phase-Transition Studies. Phase Transitions. 76, 155–170. doi:10.1080/0141159031000076110
Laplaza, C. E., Johnson, M. J. A., Peters, J. C., Odom, A. L., Kim, E., Cummins, C. C., et al. (1996). Dinitrogen Cleavage by Three-Coordinate Molybdenum(III) Complexes: Mechanistic and Structural Data1. J. Am. Chem. Soc. 118, 8623–8638. doi:10.1021/ja960574x
Liao, Y. (2006). Practical Electron Microscopy and Database. Available at https://www.globalsino.com/EM/page5001.html.
Liss, K.-D., Hunter, B., Hagen, M., Noakes, T., and Kennedy, S. (2006). Echidna-the New High-Resolution Powder Diffractometer Being Built at OPAL. Physica B: Condensed Matter. 385-386, 1010–1012. doi:10.1016/j.physb.2006.05.322
Loudon, R. (1964). The Raman Effect in Crystals. Adv. Phys. 13, 423–482. doi:10.1080/00018736400101051
McCauley, R. A. (1973). Infrared-absorption Characteristics of the Pyrochlore Structure*. J. Opt. Soc. Am. 63, 721–725. doi:10.1364/josa.63.000721
Mravlje, J., Aichhorn, M., and Georges, A. (2012). Origin of the High Néel Temperature in SrTc. Phys. Rev. Lett. 108, 197202. doi:10.1103/physrevlett.108.219903
Muller, O., White, W. B., and Roy, R. (1964). Crystal Chemistry of Some Technetium-Containing Oxides. J. Inorg. Nucl. Chem. 26, 2075–2086. doi:10.1016/0022-1902(64)80152-4
Ravel, B., and Newville, M. (2005). ATHENA,ARTEMIS,HEPHAESTUS: Data Analysis for X-ray Absorption Spectroscopy usingIFEFFIT. J. Synchrotron Radiat. 12, 537–541. doi:10.1107/s0909049505012719
Ressler, T., Timpe, O., Neisius, T., Find, J., Mestl, G., Dieterle, M., et al. (2000). Time-Resolved XAS Investigation of the Reduction/Oxidation of MoO3−x. J. Catal. 191, 75–85. doi:10.1006/jcat.1999.2772
Reynolds, E., Avdeev, M., Thorogood, G. J., Poineau, F., Czerwinski, K. R., Kimpton, J. A., et al. (2017). Structure and Magnetism in $\Mathrm{S}{\mathrm{r}}_{1\ensuremath{-}x}{A}_{x}\mathrm{Tc}{\Mathrm{O}}_{3}$ Perovskites: Importance of the $A$-site Cation. Phys. Rev. B. 95, 54430. doi:10.1063/1.2644692
Reynolds, E., Zhang, Z., Avdeev, M., Thorogood, G. J., Poineau, F., Czerwinski, K. R., et al. (2017). Thermal Expansion Behavior in TcO2. Toward Breaking the Tc-Tc Bond. Inorg. Chem. 56, 9219–9224. doi:10.1021/acs.inorgchem.7b01235
Rodriguez, E. E., Poineau, F., Llobet, A., Czerwinski, K., Seshadri, R., and Cheetham, A. K. (2008). Preparation and Crystal Structures of Bismuth Technetates: A New Metal Oxide System. Inorg. Chem. 47, 6281–6288. doi:10.1021/ic8003273
Rodriguez, E. E., Poineau, F., Llobet, A., Kennedy, B. J., and Avdeev, M. (2011). High Temperature Magnetic Ordering in the 4d Perovskite SrTcO3. Phys. Rev. Lett. 106, 67201. doi:10.1103/physrevlett.106.067201
Rodriguez, E. E., Poineau, F., Llobet, A., Sattelberger, A. P., Bhattacharjee, J., Waghmare, U. V., et al. (2007). Structural Studies of TcO2by Neutron Powder Diffraction and First-Principles Calculations. J. Am. Chem. Soc. 129, 10244–10248. doi:10.1021/ja0727363
Sikora, M., Oates, C. J., Szczerba, W., Kapusta, C., Zukrowski, J., Zajac, D., et al. (2007). XAS Study of Ru Doped N=1, 2 Ruddlesden-Popper Manganites. J. Alloys Compounds. 442, 265–267. doi:10.1016/j.jallcom.2006.06.115
Somphon, W., Ting, V., Liu, Y., Withers, R. L., Zhou, Q., and Kennedy, B. J. (2006). Local crystal Chemistry, Structured Diffuse Scattering and the Dielectric Properties of (Bi1−xYx)2(MIIINbV)O7 (M=Fe3+, In3+) Bi-pyrochlores. J. Solid State. Chem. 179, 2495–2505. doi:10.1016/j.jssc.2006.04.046
Subramanian, M. A., Aravamudan, G., and Subba Rao, G. V. (1983). Oxide Pyrochlores - A Review. Prog. Solid State. Chem. 15, 55–143. doi:10.1016/0079-6786(83)90001-8
Tabira, Y., Withers, R. L., Yamada, T., and Ishizawa, N. (2001). Annular Dynamical Disorder of the Rare Earth Ions in a La2Zr2O7 Pyrochlore via Single crystal Synchrotron X-ray Diffraction. Z. Krist. 216, 92–98. doi:10.1524/zkri.216.2.92.20338
Thorogood, G. J., Avdeev, M., Carter, M. L., Kennedy, B. J., Ting, J., and Wallwork, K. S. (2011). Structural Phase Transitions and Magnetic Order in SrTcO3. Dalton Trans. 40, 7228–7233. doi:10.1039/c1dt10445d
Thorogood, G. J., Zhang, Z., Hester, J. R., Kennedy, B. J., Ting, J., Glover, C. J., et al. (2011). Structure and Cation Ordering in Spinel-type TcCo2O4. An Example of a Trivalent Technetium Oxide. Dalton Trans. 40, 10924–10926. doi:10.1039/c1dt10954e
Vanderah, T. A., Levin, I., and Lufaso, M. W. (2005). An Unexpected crystal-chemical Principle for the Pyrochlore Structure. Eur. J. Inorg. Chem. 2005, 2895–2901. doi:10.1002/ejic.200500234
Wallwork, K. S., Kennedy, B. J., and Wang, D. (2007). The High Resolution Powder Diffraction Beamline for the Australian Synchrotron. AIP Conf. Proc. 879, 879–882.
Weck, P. F., Kim, E., Poineau, F., Rodriguez, E. E., Sattelberger, A. P., and Czerwinski, K. R. (2010). Structural and Electronic Trends in Rare-Earth Technetate Pyrochlores. Dalton Trans. 39, 7207–7210. doi:10.1039/c0dt00212g
Keywords: technetium, pyrochlore, disorder, crystallography, spectroscopy
Citation: Kennedy BJ, Ablott TA, Avdeev M, Carter ML, Losurdo L, Saura-Muzquiz M, Thorogood KJ, Ting J, Wallwork KS, Zhang Z, Zhu H and Thorogood GJ (2021) Synthesis and Structure of Oxygen Deficient Lead-Technetium Pyrochlore, the First Example of a Valence V Technetium Oxide. Front. Chem. 9:706269. doi: 10.3389/fchem.2021.706269
Received: 07 May 2021; Accepted: 08 June 2021;
Published: 01 July 2021.
Edited by:
Vladimir Dmitriev, European Synchrotron Radiation Facility, FranceReviewed by:
Jasper Plaisier, Elettra Sincrotrone Trieste, ItalyCopyright © 2021 Kennedy, Ablott, Avdeev, Carter, Losurdo, Saura-Muzquiz, Thorogood, Ting, Wallwork, Zhang, Zhu and Thorogood. This is an open-access article distributed under the terms of the Creative Commons Attribution License (CC BY). The use, distribution or reproduction in other forums is permitted, provided the original author(s) and the copyright owner(s) are credited and that the original publication in this journal is cited, in accordance with accepted academic practice. No use, distribution or reproduction is permitted which does not comply with these terms.
*Correspondence: Gordon J. Thorogood, Z2p0QGFuc3RvLmdvdi5hdQ==
Disclaimer: All claims expressed in this article are solely those of the authors and do not necessarily represent those of their affiliated organizations, or those of the publisher, the editors and the reviewers. Any product that may be evaluated in this article or claim that may be made by its manufacturer is not guaranteed or endorsed by the publisher.
Research integrity at Frontiers
Learn more about the work of our research integrity team to safeguard the quality of each article we publish.