- School of Chemical Engineering and Technology, Sun Yat-sen University, Zhuhai, China
Electrochemical water splitting involving hydrogen evolution reaction (HER) and oxygen evolution reaction (OER) is a greatly promising technology to generate sustainable and renewable energy resources, which relies on the exploration regarding the design of electrocatalysts with high efficiency, high stability, and low cost. Transition metal phosphides (TMPs), as nonprecious metallic electrocatalysts, have been extensively investigated and proved to be high-efficient electrocatalysts in both HER and OER. In this minireview, a general overview of recent progress in developing high-performance TMP electrocatalysts for electrochemical water splitting has been presented. Design strategies including composition engineering by element doping, hybridization, and tuning the molar ratio, structure engineering by porous structures, nanoarray structures, and amorphous structures, and surface/interface engineering by tuning surface wetting states, facet control, and novel substrate are summarized. Key scientific problems and prospective research directions are also briefly discussed.
Introduction
In the past decades, global energy consumption has been growing dramatically, with fossil fuels still providing over 80% of energy consumption, resulting in severe energy crisis and greenhouse effect (Zhang et al., 2017a; Peng et al., 2016). To address the key issue of these energy sources, researchers have begun to exploit clean and renewable energy resources such as solar energy, geothermal energy, wind power, and hydropower (Gallagher et al., 2015; Hou et al., 2011). Since hydrogen is regarded as a pollution-free energy source with ultrahigh energy density, water electrolysis has attracted tremendous attention for producing hydrogen energy from water in abundance (Peng et al., 2014; Ying et al., 2017; Yu et al., 2019a). As shown in Figure 1, electrochemical water splitting consists of two half-cell reactions, namely, hydrogen evolution reaction (HER) at the cathode and oxygen evolution reaction (OER) at the anode. The current state-of-the-art water electrolysis technology requires the use of precious metal (e.g., Pt and IrO2) electrocatalysts (Fujishima et al., 2008; Stoerzinger et al., 2015). Nevertheless, the high cost and low abundance of precious metals are restricting the widespread application of water electrolysis technology (Ying et al., 2014a; Ying et al., 2018a; Xiao et al., 2019; Xiao et al., 2021a).
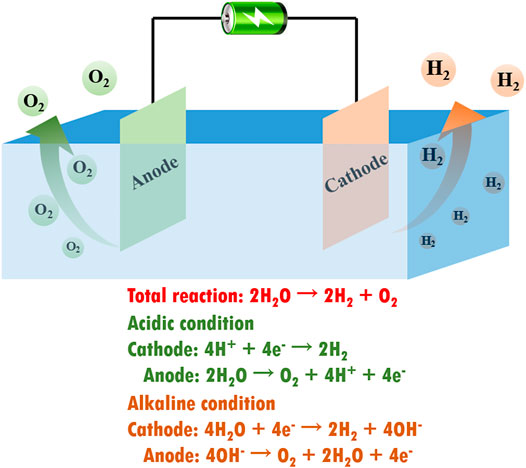
FIGURE 1. Schematic illustration of an electrolyzer for electrochemical water splitting and corresponding reaction equations in different reaction conditions.
Accordingly, tremendous substantial efforts have been devoted to the development of sustainable alternative electrocatalysts, one of which involves high efficiency, high stability, and low cost. Thus, many nonprecious metal electrocatalysts including carbon/carbon-based nanomaterials (Lu et al., 2015; Han et al., 2019; Ji et al., 2019), metal chalcogenides (Li et al., 2016), carbides (Yang et al., 2019; Lai et al., 2020), borides (Li et al., 2019), nitrides (Ye et al., 2018; Tareen et al., 2019), oxides (Song et al., 2018; Li et al., 2020), and especially phosphides (Ren et al., 2020; Sarkar et al., 2020) are currently representative electrocatalytic materials for both HER and OER. Among them, transition metal phosphides (TMPs) have been widely investigated and demonstrated to be very suitable for electrochemical water splitting (Dutta and Pradhan, 2017; Wang et al., 2018).
As the first nickel phosphide was prepared for vapor phase catalysis in the 1950s, it has been gradually depleted for a long time (Sweeny et al., 1958). In the 1990s, Kupka et al. first used metal phosphides as electrocatalysts (Kupka and Budniok, 1990). In 2005, Liu et al. presented the high HER activity of Ni2P(001) facet by density functional theory (DFT) calculations and suggested that the Ni-P bonds form a weak “ligand effect” that endows the fast dissociation of thiophene and hydrogen (Liu and Rodriguez, 2005). In 2013, Lewis et al. used nanostructured TMPs as HER electrocatalysts in acid media (Popczun et al., 2013). Until 2015, Yoo et al. made further progress demonstrating that the surface oxidized compounds are the true catalytic site of the metal phosphides (Ryu et al., 2015). Inspired by this work, massive research studies on TMPs for water electrolysis in the past several years have been reported. For example, Liu et al. reported an oxygen doping strategy to prepare an effective NiCoP electrocatalyst with optimized hydrogen adsorption energy and plentiful exposed active sites (Liu et al., 2018a).
Currently, the field of synthesis of TMP electrocatalysts for water splitting is experiencing a prosperous development with increasing achievements. It is necessary to timely provide a brief overview of this type of advanced material. In this minireview, we provide a general overview of the recent advances in efficient TMPs for electrochemical water splitting based on the understanding of their relationship between structure and performance. The developments in the design strategies based on composition engineering, structure engineering, and surface/interface engineering are summarized. Moreover, key scientific problems and prospective research directions are also proposed.
Composition Engineering
Considering the influence of electron structure and intermediate adsorption energy, the introduction of elements into TMPs, such as element doping, hybridization with other compositions, and tuning their molar ratios is often used to improve their electrocatalytic performance.
Element Doping
In general, doping foreign elements can boost the intrinsic activity of electrocatalysts (Niu et al., 2019; Song et al., 2021; Gao et al., 2019). What is more, the optimal ratio of doping elements can be predicted in advance via DFT calculations (Peng et al., 2021; Fu et al., 2019). Hence, the electrocatalytic behavior can be precisely regulated at the atomic scale. Most single metal phosphides have a limited intrinsic activity due to the difficulty in balancing the adsorption and desorption of reaction intermediates (Parra-Puerto et al., 2019; Read et al., 2016; Dutta et al., 2016). To solve the shortcomings, incorporating foreign atoms into the single TMPs has been studied by many research groups (Wu et al., 2018; Du et al., 2020; Yue et al., 2019; Shin et al., 2020; Lv et al., 2020). Liu et al. reported that doping element Zn into pristine CoP could significantly enhance HER performance (Liu et al., 2017). Because Zn had lower electron negativity as compared with Co, it could provide some electrons to nearby P atoms and generate some electron-deficient cations. As a result, the surrounding Co will lose more electrons, thus weakening H chemisorption strength with Co and improving HER performance (Figure 2A). Not only that many research studies have been achieved to explore the effects of introducing other elements such as N, S, or O into TMPs (Chang et al., 2018; Xi et al., 2018; Wang et al., 2019; Mu et al., 2021).
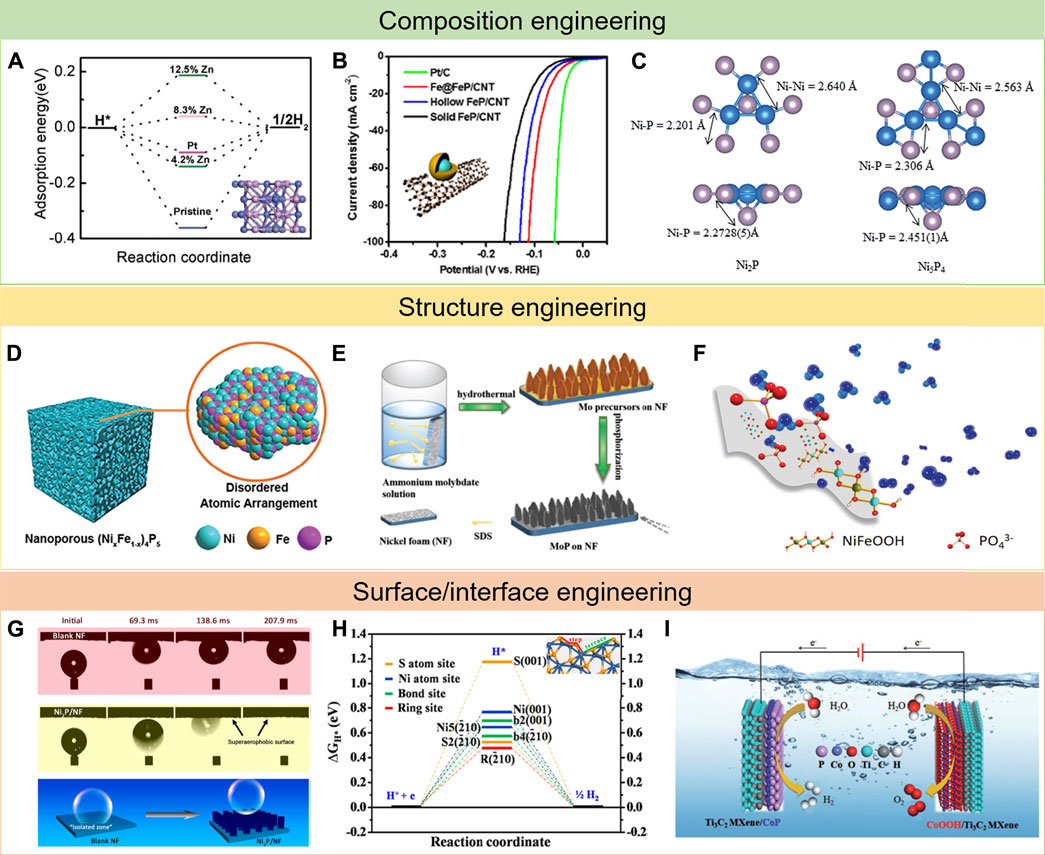
FIGURE 2. Typical examples of strategies for the development of TMP electrocatalysts: (A–C) composition engineering, reproduced with permission from ref. Liu et al. (2017), Li et al. (2017), and Laursen et al. (2015), respectively; (D–F) structure engineering, reproduced with permission from ref. Xu et al. (2018a), Jiang et al. (2018), and Hu et al. (2017), respectively; and (G–I) surface/interface engineering, reproduced with permission from ref.(Yu et al. (2019b), Feng et al. (2015), and Yan et al. (2020), respectively.
Hybridization
Hybridization with other compositions also remarkably increased catalytic activity because of the strong synergistic effect between multiple compositions and the improved mass transportation ability. In a multicomponent composite, the contacted components/phases show special interactions (Wang et al., 2018). By the rational design of the heterogeneous structure, the physicochemical properties of the interface can be obviously changed, thus presenting better performance than the single bulk phase (He et al., 2018; Saha et al., 2020). Li et al. developed a novel Fe@FeP core–shell nanoparticles on carbon nanotubes as an efficient HER catalyst (Li et al., 2017). The resulting Fe@FeP hybrid catalyst presented a low overpotential of 53 mV at a current density of 10 mA cm−2 and a Tafel slope of 55 mV dec−1 due to the presence of strong electron interactions and synergistic effects between Fe and FeP (Figure 2B). DFT calculations showed that the hydrogen adsorption energy on Fe@FeP is very close to that on Pt (111), revealing the enhancement effect of the Fe@FeP core–shell structure. In addition, Yu et al. synthesized a FeP/Ni2P hybrid catalyst via the chemical vapor deposition method (Yu et al., 2018). The obtained hybrid catalyst showed excellent catalytic performance in HER because it preferentially exposed the most active facets compared to the FeP(001) crystal, which contributed to the high activity not observed in typical FeP crystals.
Tuning the Molar Ratio of M/P
Since the first use of TMPs in electrocatalysis, enormous efforts have been devoted to pursuing the optimal metal/phosphorus (M/P) molar ratios (Callejas et al., 2015). As studied before, different M/P molar ratios lead to variations in crystal structures, hence directly influencing the electrocatalytic properties (Pei et al., 2017). In some situations, tuning molar ratios of M/P could boost excellent enhancements on HER activity. For example, Laursen et al. have explored the superior HER activity of Ni5P4 nanoparticles by researching the different catalytic performances between Ni2P and Ni5P4 nanoparticles (Laursen et al., 2015). The combination of the Volmer–Tafel mechanism and DFT calculation disclosed that Ni5P4 displayed a faster reaction rate because of the increased binding energy of the first hydrogenic intermediate, which in turn increases the second proton affinity. Moreover, the bond length of Ni-P in Ni4P5 is also longer than that in Ni2P (Figure 2C), explaining that Ni4P5 exhibited much better catalytic activity compared to Ni2P.
Structure Engineering
Besides the composition engineering mentioned above, structure engineering is also an indispensable strategy to improve the water splitting performance of electrocatalysts. Three main aspects including porous structures, nanoarray structures, and amorphous structures are discussed in this section.
Porous Structures
It is well known that the electrocatalytic reactions proceed on the surface of electrocatalysts; tiny pore structure could lead to improving the surface area and exposing more active sites (Peng et al., 2018; Xiao et al., 2021b; Ying et al., 2018b; Ying et al., 2014b). Since Erlebacher’s group put forward a continuum model to explain the fundamental mechanism of nanoporosity formation in the dealloying process (Erlebacher et al., 2001), many scientists have continuously continued to explore this. In this regard, Xu et al. developed a facile technique to prepare a nanoporous (NixFe1-x)4P5 with controllable metal ratio (Figure 2D), which serves as a bifunctional catalyst for both HER and OER with an outstanding catalyst performance (Xu et al., 2018a). Sun et al. designed porous and multishelled Ni2P hollow spheres by using the carbon spheres as the template, subsequently phosphating along with thermal treatment (Sun et al., 2017). The hollow porous multishells and nanosized subunits endow Ni2P with short charge transport distances, abundant active sites, and high stability against agglomeration, representing outstanding OER catalytic performance.
Nanoarray Structures
The nanoarray-structured self-supported electrodes, growing on the free-standing substrates, with discontinuous phase contact areas are very attractive due to avoiding the negative effects of binders and generating excellent stability (Liang et al., 2016; Zhang et al., 2020; Hou et al., 2020). In contrast to swarming bubbles adsorbed onto the planar surface of bulk materials, these nanoarrays can always show very preeminent electrocatalytic activity. For example, Shen et al. reported a porous MoP nanoflake array grown on nickel foam (MoP/NF) with excellent performance in water electrolysis (Figure 2E) (Jiang et al., 2018). In contrast to MoP, the enhanced electrocatalytic performance of MoP/NF is ascribed to the reduced size and the special nanoflake array structure. Moreover, besides avoiding the use of binders, the porous MoP/NF could be directly treated as both current collector and electrocatalyst, which made the active sites fully exposed and conductive to gas penetration and mass transfer. For another example, Su et al. successfully prepared self-supported NiMoP2 with grain boundary rich nanowire architecture on a carbon cloth substrate, showing small overpotential and remarkable electrochemical durability for water splitting (Wang et al., 2017). Such impressive characteristics can be tracked to the hierarchical architecture of the NiMoP2 nanowire in situ grown on a 3D carbon cloth substrate weakening the disintegration tendency of catalyst, and the special grain boundary nanowire structure of NiMoP2 provides the maximum number of electroactive surface/active sites.
Amorphous Structures
Since vacancies and defects are often considered as active sites of catalysis, amorphous catalysts are widely researched because of the disordered domains containing lots of vacancies and defects (Yan et al., 2017; Chu et al., 2020). In general, amorphous structures are always rigorous to preparation conditions (Zhang et al., 2016; Anantharaj et al., 2017; Ray et al., 2017). For instance, Xiong et al. reported a high-efficiency OER electrocatalyst based on bulk amorphous NiFeP in both alkaline and acidic electrolytes (Hu et al., 2017). In this case, the element P with proper electronegativity has been utilized to stabilize Ni and Fe atoms as an amorphous metallic phase with high conductivity and may also form active species for enhancing OER performance. Besides, the metallic bonds facilitated the electron transfer, whereas the P atoms supplied suitable bonds with reaction intermediates (Figure 2F). At the same time, the coordination numbers of Ni and Fe in Ni40Fe40P20 were largely lower than those counterparts, indicating metal atoms in the Ni40Fe40P20 were unsaturated. Combining these features together, this Ni40Fe40P20 electrode manifested an outstanding performance in OER. Therefore, the amorphous NiFeP accomplishes unprecedentedly excellent OER performance.
Surface/Interface Engineering
Surface/interface engineering, including the tuning surface wetting states, facet control, and novel substrate, is another effective way to enhance the performance of electrocatalysts. Since both mass transfer and gas delivery play a crucial role during water splitting, modifying the surface wettability such as superhydrophilicity is beneficial to HER and OER. In addition, the facet control and novel substrate are also beneficial to electrocatalytic properties via exposing more catalytic active sites and facilitating the electrolyte transfer.
Tuning Surface Wetting States
During the electrolysis of water, the surface wettability such as superhydrophilicity and superaerophobicity of the electrocatalysts are key to the electrocatalytic process due to the evolution of gas bubbles in the solution (Hao et al., 2016; Tian et al., 2020). It is commonly thought that superhydrophilicity is advantageous for mass transfer inside the electrode and can improve the interaction between electrocatalysts and electrolytes (Kim et al., 2021; Riyajuddin et al., 2021). Likewise, the superaerophobicity can be beneficial to electrocatalysts by avoiding bubble effects (Sheng et al., 2020). The formed gas bubbles would adhere to the surface of the electrocatalyst during the water splitting process, which would be a barrier to the solution diffusing to the active sites and result in a dissatisfied performance (Xu et al., 2018b). Precise surface design can improve the surface’s superhydrophilicity and superaerophobicity to boost the interaction between electrodes and electrolytes and also solve the bubble adsorption issue during the electrocatalytic process. For example, Yu et al. prepare a Ni2P nanoarray catalyst with a unique superaerophobic surface feature grown on a Ni foam substrate, which represents remarkable electrocatalytic activity and stability in basic media (Yu et al., 2019b). The special superaerophobicity endows an outstanding capability to withstand inside and outside forces and releases the in situ–formed H2 bubbles timely (Figure 2G), resulting in highly efficient electrocatalytic activity and outstanding stability of the Ni2P/NF.
Facet Control
While researching the composition of a nanostructure to TMPs that display reasonable electrocatalytic performance during water splitting progress, a large portion of research studies have focused on enhancing the intrinsic activity of active sites on the surface of nanostructures (Dutta et al., 2016; Lei et al., 2018; Ying et al., 2018c). Hence, there have been enormous efforts to prepare facet-controlled TMP nanostructures restricted by special facets which exert excellent catalytic activity (Liu and Rodriguez, 2005; Popczun et al., 2013). For example, Zhang et al. reported the ultrathin CoP nanosheets with dominant active facets by a chemical transformation method (Zhang et al., 2017b). The as-prepared samples consist of porous nanosheets, which are in single crystalline structure with a preferential [100] orientation. DFT calculation disclosed that on CoP (Lv et al., 2021) facets, a near-zero Gibbs free energy could lead to high utilization efficiency of active sites, therefore endowing (Lv et al., 2021) highly active CoP. In addition to single crystalline, other crystallines are, of course, affected by the crystal surface. For instance, Feng et al. reported novel {2—10} facet-exposed Ni3S2 nanosheet arrays on nickel foam (NF) (Figure 2H), which can efficiently catalyze both HER and OER (Feng et al., 2015). The excellent electrocatalytic performance in water splitting can be attributed mainly to the synergistic effect between the nanosheet array architecture and exposed {2—10} high-index facets.
Novel Substrate
Until now, two novel substrates with good conductivity, high mechanical strength, and corrosion resistance in electrocatalysis fields have been developed, which can act as supporting materials for stabilizing electrocatalysts and facilitate the electron transfer from the external circuit to electrocatalysts (Liu et al., 2018b; Gao et al., 2021; Yu et al., 2020; Xiu et al., 2018). One of them is black phosphorus (BP), a kind of 2D material, which is well known for its intriguing physicochemical properties, such as high charge–carrier mobility, tunable bandgap, and highly anisotropic characters (Fei and Yang, 2014; Jiang and Park, 2014; Liu et al., 2014). In view of these merits of BP, Wu et al. designed novel Ni3N-Ni2P-BP heterostructure nanosheets with enhanced OER activity in alkaline conditions (Wu et al., 2019). The DFT calculation demonstrates that Ni2P is very close to the ideal value compared to the Gibbs free energies of Ni3N and BP. In addition, the electron transfer rate can be improved by the metallic nature of the Ni3N-Ni2P-BP catalyst. As a result, the heterostructure of Ni3N-Ni2P-BP catalysts can remarkably enhance the OER activity. As another promising 2D material, transition metal carbides and nitrides (known as MXenes) with the features of great mechanical stability, high conductivity, and wide chemical variety display great potential as substrates (Du et al., 2018; Yoon et al., 2019; Lv et al., 2021). For example, Yan et al. successfully prepared novel CoP nanosheet arrays on the surface of Ti3C2 MXene nanosheets (CoP/Ti3C2 MXene) (Figure 2I) (Yan et al., 2020). Benefiting from the unique structure and synergistic effect between the active CoP and Ti3C2 MXene nanosheets, the 3D MXene matrix not only prevents the self-aggregation of active sites but also significantly facilitates the electrolyte accessibility and enhances the charge/mass transfer. Hence, the CoP/Ti3C2 MXene heterostructure represents a largely improved electrocatalytic activity and remarkable stability in HER over a wide pH range.
Conclusion and Outlook
This review has summarized the reported strategies for developing TMP electrocatalysts in electrochemical water splitting, including composition engineering, structure engineering, and surface/interface engineering. These strategies can be utilized as common and efficient strategies for preparing high-performance electrocatalysts. Moreover, the strategies discussed above can be modified and/or extended to other systems although we only focused on a limited number of examples.
Although rapid and significant development has been made in the synthesis of TMP electrocatalysts with superior HER and/or OER performance, the research in this field is still at the exploration stage, and several issues need to be addressed, such as preparation of TMPs with a special facet, stabilization of TMPs in acidic OER, and surface oxidation of TMPs. To better understand the in-depth reason for the enhanced electrocatalytic performance, two research aspects are recommended: 1) in situ structural characterization for investigating the catalytic active sites and 2) theoretical reaction simulation for predicting the optimized structures/compositions. Combining these two aspects, the field of TMP electrocatalysts for water splitting will undoubtedly keep moving forward rapidly.
Author Contributions
JY was in charge of organization and writing of this manuscript. HW contributed to the search of related literature studies.
Funding
This work was supported by the Guangdong Basic and Applied Basic Research Foundation (2019A1515110436), the Guangzhou Science and Technology Project (202102020463), the Fundamental Research Funds for the Central Universities (2021qntd13), and the Hundred Talents Project that was supported by Sun Yat-sen University, China.
Conflict of Interest
The authors declare that the research was conducted in the absence of any commercial or financial relationships that could be construed as a potential conflict of interest.
Publisher’s Note
All claims expressed in this article are solely those of the authors and do not necessarily represent those of their affiliated organizations, or those of the publisher, the editors and the reviewers. Any product that may be evaluated in this article, or claim that may be made by its manufacturer, is not guaranteed or endorsed by the publisher.
References
Anantharaj, S., Reddy, P. N., and Kundu, S. (2017). Core-Oxidized Amorphous Cobalt Phosphide Nanostructures: An Advanced and Highly Efficient Oxygen Evolution Catalyst. Inorg. Chem. 56 (3), 1742–1756. doi:10.1021/acs.inorgchem.6b02929
Callejas, J. F., Read, C. G., Popczun, E. J., McEnaney, J. M., and Schaak, R. E. (2015). Nanostructured Co2P Electrocatalyst for the Hydrogen Evolution Reaction and Direct Comparison with Morphologically Equivalent CoP. Chem. Mater. 27 (10), 3769–3774. doi:10.1021/acs.chemmater.5b01284
Chang, J., Li, K., Wu, Z., Ge, J., Liu, C., and Xing, W. (2018). Sulfur-Doped Nickel Phosphide Nanoplates Arrays: A Monolithic Electrocatalyst for Efficient Hydrogen Evolution Reactions. ACS Appl. Mater. Inter. 10 (31), 26303–26311. doi:10.1021/acsami.8b08068
Chu, W., Shi, Z., Hou, Y., Ma, D., Bai, X., Gao, Y., et al. (2020). Trifunctional of Phosphorus-Doped NiCo2O4 Nanowire Materials for Asymmetric Supercapacitor, Oxygen Evolution Reaction, and Hydrogen Evolution Reaction. ACS Appl. Mater. Inter. 12 (2), 2763–2772. doi:10.1021/acsami.9b13182
Du, C.-F., Dinh, K. N., Liang, Q., Zheng, Y., Luo, Y., Zhang, J., et al. (2018). Self-Assemble and In Situ Formation of Ni1−xFexPS3Nanomosaic-Decorated MXene Hybrids for Overall Water Splitting. Adv. Energ. Mater. 8 (26), 1801127. doi:10.1002/aenm.201801127
Du, Y., Ding, X., Han, M., and Zhu, M. (2020). Morphology and Composition Regulation of FeCoNi Prussian Blue Analogues to Advance in the Catalytic Performances of the Derivative Ternary Transition‐Metal Phosphides for OER. ChemCatChem 12 (17), 4339–4345. doi:10.1002/cctc.202000466
Dutta, A., and Pradhan, N. (2017). Developments of Metal Phosphides as Efficient OER Precatalysts. J. Phys. Chem. Lett. 8 (1), 144–152. doi:10.1021/acs.jpclett.6b02249
Dutta, A., Samantara, A. K., Dutta, S. K., Jena, B. K., and Pradhan, N. (2016). Surface-Oxidized Dicobalt Phosphide Nanoneedles as a Nonprecious, Durable, and Efficient OER Catalyst. ACS Energ. Lett. 1 (1), 169–174. doi:10.1021/acsenergylett.6b00144
Erlebacher, J., Aziz, M. J., Karma, A., Dimitrov, N., and Sieradzki, K. (2001). Evolution of Nanoporosity in Dealloying. Nature 410 (6827), 450–453. doi:10.1038/35068529
Fei, R., and Yang, L. (2014). Strain-engineering the Anisotropic Electrical Conductance of Few-Layer Black Phosphorus. Nano Lett. 14 (5), 2884–2889. doi:10.1021/nl500935z
Feng, L.-L., Yu, G., Wu, Y., Li, G.-D., Li, H., Sun, Y., et al. (2015). High-Index Faceted Ni3S2 Nanosheet Arrays as Highly Active and Ultrastable Electrocatalysts for Water Splitting. J. Am. Chem. Soc. 137 (44), 14023–14026. doi:10.1021/jacs.5b08186
Fu, G., Wen, X., Xi, S., Chen, Z., Li, W., Zhang, J.-Y., et al. (2019). Tuning the Electronic Structure of NiO via Li Doping for the Fast Oxygen Evolution Reaction. Chem. Mater. 31 (2), 419–428. doi:10.1021/acs.chemmater.8b03776
Fujishima, A., Zhang, X., and Tryk, D. (2008). TiO2 Photocatalysis and Related Surface Phenomena. Surf. Sci. Rep. 63 (12), 515–582. doi:10.1016/j.surfrep.2008.10.001
Gallagher, J., Styles, D., McNabola, A., and Williams, A. P. (2015). Current and Future Environmental Balance of Small-Scale Run-Of-River Hydropower. Environ. Sci. Technol. 49 (10), 6344–6351. doi:10.1021/acs.est.5b00716
Gao, K., Wang, B., Tao, L., Cunning, B. V., Zhang, Z., Wang, S., et al. (2019). Efficient Metal‐Free Electrocatalysts from N‐Doped Carbon Nanomaterials: Mono‐Doping and Co‐Doping. Adv. Mater. 31 (13), 1805121. doi:10.1002/adma.201805121
Gao, L., Bao, W., Kuklin, A. V., Mei, S., Zhang, H., and Ågren, H. (2021). Hetero‐MXenes: Theory, Synthesis, and Emerging Applications. Adv. Mater. 33 (10), 2004129. doi:10.1002/adma.202004129
Han, X., Ling, X., Yu, D., Xie, D., Li, L., Peng, S., et al. (2019). Atomically Dispersed Binary Co-Ni Sites in Nitrogen-Doped Hollow Carbon Nanocubes for Reversible Oxygen Reduction and Evolution. Adv. Mater. 31 (49), 1905622. doi:10.1002/adma.201905622
Hao, J., Yang, W., Huang, Z., and Zhang, C. (2016). Superhydrophilic and Superaerophobic Copper Phosphide Microsheets for Efficient Electrocatalytic Hydrogen and Oxygen Evolution. Adv. Mater. Inter. 3 (16), 1600236. doi:10.1002/admi.201600236
He, L., Cui, B., Hu, B., Liu, J., Tian, K., Wang, M., et al. (2018). Mesoporous Nanostructured CoFe-Se-P Composite Derived from a Prussian Blue Analogue as a Superior Electrocatalyst for Efficient Overall Water Splitting. ACS Appl. Energ. Mater. 1 (8), 3915–3928. doi:10.1021/acsaem.8b00663
Hou, Y., Vidu, R., and Stroeve, P. (2011). Solar Energy Storage Methods. Ind. Eng. Chem. Res. 50 (15), 8954–8964. doi:10.1021/ie2003413
Hou, C. C., Zou, L., Wang, Y., and Xu, Q. (2020). MOF‐Mediated Fabrication of a Porous 3D Superstructure of Carbon Nanosheets Decorated with Ultrafine Cobalt Phosphide Nanoparticles for Efficient Electrocatalysis and Zinc-Air Batteries. Angew. Chem. Int. Ed. 59 (48), 21360–21366. doi:10.1002/anie.202011347
Hu, F., Zhu, S., Chen, S., Li, Y., Ma, L., Wu, T., et al. (2017). Amorphous Metallic NiFeP: A Conductive Bulk Material Achieving High Activity for Oxygen Evolution Reaction in Both Alkaline and Acidic Media. Adv. Mater. 29 (32), 1606570. doi:10.1002/adma.201606570
Ji, D., Fan, L., Li, L., Peng, S., Yu, D., Song, J., et al. (2019). Atomically Transition Metals on Self‐Supported Porous Carbon Flake Arrays as Binder‐Free Air Cathode for Wearable Zinc−Air Batteries. Adv. Mater. 31 (16), 1808267. doi:10.1002/adma.201808267
Jiang, J.-W., and Park, H. S. (2014). Negative Poisson's Ratio in Single-Layer Black Phosphorus. Nat. Commun. 5 (1), 4727. doi:10.1038/ncomms5727
Jiang, Y., Lu, Y., Lin, J., Wang, X., and Shen, Z. (2018). A Hierarchical MoP Nanoflake Array Supported on Ni Foam: A Bifunctional Electrocatalyst for Overall Water Splitting. Small Methods 2, 1700369. doi:10.1002/smtd.201700369
Kim, B. K., Kim, M. J., and Kim, J. J. (2021). Impact of Surface Hydrophilicity on Electrochemical Water Splitting. ACS Appl. Mater. Inter. 13 (10), 11940–11947. doi:10.1021/acsami.0c22409
Kupka, J., and Budniok, A. (1990). Electrolytic Oxygen Evolution on Ni-Co-P Alloys. J. Appl. Electrochem. 20 (6), 1015–1020. doi:10.1007/BF01019582
Lai, X., Guo, R., Cui, C., Ren, E., Xiao, H., Qin, Q., et al. (2020). Co-N-Codoped Carbon/Co@Carbon Cloth Hybrid Derived from ZIF-67 for the Oxygen Evolution Reaction and Supercapacitors. Energy Fuels 34 (10), 13023–13031. doi:10.1021/acs.energyfuels.0c00814
Laursen, A. B., Patraju, K. R., Whitaker, M. J., Retuerto, M., Sarkar, T., Yao, N., et al. (2015). Nanocrystalline Ni5P4: A Hydrogen Evolution Electrocatalyst of Exceptional Efficiency in Both Alkaline and Acidic media. Energy Environ. Sci. 8, 1027–1034. doi:10.1039/c4ee02940b
Lei, W., Deng, Y.-P., Li, G., Cano, Z. P., Wang, X., Luo, D., et al. (2018). Two-Dimensional Phosphorus-Doped Carbon Nanosheets with Tunable Porosity for Oxygen Reactions in Zinc-Air Batteries. ACS Catal. 8 (3), 2464–2472. doi:10.1021/acscatal.7b02739
Li, H., Tsai, C., Koh, A. L., Cai, L., Contryman, A. W., Fragapane, A. H., et al. (2016). Activating and Optimizing MoS2 Basal Planes for Hydrogen Evolution through the Formation of Strained sulphur Vacancies. Nat. Mater 15 (1), 48–53. doi:10.1038/nmat4465
Li, X., Liu, W., Zhang, M., Zhong, Y., Weng, Z., Mi, Y., et al. (2017). Strong Metal-Phosphide Interactions in Core-Shell Geometry for Enhanced Electrocatalysis. Nano Lett. 17 (3), 2057–2063. doi:10.1021/acs.nanolett.7b00126
Li, Y., Huang, B., Sun, Y., Luo, M., Yang, Y., Qin, Y., et al. (2019). Multimetal Borides Nanochains as Efficient Electrocatalysts for Overall Water Splitting. Small 15 (1), 1804212. doi:10.1002/smll.201804212
Li, J., Chu, D., Dong, H., Baker, D. R., and Jiang, R. (2020). Boosted Oxygen Evolution Reactivity by Igniting Double Exchange Interaction in Spinel Oxides. J. Am. Chem. Soc. 142 (1), 50–54. doi:10.1021/jacs.9b10882
Liang, H., Gandi, A. N., Anjum, D. H., Wang, X., Schwingenschlögl, U., and Alshareef, H. N. (2016). Plasma-Assisted Synthesis of NiCoP for Efficient Overall Water Splitting. Nano Lett. 16 (12), 7718–7725. doi:10.1021/acs.nanolett.6b03803
Liu, P., and Rodriguez, J. A. (2005). Catalysts for Hydrogen Evolution from the [NiFe] Hydrogenase to the Ni2P(001) Surface: The Importance of Ensemble Effect. J. Am. Chem. Soc. 127 (42), 14871–14878. doi:10.1021/ja0540019
Liu, H., Neal, A. T., Zhu, Z., Luo, Z., Xu, X., Tománek, D., et al. (2014). Phosphorene: An Unexplored 2D Semiconductor with a High Hole Mobility. ACS Nano 8 (4), 4033–4041. doi:10.1021/nn501226z
Liu, T., Liu, D., Qu, F., Wang, D., Zhang, L., Ge, R., et al. (2017). Enhanced Electrocatalysis for Energy-Efficient Hydrogen Production over CoP Catalyst with Nonelectroactive Zn as a Promoter. Adv. Energ. Mater. 7 (15), 1700020. doi:10.1002/aenm.201700020
Liu, C., Zhang, G., Yu, L., Qu, J., and Liu, H. (2018). Oxygen Doping to Optimize Atomic Hydrogen Binding Energy on NiCoP for Highly Efficient Hydrogen Evolution. Small 14 (22), 1800421. doi:10.1002/smll.201800421
Liu, H., Hu, K., Yan, D., Chen, R., Zou, Y., Liu, H., et al. (2018). Recent Advances on Black Phosphorus for Energy Storage, Catalysis, and Sensor Applications. Adv. Mater. 30 (32), 1800295. doi:10.1002/adma.201800295
Lu, X., Yim, W.-L., Suryanto, B. H. R., and Zhao, C. (2015). Electrocatalytic Oxygen Evolution at Surface-Oxidized Multiwall Carbon Nanotubes. J. Am. Chem. Soc. 137 (8), 2901–2907. doi:10.1021/ja509879r
Lv, X., Li, X., Yang, C., Ding, X., Zhang, Y., Zheng, Y. Z., et al. (2020). Large‐Size, Porous, Ultrathin NiCoP Nanosheets for Efficient Electro/Photocatalytic Water Splitting. Adv. Funct. Mater. 30, 1910830. doi:10.1002/adfm.201910830
Lv, Z., Wang, M., Liu, D., Jian, K., Zhang, R., and Dang, J. (2021). Synergetic Effect of Ni2P and MXene Enhances Catalytic Activity in the Hydrogen Evolution Reaction. Inorg. Chem. 60 (3), 1604–1611. doi:10.1021/acs.inorgchem.0c03072
Mu, J., Xu, J., Zhou, C., Wang, Q., Wang, X. G., Liu, Z. Y., et al. (2021). Self‐Supported Oxygen and Molybdenum Dual‐Doped Cobalt Phosphide Hierarchical Nanomaterials as Superior Bifunctional Electrocatalysts for Overall Water Splitting. ChemElectroChem 8 (1), 103–111. doi:10.1002/celc.202001105
Niu, S., Jiang, W.-J., Wei, Z., Tang, T., Ma, J., Hu, J.-S., et al. (2019). Se-Doping Activates FeOOH for Cost-Effective and Efficient Electrochemical Water Oxidation. J. Am. Chem. Soc. 141 (17), 7005–7013. doi:10.1021/jacs.9b01214
Parra-Puerto, A., Ng, K. L., Fahy, K., Goode, A. E., Ryan, M. P., and Kucernak, A. (2019). Supported Transition Metal Phosphides: Activity Survey for HER, ORR, OER, and Corrosion Resistance in Acid and Alkaline Electrolytes. ACS Catal. 9 (12), 11515–11529. doi:10.1021/acscatal.9b03359
Pei, Y., Yang, Y., Zhang, F., Dong, P., Baines, R., Ge, Y., et al. (2017). Controlled Electrodeposition Synthesis of Co-Ni-P Film as a Flexible and Inexpensive Electrode for Efficient Overall Water Splitting. ACS Appl. Mater. Inter. 9 (37), 31887–31896. doi:10.1021/acsami.7b09282
Peng, S., Li, L., Han, X., Sun, W., Srinivasan, M., Mhaisalkar, S. G., et al. (2014). Cobalt Sulfide Nanosheet/Graphene/Carbon Nanotube Nanocomposites as Flexible Electrodes for Hydrogen Evolution. Angew. Chem. Int. Ed. 53 (46), 12594–12599. doi:10.1002/anie.201408876
Peng, S., Jin, G., Li, L., Li, K., Srinivasan, M., Ramakrishna, S., et al. (2016). Multi-functional Electrospun Nanofibres for Advances in Tissue Regeneration, Energy Conversion & Storage, and Water Treatment. Chem. Soc. Rev. 45, 1225–1241. doi:10.1039/C5CS00777A
Peng, S., Gong, F., Li, L., Yu, D., Ji, D., Zhang, T., et al. (2018). Necklace-like Multishelled Hollow Spinel Oxides with Oxygen Vacancies for Efficient Water Electrolysis. J. Am. Chem. Soc. 140 (42), 13644–13653. doi:10.1021/jacs.8b05134
Peng, Y., Hajiyani, H., and Pentcheva, R. (2021). Influence of Fe and Ni Doping on the OER Performance at the Co3O4(001) Surface: Insights from DFT+U Calculations. ACS Catal. 11, 5601–5613. doi:10.1021/acscatal.1c00214
Popczun, E. J., Mckone, J. R., Read, C. G., Biacchi, A. J., Wiltrout, A. M., Lewis, N. S., et al. (2013). Nanostructured Nickel Phosphide as an Electrocatalyst for the Hydrogen Evolution Reaction. J. Am. Chem. Soc. 135 (25), 9267–9270. doi:10.1021/ja403440e
Ray, C., Lee, S. C., Sankar, K. V., Jin, B., Lee, J., Park, J. H., et al. (2017). Amorphous Phosphorus-Incorporated Cobalt Molybdenum Sulfide on Carbon Cloth: An Efficient and Stable Electrocatalyst for Enhanced Overall Water Splitting over Entire pH Values. ACS Appl. Mater. Inter. 9 (43), 37739–37749. doi:10.1021/acsami.7b11192
Read, C. G., Callejas, J. F., Holder, C. F., and Schaak, R. E. (2016). General Strategy for the Synthesis of Transition Metal Phosphide Films for Electrocatalytic Hydrogen and Oxygen Evolution. ACS Appl. Mater. Inter. 8 (20), 12798–12803. doi:10.1021/acsami.6b02352
Ren, D., Ying, J., Xiao, M., Deng, Y. P., Ou, J., Zhu, J., et al. (2020). Hierarchically Porous Multimetal‐Based Carbon Nanorod Hybrid as an Efficient Oxygen Catalyst for Rechargeable Zinc-Air Batteries. Adv. Funct. Mater. 30 (7), 1908167. doi:10.1002/adfm.201908167
Riyajuddin, S., Azmi, K., Pahuja, M., Kumar, S., Maruyama, T., Bera, C., et al. (2021). Super-Hydrophilic Hierarchical Ni-Foam-Graphene-Carbon Nanotubes-Ni2P-CuP2 Nano-Architecture as Efficient Electrocatalyst for Overall Water Splitting. ACS Nano 15 (3), 5586–5599. doi:10.1021/acsnano.1c00647
Ryu, J., Jung, N., Jang, J. H., Kim, H.-J., and Yoo, S. J. (2015). In Situ Transformation of Hydrogen-Evolving CoP Nanoparticles: Toward Efficient Oxygen Evolution Catalysts Bearing Dispersed Morphologies with Co-oxo/hydroxo Molecular Units. ACS Catal. 5 (7), 4066–4074. doi:10.1021/acscatal.5b00349
Saha, J., Verma, S., Ball, R., Subramaniam, C., and Murugavel, R. (2020). Compositional Control as the Key for Achieving Highly Efficient OER Electrocatalysis with Cobalt Phosphates Decorated Nanocarbon Florets. Small 16 (12), 1903334. doi:10.1002/smll.201903334
Sarkar, S., Dheer, L., Vinod, C. P., Thapa, R., Waghmare, U. V., and Peter, S. C. (2020). Stress-Induced Electronic Structure Modulation of Manganese-Incorporated Ni2P Leading to Enhanced Activity for Water Splitting. ACS Appl. Energ. Mater. 3 (2), 1271–1278. doi:10.1021/acsaem.9b02097
Sheng, S., Shi, B., Wang, C., Luo, L., Lin, X., Li, P., et al. (2020). Antibuoyancy and Unidirectional Gas Evolution by Janus Electrodes with Asymmetric Wettability. ACS Appl. Mater. Inter. 12 (20), 23627–23634. doi:10.1021/acsami.0c04796
Shin, D., Kim, H. J., Kim, M., Shin, D., Kim, H., Song, H., et al. (2020). FexNi2-xP Alloy Nanocatalysts with Electron-Deficient Phosphorus Enhancing the Hydrogen Evolution Reaction in Acidic Media. ACS Catal. 10 (19), 11665–11673. doi:10.1021/acscatal.0c02301
Song, F., Bai, L., Moysiadou, A., Lee, S., Hu, C., Liardet, L., et al. (2018). Transition Metal Oxides as Electrocatalysts for the Oxygen Evolution Reaction in Alkaline Solutions: An Application-Inspired Renaissance. J. Am. Chem. Soc. 140 (25), 7748–7759. doi:10.1021/jacs.8b04546
Song, J., Qiu, S., Hu, F., Ding, Y., Han, S., Li, L., et al. (2021). Sub‐2 Nm Thiophosphate Nanosheets with Heteroatom Doping for Enhanced Oxygen Electrocatalysis. Adv. Funct. Mater. 31 (19), 2100618. doi:10.1002/adfm.202100618
Stoerzinger, K. A., Liang, Q., Biegalski, M. D., and Yang, S. H. (2015). Orientation-Dependent Oxygen Evolution Activities of Rutile IrO2 and RuO2. J. Phys. Chem. Lett. 6 (10), 1636–1641. doi:10.1021/acs.jpclett.5b00694
Sun, H., Xu, X., Yan, Z., Chen, X., Cheng, F., Weiss, P. S., et al. (2017). Porous Multishelled Ni2P Hollow Microspheres as an Active Electrocatalyst for Hydrogen and Oxygen Evolution. Chem. Mater. 29 (19), 8539–8547. doi:10.1021/acs.chemmater.7b03627
Sweeny, N. P., Rohrer, C. S., and Brown, O. W. (1958). Dinickel Phosphide as a Heterogeneous Catalyst for the Vapor Phase Reduction of Nitrobenzene with Hydrogen to Aniline and Water. J. Am. Chem. Soc. 80 (4), 799–800. doi:10.1021/ja01537a012
Tareen, A. K., Priyanga, G. S., Khan, K., Pervaiz, E., Thomas, T., and Yang, M. (2019). Nickel‐Based Transition Metal Nitride Electrocatalysts for the Oxygen Evolution Reaction. ChemSusChem 12 (17), 3941–3954. doi:10.1002/cssc.201900553
Tian, H., Wang, X., Li, H., Pi, M., Zhang, D., and Chen, S. (2020). Superhydrophilic Al‐Doped NiP 2 Nanosheets as Efficient Electrocatalysts for Hydrogen Evolution Reaction. Energy Technol. 8 (1), 1900936. doi:10.1002/ente.201900936
Wang, X.-D., Chen, H.-Y., Xu, Y.-F., Liao, J.-F., Chen, B.-X., Rao, H.-S., et al. (2017). Self-supported NiMoP2 Nanowires on Carbon Cloth as an Efficient and Durable Electrocatalyst for Overall Water Splitting. J. Mater. Chem. A. 5, 7191–7199. doi:10.1039/C6TA11188B
Wang, M., Dong, C.-L., Huang, Y.-C., Li, Y., and Shen, S. (2018). Electronic Structure Evolution in Tricomponent Metal Phosphides with Reduced Activation Energy for Efficient Electrocatalytic Oxygen Evolution. Small 14 (35), 1801756. doi:10.1002/smll.201801756
Wang, L., Wu, H., Xi, S., Chua, S. T., Wang, F., Pennycook, S. J., et al. (2019). Nitrogen-Doped Cobalt Phosphide for Enhanced Hydrogen Evolution Activity. ACS Appl. Mater. Inter. 11 (19), 17359–17367. doi:10.1021/acsami.9b01235
Wu, R., Xiao, B., Gao, Q., Zheng, Y. R., Zheng, X. S., Zhu, J. F., et al. (2018). A Janus Nickel Cobalt Phosphide Catalyst for High‐Efficiency Neutral‐pH Water Splitting. Angew. Chem. Int. Ed. 57 (47), 15445–15449. doi:10.1002/anie.201808929
Wu, T., Zhang, S., Bu, K., Zhao, W., Bi, Q., Lin, T., et al. (2019). Nickel Nitride-Black Phosphorus Heterostructure Nanosheets for Boosting the Electrocatalytic Activity towards the Oxygen Evolution Reaction. J. Mater. Chem. A. 7 (38), 22063–22069. doi:10.1039/C9TA07962A
Xi, W., Yan, G., Lang, Z., Ma, Y., Tan, H., Zhu, H., et al. (2018). Oxygen-Doped Nickel Iron Phosphide Nanocube Arrays Grown on Ni Foam for Oxygen Evolution Electrocatalysis. Small 14 (42), 1802204. doi:10.1002/smll.201802204
Xiao, Y.-X., Ying, J., Tian, G., Tao, Y., Wei, H., Fan, S.-Y., et al. (2019). Highly Dispersed PtPd on Graphitic Nanofibers and its Heavy D-π Effect. Appl. Catal. B: Environ. 259, 118080. doi:10.1016/j.apcatb.2019.118080
Xiao, Y.-X., Ying, J., Tian, G., Zhang, X.-Q., Janiak, C., Ozoemena, K. I., et al. (2021). PtPd Hollow Nanocubes with Enhanced alloy Effect and Active Facets for Efficient Methanol Oxidation Reaction. Chem. Commun. 57 (8), 986–989. doi:10.1039/D0CC06876D
Xiao, Y.-X., Ying, J., Tian, G., Yang, X., Zhang, Y.-X., Chen, J.-B., et al. (2021). Hierarchically Fractal PtPdCu Sponges and Their Directed Mass- and Electron-Transfer Effects. Nano Lett. doi:10.1021/acs.nanolett.1c02268
Xiu, L., Wang, Z., Yu, M., Wu, X., and Qiu, J. (2018). Aggregation-Resistant 3D MXene-Based Architecture as Efficient Bifunctional Electrocatalyst for Overall Water Splitting. ACS Nano 12 (8), 8017–8028. doi:10.1021/acsnano.8b02849
Xu, W., Zhu, S., Liang, Y., Cui, Z., Yang, X., and Inoue, A. (2018). A Nanoporous Metal Phosphide Catalyst for Bifunctional Water Splitting. J. Mater. Chem. A. 6, 5574–5579. doi:10.1039/C8TA00622A
Xu, W., Lu, Z., Sun, X., Jiang, L., and Duan, X. (2018). Superwetting Electrodes for Gas-Involving Electrocatalysis. Acc. Chem. Res. 51 (7), 1590–1598. doi:10.1021/acs.accounts.8b00070
Yan, D., Li, Y., Huo, J., Chen, R., Dai, L., and Wang, S. (2017). Defect Chemistry of Nonprecious-Metal Electrocatalysts for Oxygen Reactions. Adv. Mater. 29 (48), 1606459. doi:10.1002/adma.201606459
Yan, L., Zhang, B., Wu, S., and Yu, J. (2020). A General Approach to the Synthesis of Transition Metal Phosphide Nanoarrays on MXene Nanosheets for pH-Universal Hydrogen Evolution and Alkaline Overall Water Splitting. J. Mater. Chem. A. 8 (28), 14234–14242. doi:10.1039/D0TA05189F
Yang, Z., Zhao, C., Qu, Y., Zhou, H., Zhou, F., Wang, J., et al. (2019). Trifunctional Self‐Supporting Cobalt‐Embedded Carbon Nanotube Films for ORR, OER, and HER Triggered by Solid Diffusion from Bulk Metal. Adv. Mater. 31 (12), 1808043. doi:10.1002/adma.201808043
Ye, Z., Zhang, P., Lei, X., Wang, X., Zhao, N., and Yang, H. (2018). Iron Carbides and Nitrides: Ancient Materials with Novel Prospects. Chem. Eur. J. 24 (36), 8922–8940. doi:10.1002/chem.201706028
Ying, J., Yang, X.-Y., Hu, Z.-Y., Mu, S.-C., Janiak, C., Geng, W., et al. (2014). One Particle@one Cell: Highly Monodispersed PtPd Bimetallic Nanoparticles for Enhanced Oxygen Reduction Reaction. Nano Energy 8, 214–222. doi:10.1016/j.nanoen.2014.06.010
Ying, J., Yang, X.-Y., Tian, G., Janiak, C., and Su, B.-L. (2014). Self-assembly: an Option to Nanoporous Metal Nanocrystals. Nanoscale 6 (22), 13370–13382. doi:10.1039/C4NR03225J
Ying, J., Jiang, G., Paul Cano, Z., Han, L., Yang, X.-Y., and Chen, Z. (2017). Nitrogen-doped Hollow Porous Carbon Polyhedrons Embedded with Highly Dispersed Pt Nanoparticles as a Highly Efficient and Stable Hydrogen Evolution Electrocatalyst. Nano Energy 40, 88–94. doi:10.1016/j.nanoen.2017.07.032
Ying, J., Li, J., Jiang, G., Cano, Z. P., Ma, Z., Zhong, C., et al. (2018). Metal-organic Frameworks Derived Platinum-Cobalt Bimetallic Nanoparticles in Nitrogen-Doped Hollow Porous Carbon Capsules as a Highly Active and Durable Catalyst for Oxygen Reduction Reaction. Appl. Catal. B: Environ. 225, 496–503. doi:10.1016/j.apcatb.2017.11.077
Ying, J., Jiang, G., Cano, Z. P., Ma, Z., and Chen, Z. (2018). Spontaneous Weaving: 3D Porous PtCu Networks with Ultrathin Jagged Nanowires for Highly Efficient Oxygen Reduction Reaction. Appl. Catal. B: Environ. 236, 359–367. doi:10.1016/j.apcatb.2018.04.035
Ying, J., Janiak, C., Xiao, Y.-X., Wei, H., Yang, X.-Y., and Su, B.-L. (2018). Shape-Controlled Surface-Coating to Pd@Mesoporous Silica Core-Shell Nanocatalysts with High Catalytic Activity and Stability. Chem. Asian J. 13 (1), 31–34. doi:10.1002/asia.201701452
Yoon, Y., Tiwari, A. P., Choi, M., Novak, T. G., Song, W., Chang, H., et al. (2019). Precious‐Metal‐Free Electrocatalysts for Activation of Hydrogen Evolution with Nonmetallic Electron Donor: Chemical Composition Controllable Phosphorous Doped Vanadium Carbide MXene. Adv. Funct. Mater. 29 (30), 1903443. doi:10.1002/adfm.201903443
Yu, F., Zhou, H., Huang, Y., Sun, J., Qin, F., Bao, J., et al. (2018). High-Performance Bifunctional Porous Non-Noble Metal Phosphide Catalyst for Overall Water Splitting. Nat. Commun. 9 (1), 2551. doi:10.1038/s41467-018-04746-z
Yu, H.-Z., Wang, Y., Ying, J., Wu, S.-M., Lu, Y., Hu, J., et al. (2019). Hydrogen Evolution Enhancement Over a Cobalt-Based Schottky Interface. ACS Appl. Mater. Inter. 11 (31), 27641–27647. doi:10.1021/acsami.9b03368
Yu, X., Yu, Z.-Y., Zhang, X.-L., Zheng, Y.-R., Duan, Y., Gao, Q., et al. (2019). "Superaerophobic" Nickel Phosphide Nanoarray Catalyst for Efficient Hydrogen Evolution at Ultrahigh Current Densities. J. Am. Chem. Soc. 141 (18), 7537–7543. doi:10.1021/jacs.9b02527
Yu, Y., Zhou, J., and Sun, Z. (2020). Novel 2D Transition‐Metal Carbides: Ultrahigh Performance Electrocatalysts for Overall Water Splitting and Oxygen Reduction. Adv. Funct. Mater. 30 (47), 2000570. doi:10.1002/adfm.202000570
Yue, S., Wang, S., Jiao, Q., Feng, X., Zhan, K., Dai, Y., et al. (2019). Preparation of Yolk-Shell‐Structured Co X Fe 1− X P with Enhanced OER Performance. ChemSusChem 12 (19), 4461–4470. doi:10.1002/cssc.201901604
Zhang, C., Wang, X., Liang, Q., Liu, X., Weng, Q., Liu, J., et al. (2016). Amorphous Phosphorus/Nitrogen-Doped Graphene Paper for Ultrastable Sodium-Ion Batteries. Nano Lett. 16 (3), 2054–2060. doi:10.1021/acs.nanolett.6b00057
Zhang, H., Nai, J., Yu, L., and Lou, X. W. (2017). Metal-Organic-Framework-Based Materials as Platforms for Renewable Energy and Environmental Applications. Joule 1 (1), 77–107. doi:10.1016/j.joule.2017.08.008
Zhang, C., Huang, Y., Yu, Y., Zhang, J., Zhuo, S., and Zhang, B. (2017). Sub-1.1 Nm Ultrathin Porous CoP Nanosheets with Dominant Reactive {200} Facets: a High Mass Activity and Efficient Electrocatalyst for the Hydrogen Evolution Reaction. Chem. Sci. 8, 2769–2775. doi:10.1039/C6SC05687C
Keywords: transition metal phosphides, hydrogen evolution reaction, oxygen evolution reaction, water splitting, modulated strategies
Citation: Ying J and Wang H (2021) Strategies for Developing Transition Metal Phosphides in Electrochemical Water Splitting. Front. Chem. 9:700020. doi: 10.3389/fchem.2021.700020
Received: 25 April 2021; Accepted: 20 August 2021;
Published: 03 November 2021.
Edited by:
Hyun-Seok Cho, Korea Institute of Energy Research, South KoreaReviewed by:
Shengjie Peng, Nanjing University of Aeronautics and Astronautics, ChinaTaekeun Kim, Chungnam National University, South Korea
Copyright © 2021 Ying and Wang. This is an open-access article distributed under the terms of the Creative Commons Attribution License (CC BY). The use, distribution or reproduction in other forums is permitted, provided the original author(s) and the copyright owner(s) are credited and that the original publication in this journal is cited, in accordance with accepted academic practice. No use, distribution or reproduction is permitted which does not comply with these terms.
*Correspondence: Jie Ying, d2h1dHlpbmdqaWVAZ21haWwuY29t