- 1Institute of Biological and Chemical Systems- Functional Molecular Systems (IBCS-FMS), Karlsruhe Institute of Technology (KIT), Karlsruhe, Germany
- 2Institute of Functional Interfaces (IFG), Karlsruhe Institute of Technology (KIT), Karlsruhe, Germany
- 3Karlsruhe Institute of Technology (KIT), EPICUR European University, Karlsruhe, Germany
- 4Institute of Organic Chemistry (IOC), Karlsruhe Institute of Technology (KIT), Karlsruhe, Germany
Addressing intracellular targets is a challenging task that requires potent molecular transporters capable to deliver various cargos. Herein, we report the synthesis of hydrophobic macrocycles composed of both amino acids and peptoid monomers. The cyclic tetramers and hexamers were assembled in a modular approach using solid as well as solution phase techniques. To monitor their intracellular localization, the macrocycles were attached to the fluorophore Rhodamine B. Most molecular transporters were efficiently internalized by HeLa cells and revealed a specific accumulation in mitochondria without the need for cationic charges. The data will serve as a starting point for the design of further cyclic peptoid-peptide hybrids presenting a new class of highly efficient, versatile molecular transporters.
Introduction
Biological membranes envelop intracellular structures, protect them from harmful substances and enable diverse physiological processes. The unique function of these phospholipid bilayers is crucial for all processes of life but also hampers the intracellular delivery of therapeutics or other cargos. The efficient internalization of a certain compound is a key step for both pharmaceutical and basic research. Thus, molecules that overcome the physiological barrier, so-called molecular transporters, are constantly needed not only to resolve and understand the fascinating processes of life but also to provide treatments poor in side effects.
In the late 1980s, the capability of the Trans-Activator of Transcription (Tat) protein of the Human Immunodeficiency Virus (HIV) to overcome cellular barriers without any receptor interaction was discovered. (Green and Loewenstein, 1988; Frankel and Pabo, 1988). Thereby, the foundation for a new compound class, the cell-penetrating peptides (CPPs), was laid. Since then, plenty of different CPPs capable to transport diverse cargos across cellular membranes have been published and outlined in numerous comprehensive reviews. (Koren and Torchilin, 2012; Copolovici et al., 2014; Guidotti et al., 2017; Kalafatovic and Giralt, 2017; Taylor and Zahid, 2020; Falanga et al., 2020; Desale et al., 2021) Although a lot of research is focused on CPPs, some questions remain open. As an example, the uptake mechanism of these molecular transporters is still not fully understood. (Richard et al., 2003; Madani et al., 2011; Ruseska and Zimmer, 2020). Thus, the efficiency enhancement of cellular uptake is one of many subjects of current research. (Nadal Bufí and Henriques, 2020; Fazil et al., 2020; Yin et al., 2020). It has already been shown that a rigidification of the spatial structure increases the activity of some CPPs due to improved interactions with the phospholipid bilayer, limited off-target effects, and a lower entropy penalty. (Lättig-Tünnemann et al., 2011; Nischan et al., 2015; Dougherty et al., 2019; Park et al., 2019) Moreover, the incorporation of certain peptidomimetics could enhance both cellular uptake efficiency and metabolic stability. (Kölmel et al., 2012; Huang et al., 2012; Jing et al., 2012; Vollrath et al., 2013; Koelmel et al., 2014; Dougherty et al., 2019) As CPPs resemble endogenous structures, they are prone to fast proteolytic degradation. (Goodwin et al., 2012; Zhang et al., 2020) Peptidomimetics such as peptoids mimic the structural and functional features of peptides while enhancing their bioavailability. (Dohm et al., 2011; Simon et al., 1992; Zuckermann et al., 1992; Zuckermann, 2011) Peptoids are N-substituted oligoglycines are readily accessible via well-established solid-phase synthesis techniques. (Zuckermann, 2011) Formally, they solely differ from peptides in the location of their side chain which is moved from the α-carbon to the nitrogen atom (Figure 1). However, this shift has a major impact on the conformational flexibility and, thus, on the spatial structure of peptoids (Yoo and Kirshenbaum, 2008; Zuckermann, 2011; Kirshenbaum and Zuckermann, 2019).
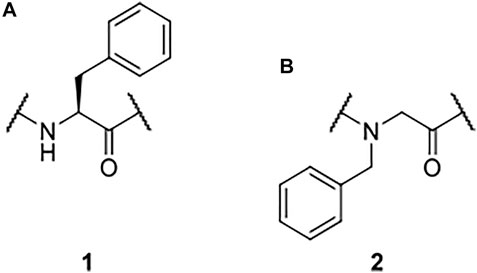
FIGURE 1. Phenylalanine (Green and Loewenstein, 1988) as an example for an amino acid as the building block of a peptide (A) and N1ph (Frankel and Pabo, 1988) as an example for an alkylated glycine monomer (B).
Besides the capability to form hydrogen bonds, the chirality at the α-carbon is lost when transforming peptides to peptoids. Interestingly, it has been shown that the absence of these features can even increase the efficiency of cellular uptake. (Wender et al., 2000; Goun et al., 2006; Tan et al., 2008; Schwochert et al., 2015) Together with their high stability against enzymatic degradation, cell-penetrating peptoids (CPPos) constitute promising tools for cargo delivery.
So far, mainly positively charged CPPos have been synthesized with cellular uptake specificity for endosomes, the cytosol, or the nucleus. (Murphy et al., 1998; Wender et al., 2000; Wright et al., 2003; Schröder et al., 2007; Schröder et al., 2008; Eggenberger et al., 2009; Unciti-Broceta et al., 2009; Huang et al., 2012; Kölmel et al., 2012; Sternberg et al., 2013; Kölmel et al., 2014; Marouseau et al., 2017) The incorporation of a critical amount of lipophilic side chains led to a specific accumulation in mitochondria. (Kölmel et al., 2012; Nam et al., 2018) It was stated, that a cationic charge may be a prerequisite for interaction with the negatively charged head groups of the phospholipid bilayer and subsequent cellular uptake through the plasma membrane. (Wender et al., 2000; Peretto et al., 2003; Madani et al., 2011; Koren and Torchilin, 2012; Guidotti et al., 2017) Shin et al. (Shin et al., 2018) showed that the efficiency of this cellular uptake is significantly increased after cyclization of linear CPPos.
As a defined three-dimensional structure caused by cyclization has proved to be beneficial for cellular uptake, (Lättig-Tünnemann et al., 2011; Nischan et al., 2015; Shin et al., 2018; Dougherty et al., 2019; Park et al., 2019), we herein report the synthesis of macrocyclic tetramers and hexamers as novel molecular transporters. The macrocycles are composed of both alkylated glycine monomers and amino acids to maintain steric information that may be crucial for high biological activity. (Agrawal et al., 2007; Henriques et al., 2019) These cyclic hybrids constitute a promising substance class combining the unique selectivity of peptides with the outstanding bioavailability of peptoids (Olsen, 2010; Schwochert et al., 2015).
Results and Discussion
Macrocyclic tetramers and hexamers were synthesized in a multistep procedure using solid and solution phase methods. The synthetic approach involved the assembly of linear precursors on solid supports following the submonomer method published by Zuckermann (Zuckermann et al., 1992) as well as the solid phase peptide synthesis (SPPS) described by Merrifield (Merrifield, 1963). A 2-chlorotrityl chloride polystyrene resin (3) served as solid support (Scheme 1).
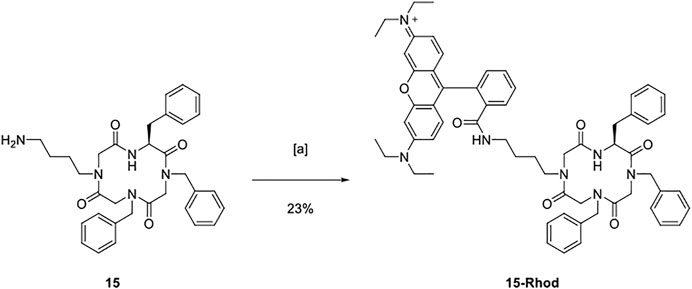
SCHEME 1. Synthetic approach for the assembly of linear precursors on solid support. [A]: Fmoc-protected amino acid, N,N′-diisopropylethylamine (DIPEA), N-methyl-2-pyrrolidone (NMP), 21°C, 16 h; [B]: piperidine, dimethylformamide (DMF), 21°C, 3 × 5 min; [C]: Fmoc-protected amino acid, N,N’-diisopropylcarbodiimide (DIC), hydroxybenzotriazole, NMP, 21°C, 4 h; [D]: bromoacetic acid, DIPEA, DMF, 21°C, 1 h; [E]: amine, DMF, 21°C, 1–16 h; [F]: bromoacetic acid, DIC, DMF, 21°C, 30 min; [G]: hexafluoroisopropanol, methylene chloride, 21°C, 16 h.
The submonomer method requires an acetylation step using a haloacetic acid followed by a substitution implementing the desired side chain. (Zuckermann et al., 1992) Thus, bromoacetic acid was either attached to the resin under basic conditions (→ 7) or to the growing oligomer chain using diisopropylcarbodiimide (DIC) as a coupling reagent. A certain amine was added allowing for the assembly of an alkylated glycine monomer (→ 8). The possibility to incorporate any amine gives rise to various side chains and, thus, easily tunable properties of the resulting peptoids. For the design of molecular transporters, the substitution step was performed with four different amines that led to the assembly of the alkylated glycine monomers N1ph (2), N1phpCl (12), N3m (13), and N4am (14, Figure 2). The former ones were empirically chosen to examine the influence of lipophilicity on the biological activity of the respective macrocycles. Thereby, all lipophilic monomers should allow for an accumulation in mitochondria. (Kölmel et al., 2012; Nam et al., 2018) The peptoid monomer N4am (14) served as conjugation site for the fluorophore Rhodamine B.
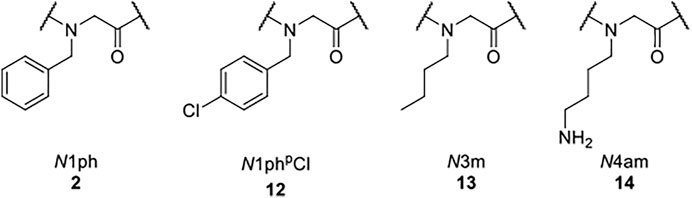
FIGURE 2. Alkylated glycine monomers 2 and 12–14 used for the synthesis of molecular transporters and their applied designation.
The SPPS was performed using Fmoc-protected amino acids. They were analogously attached to the resin (→ 4) under basic conditions or to the growing linear precursor using DIC. To avoid racemization at the α-carbon, hydroxybenzotriazole was added for amino acid couplings. The temporary Fmoc-protecting group was cleaved with piperidine after coupling (→ 5). The combination of both solid-phase methods enabled the straightforward synthesis of linear hybrids composed of alkylated glycine monomers as well as amino acids. The linear precursors 11 were cleaved from the resin under mildly acidic conditions releasing a carboxylic acid at the C-terminus.
The subsequent head-to-tail cyclization using the crude linear precursors and [dimethylamino(triazolo[4,5-b]pyridine-3-yloxy)methylidene]-dimethylazanium hexafluorophosphate (HATU) as potent coupling agent was performed under high dilution conditions to avoid favored side reactions like dimerizations. (Aldrich et al., 2011; Thakkar et al., 2013) Thus, the respective linear precursor was added dropwise to an extensively stirred solution of HATU. Thereby, the concentration of the added linear peptide-peptoid hybrid solution did not exceed 7.50 mm. After eleven or respectively fifteen reaction steps, the macrocycles were purified via preparative reversed-phase high performance liquid chromatography (HPLC). Product formation was confirmed via matrix-assisted laser desorption/ionization-time of flight (MALDI-TOF) mass spectrometry and subsequent nuclear magnetic resonance (NMR) spectroscopy. The synthetic protocol yielded the macrocyclic compounds 15–24 (Figure 3).
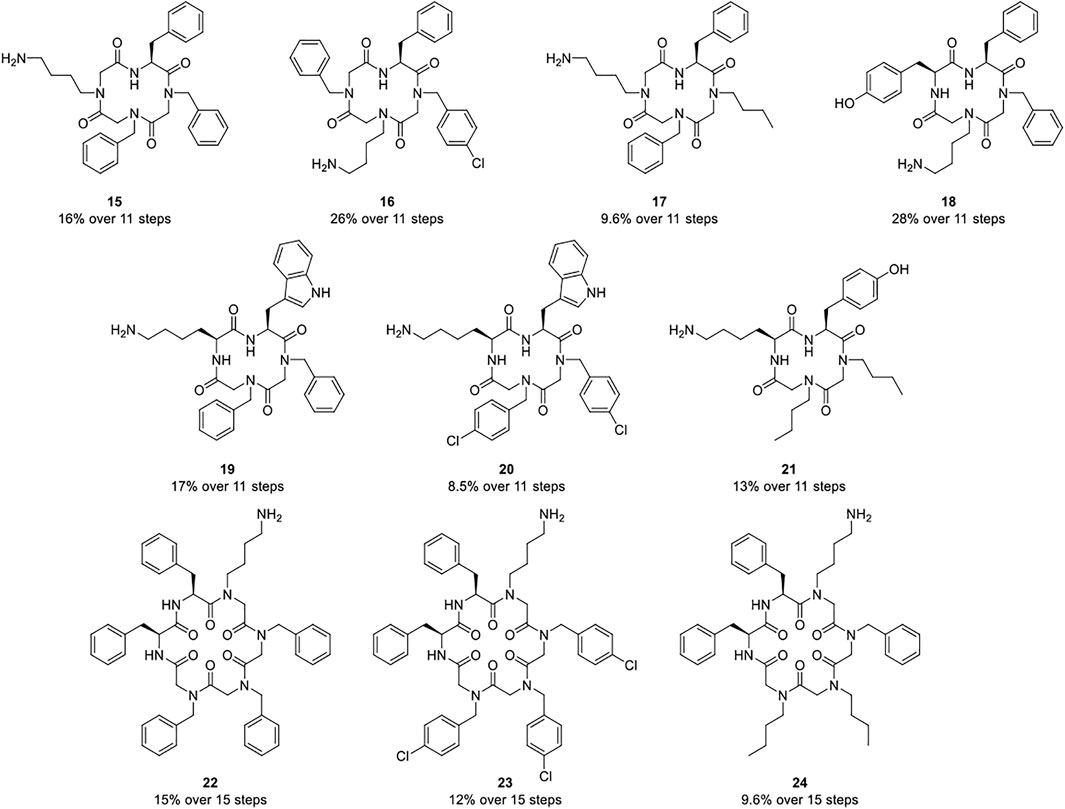
FIGURE 3. Macrocyclic hybrids 15–24 composed of alkylated glycine monomers and amino acids that were assembled via a combination of solid and solution phase methods.
Seven cyclic tetramers (15–21) and three cyclic hexamers (22–24) were successfully synthesized. Every macrocycle is composed of several alkylated glycine monomers and up to two amino acids. The hybrids share aromatic as well as aliphatic residues and an aminobutyl side chain. In every case, an aromatic amino acid represents the N-terminus of the linear precursor. It was assumed that the higher nucleophilicity of a primary amine might be beneficial for the subsequent ring closure reaction.
Regardless of their size and composition, all macrocycles were isolated in similar yields (14% ± 5) and satisfying purities (94% ± 5). For the assembly of hybrids 15–17, an alkylated glycine monomer was attached to the solid support. Compared to tetramers 18–21 that were immobilized via an amino acid, the yields of the respective linear precursors were slightly decreased: While the crude linear tetramers of macrocycles 18–21 were isolated in 70% ± 14 yields, the precursors of tetramers 15–17 yielded 41% ± 5. It is noteworthy, that either the attachment of an alkylated glycine monomer to the solid support or the number of alkylated glycine monomers might be decisive for this discrepancy. Cyclization occurred to be more efficient for hybrids 15–17, possibly due to increased conformational flexibility, (Yoo et al., 2010; De Riccardis, 2020), resulting in similar overall yields.
As the attachment of an amino acid to the solid support proved to be more efficient, linear hexamers were immobilized vial-phenylalanine (1). Due to the additional reaction steps, the yields of the crude linear hexamers were lowered (49% ± 13). Nevertheless, every linear precursor was synthesized in a sufficient quantity for cyclization. Depending on the amount of the linear precursor isolated, cyclization was carried out by the addition of a 3.25–7.50 mm solution of the respective linear hybrid. However, no effects on the efficiency of the ring closure were observed within this concentration range.
With the macrocyclic hybrids in hands, ten hydrophobic molecular transporters were synthesized. As a cargo, the potent fluorophore Rhodamine B was chosen. The chromophore is known for its biocompatibility and is widely used in biological research. (Lavis and Raines, 2008) Rhodamine B was attached via its carboxylic moiety to the aminobutyl side chains of the single macrocycles (Scheme 2).
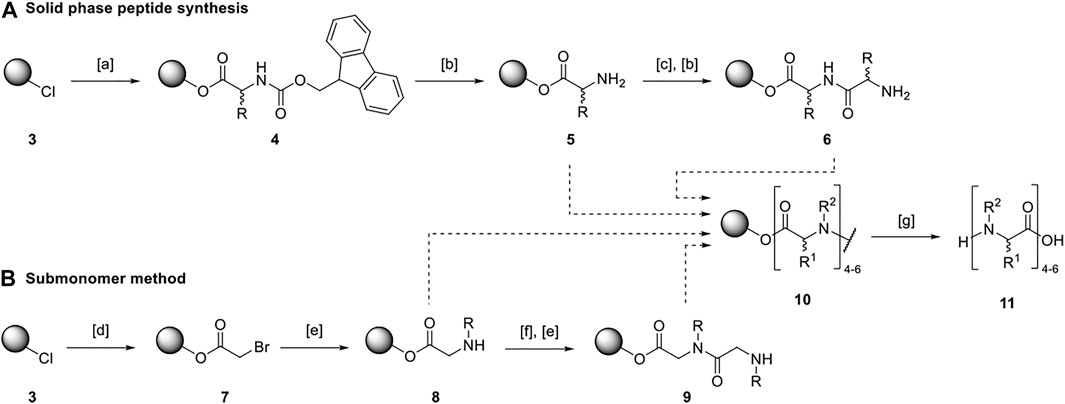
SCHEME 2. Conjugation of the chromophore Rhodamine B to a cyclic hybrid exemplarily shown for macrocycle 15. [A] Rhodamine B, HATU, DIPEA, DMF, 36 h, 21°C.
The conjugation of Rhodamine B was conducted by the cyclization of linear precursors using HATU as a reliable activating agent. The labeling occurred in moderate yields (27% ± 5) and excellent purities (Table 1).
The cytotoxicity of the macrocyclic hybrids 15–24, as well as their conjugates 15-Rhod to 24-Rhod, was examined using a standard MTT assay in human epithelial cervix carcinoma (HeLa) cells. This colorimetric assay displays the cell viability based on their capability to reduce the tetrazolium dye 3-(4,5-dimethylthiazol-2-yl)-2,5-diphenyltetrazolium bromide (MTT). (Mosmann, 1983; Tolosa et al., 2015) After treatment of HeLa cells with different concentrations of the respective macrocycles 15–24 (0.50─50 µm) for 72 h, no significant cytotoxic effects were observed. Most hybrids resulted in IC50 > 50 µm. However, in most cases the attachment of the chromophore Rhodamine B slightly increased the cytotoxicity of the respective molecular transporters 15-Rhod–24-Rhod (Table 2). As shown by many research groups that Rhodamine B by itself is not efficiently entering the cells showing therefore an LD50 > 50 μM, we assume that this the toxicity of the Rhodamine moiety might be higher when increasingly localizing in the mitochondria to a higher amount.
Conjugate 23-Rhod is the only hybrid with an IC50 value in a single-digit micromolar range (IC50 = 6 µm). Its parent macrocycle 23 turned out to be the most cytotoxic hybrid tested (IC50 = 20 µm). Considering every cyclic hybrid, the incorporation of the alkylated glycine monomer N1phpCl (12) was decisive for the cytotoxicity of the respective macrocycles: the higher the amount of N1phpCl building blocks, the lower the viability of the HeLa cells after treatment. However, comparing the macrocyclic transporters 19-Rhod and 20-Rhod, the substitution of two N1ph (2) monomers by two N1phpCl building blocks led to a decrease of cytotoxicity.
As 15-Rhod to 24-Rhod were not toxic at 5 µM the following transport experiments were carried out using this concentration. To study their cellular uptake and organellar localization, HeLa cells were treated with 5 µM of the cyclic conjugates 15-Rhod to 24-Rhod for 5 h and eventually imaged using confocal microscopy (for experimental details, see supplemental Material).
The molecular transporters 15-Rhod, 16-Rhod, 20-Rhod-22-Rhod, and 24-Rhod were efficiently taken up by the cells and localized to different organelles due to their differences in their side-chain corona. Counterstaining of mitochondria using the mitochondrial marker MitoTracker Green® gave evidence of a mitochondrial accumulation for conjugates 15-Rhod to 17-Rhod and 19-Rhod to 24-Rhod (Figure 4).
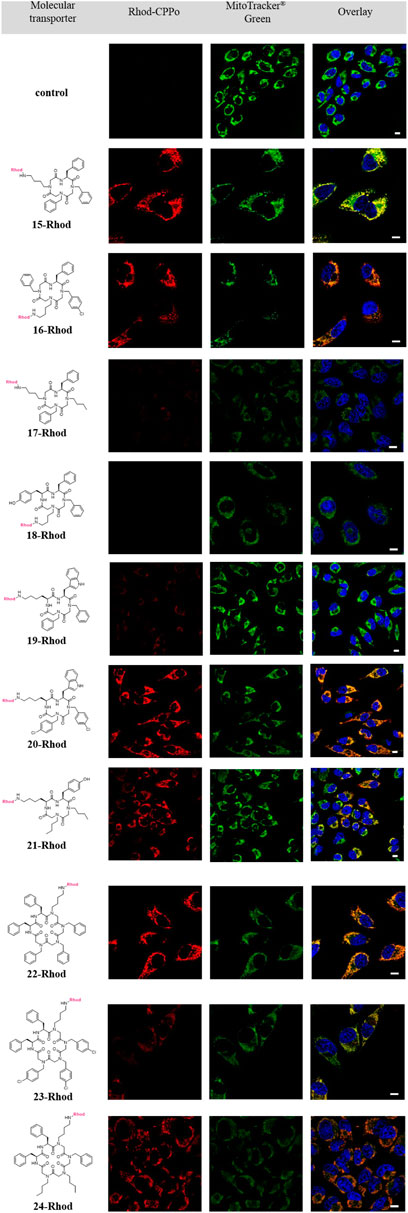
FIGURE 4. Localization of the macrocyclic conjugates 15-Rhod to 24-Rhod in HeLa cells. After incubation with a 5.0 µm solution of the respective transporters for 5 h, the cellular uptake was monitored via fluorescent confocal microscopy (Rhodamine B labeled hybrids: λExc = 532 nm, λEm = 570–620 nm; MitoTracker® Green: λExc = 488 nm, λEm = 490–540 nm; Hoechst 33,342: λExc = 405 nm, λEm = 430–490 nm). Counterstaining with MitoTracker® Green visualized mitochondria (green). Staining with 2 μg/ml Hoechst 33,342 indicated nuclei (blue). Scale bar: 10 µm.
A high cellular uptake efficiency, as well as mitochondrial specificity, was observed for the molecular transporters 15-Rhod, 16-Rhod, 20-Rhod to 22-Rhod, and 24-Rhod. Mitochondrial accumulation was also shown for conjugates 17-Rhod, 19-Rhod and 23-Rhod, however, their cellular uptake was significantly less efficient. An interesting discrepancy in the uptake efficiency was observed when comparing the molecular transporters 18-Rhod and 21-Rhod which are both characterized by the amino acid l-tyrosine. While 18-Rhod containing two benzyl side chains was not internalized by the HeLa cells, conjugate 21-Rhod, in which both benzyl moieties were replaced by two aliphatic side chains, was efficiently taken up. By comparison of the cyclic tetramers 15-Rhod and 17-Rhod as well as the hexamers 22-Rhod and 24-Rhod, the substitution of aromatic residues by aliphatic side chains had no positive effect on the cellular efficiency. 17-Rhod was even less internalized than 15-Rhod. Regarding substitutions of N1ph (2) by its halogenated relative N1phpCl (13), no conclusive effects were observed as well. Conjugates 15-Rhod and 16-Rhod showed a similar cellular uptake efficiency, while an exchange of two N1ph (2) side chains by N1phpCl (13) increased the uptake of the molecular transporter 20-Rhod compared to conjugate 19-Rhod. However, the halogenation of three N1ph (2) building blocks led to a significantly decreased cellular uptake efficiency which becomes evident by comparison of hexamers 22-Rhod and 23-Rhod.
The molecular transporters 15-Rhod, 20-Rhod and 22-Rhod are characterized by low cytotoxicity, efficient cellular uptake, and high specificity. Thus, they represent the most promising cyclic hybrids capable of the transport of different cargos into mitochondria. The data obtained support previous studies on peptides and peptoids indicating that a certain amount of lipophilic residues may be crucial for mitochondrial targeting. (Kölmel et al., 2012; Nam et al., 2018) Besides, the data prove that no cationic charges are needed for mitochondrial localization. The capability of hydrophobic structures to function as molecular transporters is also known for so-called hydrophobic CPPs. However, only a few members of this substance class have been discovered yet. (Xie et al., 2020; Desale et al., 2021) It is assumed, that hydrophobic CPPs may penetrate cellular membranes in a direct, energy-independent manner dispensing with endocytosis. (Gao et al., 2002; Gao et al., 2011; Nakayama et al., 2011; Guidotti et al., 2017; Tian et al., 2017) The latter leads to a major challenge as molecular transporters might not escape the endosomes and get degraded instead of reaching their designated targets. (Nadal Bufí and Henriques, 2020) As exemplaryly shown for the macrocyclic transporter 22-Rhod, the mitochondrial uptake is occurring at 37°C as well as at 4°C (see supplemental material, Supplementary Figure S1). The efficient internalization at a low temperature led us to the assumption that 22-Rhod might directly penetrate the cell membrane. However, the uptake mechanism of macrocyclic hybrids will be further examined.
The versatile substance class of peptide-peptoid macrocycles shows high potential as molecular transporters. Their specific targeting of mitochondria paves the way for the treatment of various diseases that are associated with these organelles. (Malty et al., 2015; Javadov et al., 2020; Tan and Finkel, 2020) Structural studies on macrocyclic hybrids as well as an establishment of a comprehensive structure-activity relationship will henceforth enlarge their applicability as versatile tools in pharmaceutical and basic research.
Conclusion
Ten macrocyclic peptide-peptoid hybrids were synthesized in a straightforward approach using solid and liquid phase techniques. Their unique structure combines the advantages of both peptides and peptoids. The conformational constraints introduced by chiral amino acids as well as the cyclization of their backbone render them promising model structures for selective compounds. Their capability to form hydrogen bonds makes them soluble in an aqueous environment. Furthermore, the incorporation of N-alkylated glycine monomers resulting in a hybrid structure increases their polarity and variability by allowing for the incorporation of plenty of different side chains and easily tunable properties. The hydrophobic molecular transporters presented selectively accumulated in mitochondria without the need for additional cationic charges. Thus, their uptake mechanism might differ from known CPPs and CPPos. Further studies on these versatile structures will reveal their potential as selective and highly efficient molecular transporters.
Data Availability Statement
The data presented in this study are openly available in the Chemotion repository: www.chemotion-repository.net.
Author Contributions
CH synthesized all compounds and examined their cytotoxicity. AM did the microscopy. SB and US designed the strategy and process.
Funding
This research has been funded by the Deutsche Forschungsgemeinschaft (DFG, German Research Foundation) within the Research Training Group (RTG) 2039 “Molecular architecture for fluorescent cell imaging” as well as by the Deutsche Forschungsgemeinschaft (DFG, German Research Foundation) under Germany´s Excellence Strategy via the Excellence Cluster 3D Matter Made to Order (EXC-2082/1—390761711). The authors acknowledge financial support by the Helmholtz program P3 from the Research Field Information and the KIT publication fund.
Conflict of Interest
The authors declare that the research was conducted in the absence of any commercial or financial relationships that could be construed as a potential conflict of interest.
The reviewer AB declared a past co-authorship with one of the authors CH to the handling Editor.
Supplementary Material
The Supplementary Material for this article can be found online at: https://www.frontiersin.org/articles/10.3389/fchem.2021.696957/full#supplementary-material
References
Agrawal, Y., Bhatt, H., Raval, H., Oza, P., and Gogoi, P. (2007). Chirality - A New Era of Therapeutics. Mrmc 7 (5), 451–460. doi:10.2174/138955707780619617
Aldrich, J. V., Kulkarni, S. S., Senadheera, S. N., Ross, N. C., Reilley, K. J., Eans, S. O., et al. (2011). Unexpected Opioid Activity Profiles of Analogues of the Novel Peptide Kappa Opioid Receptor Ligand CJ-15,208. ChemMedChem 6 (9), 1739–1745. doi:10.1002/cmdc.201100113
Copolovici, D. M., Langel, K., Eriste, E., and Langel, Ü. (2014). Cell-penetrating Peptides: Design, Synthesis, and Applications. ACS nano 8 (3), 1972–1994. doi:10.1021/nn4057269
De Riccardis, F. (2020). The Challenge of Conformational Isomerism in Cyclic Peptoids. Eur. J. Org. Chem. 2020 (20), 2981–2994. doi:10.1002/ejoc.201901838
Desale, K., Kuche, K., and Jain, S. (2021). Cell-penetrating Peptides (CPPs): an Overview of Applications for Improving the Potential of Nanotherapeutics. Biomater. Sci 9 (4), 1153–1188. doi:10.1039/d0bm01755h
Dougherty, P. G., Sahni, A., and Pei, D. (2019). Understanding Cell Penetration of Cyclic Peptides. Chem. Rev. 119 (17), 10241–10287. doi:10.1021/acs.chemrev.9b00008
Dohm, M. T., Kapoor, R., and Barron, A. E. (2011). Peptoids: Bio-Inspired Polymers as Potential Pharmaceuticals. Curr. Pharm. 17 (25), 2732–2747. doi:10.2174/138161211797416066
Eggenberger, K., Birtalan, E., Schröder, T., Bräse, S., and Nick, P. (2009). Passage of Trojan Peptoids into Plant Cells. ChemBioChem 10 (15), 2504–2512. doi:10.1002/cbic.200900331
Falanga, A., Lombardi, L., Galdiero, E., Genio, V. D., and Galdiero, S. (2020). The World of Cell Penetrating: the Future of Medical Applications. Future Med. Chem. 12 (15), 1431–1446. doi:10.4155/fmc-2020-0140
Fazil, M. H. U. T., Chalasani, M. L. S., Choong, Y. K., Schmidtchen, A., Verma, N. K., and Saravanan, R. (2020). A C-Terminal Peptide of TFPI-1 Facilitates Cytosolic Delivery of Nucleic Acid Cargo into Mammalian Cells. Biochim. Biophys. Acta (Bba) - Biomembranes 1862 (2), 183093. doi:10.1016/j.bbamem.2019.183093
Frankel, A. D., and Pabo, C. O. (1988). Cellular Uptake of the Tat Protein from Human Immunodeficiency Virus. Cell 55 (6), 1189–1193. doi:10.1016/0092-8674(88)90263-2
Gao, C., Mao, S., Ditzel, H. J., Farnaes, L., Wirsching, P., Lerner, R. A., et al. (2002). A Cell-Penetrating Peptide from a Novel pVII-pIX Phage-Displayed Random Peptide Library. Bioorg. Med. Chem. 10 (12), 4057–4065. doi:10.1016/s0968-0896(02)00340-1
Gao, S., Simon, M. J., Hue, C. D., Morrison, B., and Banta, S. (2011). An Unusual Cell Penetrating Peptide Identified Using a Plasmid Display-Based Functional Selection Platform. ACS Chem. Biol. 6 (5), 484–491. doi:10.1021/cb100423u
Goodwin, D., Simerska, P., and Toth, I. (2012). Peptides as Therapeutics with Enhanced Bioactivity. Cmc 19 (26), 4451–4461. doi:10.2174/092986712803251548
Goun, E. A., Pillow, T. H., Jones, L. R., Rothbard, J. B., and Wender, P. A. (2006). Molecular Transporters: Synthesis of Oligoguanidinium Transporters and Their Application to Drug Delivery and Real-Time Imaging. ChemBioChem 7 (10), 1497–1515. doi:10.1002/cbic.200600171
Green, M., and Loewenstein, P. M. (1988). Autonomous Functional Domains of Chemically Synthesized Human Immunodeficiency Virus Tat Trans-activator Protein. Cell 55 (6), 1179–1188. doi:10.1016/0092-8674(88)90262-0
Guidotti, G., Brambilla, L., and Rossi, D. (2017). Cell-penetrating Peptides: from Basic Research to Clinics. Trends Pharmacological Sciences 38 (4), 406–424. doi:10.1016/j.tips.2017.01.003
Henriques, S. T., Peacock, H., Benfield, A. H., Wang, C. K., and Craik, D. J. (2019). Is the Mirror Image a True Reflection? Intrinsic Membrane Chirality Modulates Peptide Binding. J. Am. Chem. Soc. 141 (51), 20460–20469. doi:10.1021/jacs.9b11194
Huang, W., Seo, J., Lin, J. S., and Barron, A. E. (2012). Peptoid Transporters: Effects of Cationic, Amphipathic Structure on Their Cellular Uptake. Mol. Biosyst. 8 (10), 2626–2628. doi:10.1039/c2mb25197c
Javadov, S., Kozlov, A. V., and Camara, A. K. S. (2020). Mitochondria in Health and Diseases. Cells 9, 1177. doi:10.3390/cells9051177
Jing, X., Yang, M., Kasimova, M. R., Malmsten, M., Franzyk, H., Jorgensen, L., et al. (2012). Membrane Adsorption and Binding, Cellular Uptake and Cytotoxicity of Cell-Penetrating Peptidomimetics with α-peptide/β-peptoid Backbone: Effects of Hydrogen Bonding and α-chirality in the β-peptoid Residues. Biochim. Biophys. Acta (Bba) - Biomembranes 1818 (11), 2660–2668. doi:10.1016/j.bbamem.2012.05.003
Kalafatovic, D., and Giralt, E. (2017). Cell-penetrating Peptides: Design Strategies beyond Primary Structure and Amphipathicity. Molecules 22 (11), 1929. doi:10.3390/molecules22111929
Kirshenbaum, K., and Zuckermann, R. N. (2019). Molecular Folding Science. Biopolymers 110 (6), e23314. doi:10.1002/bip.23314
Koelmel, D. K., Hoerner, A., Roenicke, F., Nieger, M., Schepers, U., and Braese, S. (2014). Cell-penetrating Peptoids: Introduction of Novel Cationic Side Chains. Eur. J. Med. Chem. 79, 231–243. doi:10.1016/j.ejmech.2014.03.078
Kölmel, D., Fürniss, D., Susanto, S., Lauer, A., Grabher, C., Bräse, S., et al. (2012). Cell Penetrating Peptoids (CPPos): Synthesis of a Small Combinatorial Library by Using IRORI MiniKans. Pharmaceuticals 5 (12), 1265–1281. doi:10.3390/ph5121265
Kölmel, D. K., Hörner, A., Rönicke, F., Nieger, M., Schepers, U., and Bräse, S. (2014). Cell-penetrating Peptoids: Introduction of Novel Cationic Side Chains. Eur. J. Med. Chem. 79, 231–243. doi:10.1016/j.ejmech.2014.03.078
Koren, E., and Torchilin, V. P. (2012). Cell-penetrating Peptides: Breaking through to the Other Side. Trends Molecular Medicine 18 (7), 385–393. doi:10.1016/j.molmed.2012.04.012
Lättig-Tünnemann, G., Prinz, M., Hoffmann, D., Behlke, J., Palm-Apergi, C., Morano, I., et al. (2011). Backbone Rigidity and Static Presentation of Guanidinium Groups Increases Cellular Uptake of Arginine-Rich Cell-Penetrating Peptides. Nat. Commun. 2 (1), 1–6. doi:10.1038/ncomms1459
Lavis, L. D., and Raines, R. T. (2008). Bright Ideas for Chemical Biology. ACS Chem. Biol. 3 (3), 142–155. doi:10.1021/cb700248m
Madani, F., Lindberg, S., Langel, Ü., Futaki, S., and Gräslund, A. (2011). Mechanisms of Cellular Uptake of Cell-Penetrating Peptides. J. Biophys. 2011, 414729. doi:10.1155/2011/414729
Malty, R. H., Jessulat, M., Jin, K., Musso, G., Vlasblom, J., Phanse, S., et al. (2015). Mitochondrial Targets for Pharmacological Intervention in Human Disease. J. Proteome Res. 14 (1), 5–21. doi:10.1021/pr500813f
Marouseau, E., Neckebroeck, A., Larkin, H., Le Roux, A., Volkov, L., Lavoie, C. L., et al. (2017). Modular Sub-monomeric Cell-Penetrating Guanidine-Rich Peptoids - Synthesis, Assembly and Biological Evaluation. RSC Adv. 7 (10), 6059–6063. doi:10.1039/c6ra27898a
Merrifield, R. B. (1963). Solid Phase Peptide Synthesis. I. The Synthesis of a Tetrapeptide. J. Am. Chem. Soc. 85 (14), 2149–2154. doi:10.1021/ja00897a025
Mosmann, T. (1983). Rapid Colorimetric Assay for Cellular Growth and Survival: Application to Proliferation and Cytotoxicity Assays. J. Immunological Methods 65, 55–63. doi:10.1016/0022-1759(83)90303-4
Murphy, J. E., Uno, T., Hamer, J. D., Cohen, F. E., Dwarki, V., and Zuckermann, R. N. (1998). A Combinatorial Approach to the Discovery of Efficient Cationic Peptoid Reagents for Gene Delivery. Proc. Natl. Acad. Sci. 95 (4), 1517–1522. doi:10.1073/pnas.95.4.1517
Nadal Bufí, F., and Henriques, S. T. (2020). How to Overcome Endosomal Entrapment of Cell‐penetrating Peptides to Release the Therapeutic Potential of Peptides?. Pept. Sci. 112 (6), e24168. doi:10.1002/pep2.24168
Nakayama, F., Yasuda, T., Umeda, S., Asada, M., Imamura, T., Meineke, V., et al. (2011). Fibroblast Growth Factor-12 (FGF12) Translocation into Intestinal Epithelial Cells Is Dependent on a Novel Cell-Penetrating Peptide Domain. J. Biol. Chem. 286 (29), 25823–25834. doi:10.1074/jbc.m110.198267
Nam, H. Y., Hong, J.-A., Choi, J., Shin, S., Cho, S. K., Seo, J., et al. (2018). Mitochondria-Targeting Peptoids. Bioconjug. Chem. 29 (5), 1669–1676. doi:10.1021/acs.bioconjchem.8b00148
Nischan, N., Herce, H. D., Natale, F., Bohlke, N., Budisa, N., Cardoso, M. C., et al. (2015). Covalent Attachment of Cyclic TAT Peptides to GFP Results in Protein Delivery into Live Cells with Immediate Bioavailability. Angew. Chem. Int. Ed. 54 (6), 1950–1953. doi:10.1002/anie.201410006
Olsen, C. A. (2010). Peptoid-Peptide Hybrid Backbone Architectures. Chem. Eur. J. Chem. Bio. 11 (2), 152–160. doi:10.1002/cbic.200900618
Park, S. E., Sajid, M. I., Parang, K., and Tiwari, R. K. (2019). Cyclic Cell-Penetrating Peptides as Efficient Intracellular Drug Delivery Tools. Mol. Pharmaceutics 16 (9), 3727–3743. doi:10.1021/acs.molpharmaceut.9b00633
Peretto, I., Sanchez-Martin, R. M., Wang, X. H., Ellard, J., Mittoo, S., and Bradley, M. (2003). Cell Penetrable Peptoid Carrier Vehicles: Synthesis and evaluation. Chem. Commun. 18 (18), 2312–2313. doi:10.1039/b306438g
Richard, J. P., Melikov, K., Vives, E., Ramos, C., Verbeure, B., Gait, M. J., et al. (2003). Cell-penetrating Peptides. J. Biol. Chem. 278 (1), 585–590. doi:10.1074/jbc.m209548200
Ruseska, I., and Zimmer, A. (2020). Internalization Mechanisms of Cell-Penetrating Peptides. Beilstein J. Nanotechnol. 11 (1), 101–123. doi:10.3762/bjnano.11.10
Schröder, T., Niemeier, N., Afonin, S., Ulrich, A. S., Krug, H. F., and Bräse, S. (2008). Peptoidic Amino- and Guanidinium-Carrier Systems: Targeted Drug Delivery into the Cell Cytosol or the Nucleus. J. Med. Chem. 51 (3), 376–379. doi:10.1021/jm070603m
Schröder, T., Schmitz, K., Niemeier, N., Balaban, T. S., Krug, H. F., Schepers, U., et al. (2007). Solid-phase Synthesis, Bioconjugation, and Toxicology of Novel Cationic Oligopeptoids for Cellular Drug Delivery. Bioconjug. Chem. 18 (2), 342–354. doi:10.1021/bc0602073
Schwochert, J., Turner, R., Thang, M., Berkeley, R. F., Ponkey, A. R., Rodriguez, K. M., et al. (2015). Peptide to Peptoid Substitutions Increase Cell Permeability in Cyclic Hexapeptides. Org. Lett. 17 (12), 2928–2931. doi:10.1021/acs.orglett.5b01162
Shin, M.-K., Hyun, Y.-J., Lee, J. H., and Lim, H.-S. (2018). Comparison of Cell Permeability of Cyclic Peptoids and Linear Peptoids. ACS Comb. Sci. 20 (4), 237–242. doi:10.1021/acscombsci.7b00194
Simon, R. J., Kania, R. S., Zuckermann, R. N., Huebner, V. D., Jewell, D. A., Banville, S., et al. (1992). Peptoids: a Modular Approach to Drug Discovery. Proc. Natl. Acad. Sci. 89 (20), 9367–9371. doi:10.1073/pnas.89.20.9367
Sternberg, U., Birtalan, E., Jakovkin, I., Luy, B., Schepers, U., Bräse, S., et al. (2013). Structural Characterization of a Peptoid with Lysine-like Side Chains and Biological Activity Using NMR and Computational Methods. Org. Biomol. Chem. 11 (4), 640–647. doi:10.1039/c2ob27039k
Tan, J. X., and Finkel, T. (2020). Mitochondria as Intracellular Signaling Platforms in Health and Disease. J. Cel Biol. 219 (5), e202002179. doi:10.1083/jcb.202002179
Tan, N. C., Yu, P., Kwon, Y.-U., and Kodadek, T. (2008). High-throughput Evaluation of Relative Cell Permeability between Peptoids and Peptides. Bioorg. Med. Chem. 16 (11), 5853–5861. doi:10.1016/j.bmc.2008.04.074
Taylor, R. E., and Zahid, M. (2020). Cell Penetrating Peptides, Novel Vectors for Gene Therapy. Pharmaceutics 12 (3), 225. doi:10.3390/pharmaceutics12030225
Thakkar, A., Trinh, T. B., and Pei, D. (2013). Global Analysis of Peptide Cyclization Efficiency. ACS Comb. Sci. 15 (2), 120–129. doi:10.1021/co300136j
Tian, Y., Zeng, X., Li, J., Jiang, Y., Zhao, H., Wang, D., et al. (2017). Achieving Enhanced Cell Penetration of Short Conformationally Constrained Peptides through Amphiphilicity Tuning. Chem. Sci. 8 (11), 7576–7581. doi:10.1039/c7sc03614k
Tolosa, L., Donato, M. T., and Gómez-Lechón, M. J. (2015). General Cytotoxicity Assessment by Means of the MTT Assay. Methods Mol. Biol. 1250, 333. doi:10.1007/978-1-4939-2074-7_26
Unciti-Broceta, A., Diezmann, F., Ou-Yang, C. Y., Fara, M. A., and Bradley, M. (2009). Synthesis, Penetrability and Intracellular Targeting of Fluorescein-Tagged Peptoids and Peptide-Peptoid Hybrids. Bioorg. Med. Chem. 17 (3), 959–966. doi:10.1016/j.bmc.2008.02.068
Vollrath, S. B. L., Fürniss, D., Schepers, U., and Bräse, S. (2013). Amphiphilic Peptoid Transporters - Synthesis and Evaluation. Org. Biomol. Chem. 11 (47), 8197–8201. doi:10.1039/c3ob41139g
Wender, P. A., Mitchell, D. J., Pattabiraman, K., Pelkey, E. T., Steinman, L., and Rothbard, J. B. (2000). The Design, Synthesis, and Evaluation of Molecules that Enable or Enhance Cellular Uptake: Peptoid Molecular Transporters. Proc. Natl. Acad. Sci. 97 (24), 13003–13008. doi:10.1073/pnas.97.24.13003
Wright, L., Rothbard, J., and Wender, P. (2003). Guanidinium Rich Peptide Transporters and Drug Delivery. Cpps 4 (2), 105–124. doi:10.2174/1389203033487252
Xie, J., Bi, Y., Zhang, H., Dong, S., Teng, L., Lee, R. J., et al. (2020). Cell-Penetrating Peptides in Diagnosis and Treatment of Human Diseases: From Preclinical Research to Clinical Application. Front. Pharmacol. 11, 697. doi:10.3389/fphar.2020.00697
Yin, H., Huang, Y-H., Deprey, K., Condon, N. D., Kritzer, J. A., Craik, D. J., et al. (2020). Cellular Uptake and Cytosolic Delivery of a Cyclic Cystine Knot Scaffold. ACS Chem. Biol. 15 (6), 1650–1661. doi:10.1021/acschembio.0c00297
Yoo, B., and Kirshenbaum, K. (2008). Peptoid Architectures: Elaboration, Actuation, and Application. Curr. Opin. Chem. Biol. 12 (6), 714–721. doi:10.1016/j.cbpa.2008.08.015
Yoo, B., Shin, S. B. Y., Huang, M. L., and Kirshenbaum, K. (2010). Peptoid Macrocycles: Making the Rounds with Peptidomimetic Oligomers. Chem. - A Eur. J. 16 (19), 5528–5537. doi:10.1002/chem.200903549
Zhang, Y., Zhang, H., Ghosh, D., and Williams, R. O. (2020). Just How Prevalent Are Peptide Therapeutic Products? A Critical Review. Int. J. Pharmaceutics 587, 119491. doi:10.1016/j.ijpharm.2020.119491
Zuckermann, R. N., Kerr, J. M., Kent, S. B. H., and Moos, W. H. (1992). Efficient Method for the Preparation of Peptoids [Oligo(N-Substituted Glycines)] by Submonomer Solid-phase Synthesis. J. Am. Chem. Soc. 114 (26), 10646–10647. doi:10.1021/ja00052a076
Keywords: peptoid, molecular transport, cyclization, peptidomimetic, amide
Citation: Herlan CN, Meschkov A, Schepers U and Bräse S (2021) Cyclic Peptoid-Peptide Hybrids as Versatile Molecular Transporters. Front. Chem. 9:696957. doi: 10.3389/fchem.2021.696957
Received: 18 April 2021; Accepted: 07 June 2021;
Published: 25 June 2021.
Edited by:
Laura Zaccaro, Institute of Biostructure and Bioimaging, ItalyReviewed by:
Annelise Emily Barron, Stanford University, United StatesYuji Nishiuchi, GlyTech, Inc., Japan
Copyright © 2021 Herlan, Meschkov, Schepers and Bräse. This is an open-access article distributed under the terms of the Creative Commons Attribution License (CC BY). The use, distribution or reproduction in other forums is permitted, provided the original author(s) and the copyright owner(s) are credited and that the original publication in this journal is cited, in accordance with accepted academic practice. No use, distribution or reproduction is permitted which does not comply with these terms.
*Correspondence: Stefan Bräse, QnJhZXNlQGtpdC5lZHU=