- 1Institute for Advanced Study, Shenzhen University, Shenzhen, China
- 2Institute of Microscale Optoelectronics, Shenzhen University, Shenzhen, China
- 3Crystal Growth and Thin Film Laboratory, Department of Physics and Nanotechnology, SRM Institute of Science and Technology, Kancheepuram, India
Semiconducting nanoparticles (SC NPs) play vital roles in several emerging technological applications including optoelectronic devices, sensors and catalysts. Recent research focusing on the single entity electrochemistry and photoelectrochemistry of SC NPs is a fascinating field which has attained an increasing interest in recent years. The nano-impact method provides a new avenue of studying electron transfer processes at single particle level and enables the discoveries of intrinsic (photo) electrochemical activities of the SC NPs. Herein, we review the recent research work on the electrochemistry and photoelectrochemistry of single SC NPs via the nano-impact technique. The redox reactions and electrocatalysis of single metal oxide semiconductor (MOS) NPs and chalcogenide quantum dots (QDs) are first discussed. The photoelectrochemistry of single SC NPs such as TiO2 and ZnO NPs is then summarized. The key findings and challenges under each topic are highlighted and our perspectives on future research directions are provided.
Introduction
Semiconductor nanomaterials have attracted increasing interest due to their excellent physical and chemical properties comparing with their bulk counterparts, such as continuous absorption bands, narrow and intensive emission spectra, high chemical and photo-bleaching stability, processability and surface functionality, which make them suitable candidates in single electron devices, sensors, imaging devices, solar cells, nano-electronics, optoelectronic devices and memory devices (Itoh et al., 1988; Wang and Herron, 1991; Bhargava et al., 1994; John and Singh, 1996; Singh and John, 1997). Electrochemical and photo-electrochemical studies of semiconductor nanomaterials are important for understanding the mechanism and kinetics of the relevant processes in their applications. Macroscopic (ensemble) measurements are still predominantly used to infer the underlying microscopic processes when investigating the (photo) electrochemistry of nanomaterials. However, this is not always straightforward or fully representative. A large population of nanoparticles may result in polydispersity, different particle orientations, the formation of the likely agglomerated and irregular “mat” associated with the dropcast technique and other phenomena that make the results difficult to interpret. A greater mechanistic understanding of the electrochemical and photo-electrochemical reactivity of nanomaterials requires the development of method for probing electron transfer events on single redox nanomaterials and individual catalytic entities. One of these emerging methods is the “nano-impact” approach (or particle-electrode impacts) which is realising an entirely fresh way of studying nanoparticles, their reactivity and catalytic properties, transforming the science of nano-chemistry (Cheng and Compton, 2014; Pumera, 2014; Rees, 2014; Sokolov et al., 2017). The phenomenon of “nano-impact” method, due to Brownian collisions of NPs with an electrode held at a suitable potential, enables NPs to be individually electro-reduced or oxidised, or mediate (catalyze) charge transfer processes which are not viable on the microelectrode itself under the conditions of study. This powerful electrochemical technique has found much strength in giving insights into the fundamental study of nanoparticles: not only the basic particle characterization (e.g. sizing, concentration, chemical identity, agglomeration/aggregation state, porosity) (Zhou et al., 2011; Stuart et al., 2012; Tschulik et al., 2014; Jiao et al., 2017; Li et al., 2019), but also in-depth understanding at single-particle levels for the mechanisms and dynamics of (photo) electrochemical processes of interest (Xiao and Bard, 2007; Bard et al., 2010; Fernando et al., 2013; Li et al., 2016; Ma et al., 2018; Peng et al., 2018).
The stochastic electrochemistry “nano-impact” was born since Lemay’s report in 2004, where the collision of single latex microspheres on (ultramicroelectrode) UMEs was discussed (Quinn et al., 2004). Research at early stage mainly include the works by Quinn et al., 2004 and Xiao and Bard, 2007 on insulating and metallic NPs. The motivation for studies of semiconducting (SC) NPs starts from the interest in investigating photoelectrochemical currents for energy conversion in SC nanostructures. Since then, single entity studies on the semiconducting materials such as metal oxides and some quantum dots materials have been performed (Sardesai et al., 2013; Tschulik, et al., 2013; Perera et al., 2015; Fernando et al., 2015; Shimizu et al., 2017; Alshalfouh et al., 2019; Karunathilake et al., 2020; Wang et al., 2021). The overarching goal of studying single SC (photo) electrochemistry is to understand the net (photo) electrochemistry catalytic process at single particle level and to establish their instinct activity-structure relationship. Moreover, the mechanistic investigation on (photo) electrochemistry of individual NPs will contribute to understand and ultimately control the charge transfer at nanoscale. Even though several reports and reviews have summarised single SC photo-electrochemistry (Peng et al., 2018; Barakoti et al. (2016); Ma et al., 2018; Alpuche-Aviles et al., 2019; Wang et al., 2019; Wang et al., 2020), their electrochemical redox behavior and electro-catalytical activities were not comprehensively included. Therefore, our distinct aim of the mini review is to facilitate insight into both the electrochemistry and photoelectrochemistry of single semiconducting nanoparticles. A summarized data collection containing the electrochemistry and photoelectrochemistry of single entity semiconducting materials via nano-impact approach is presented in Table 1.
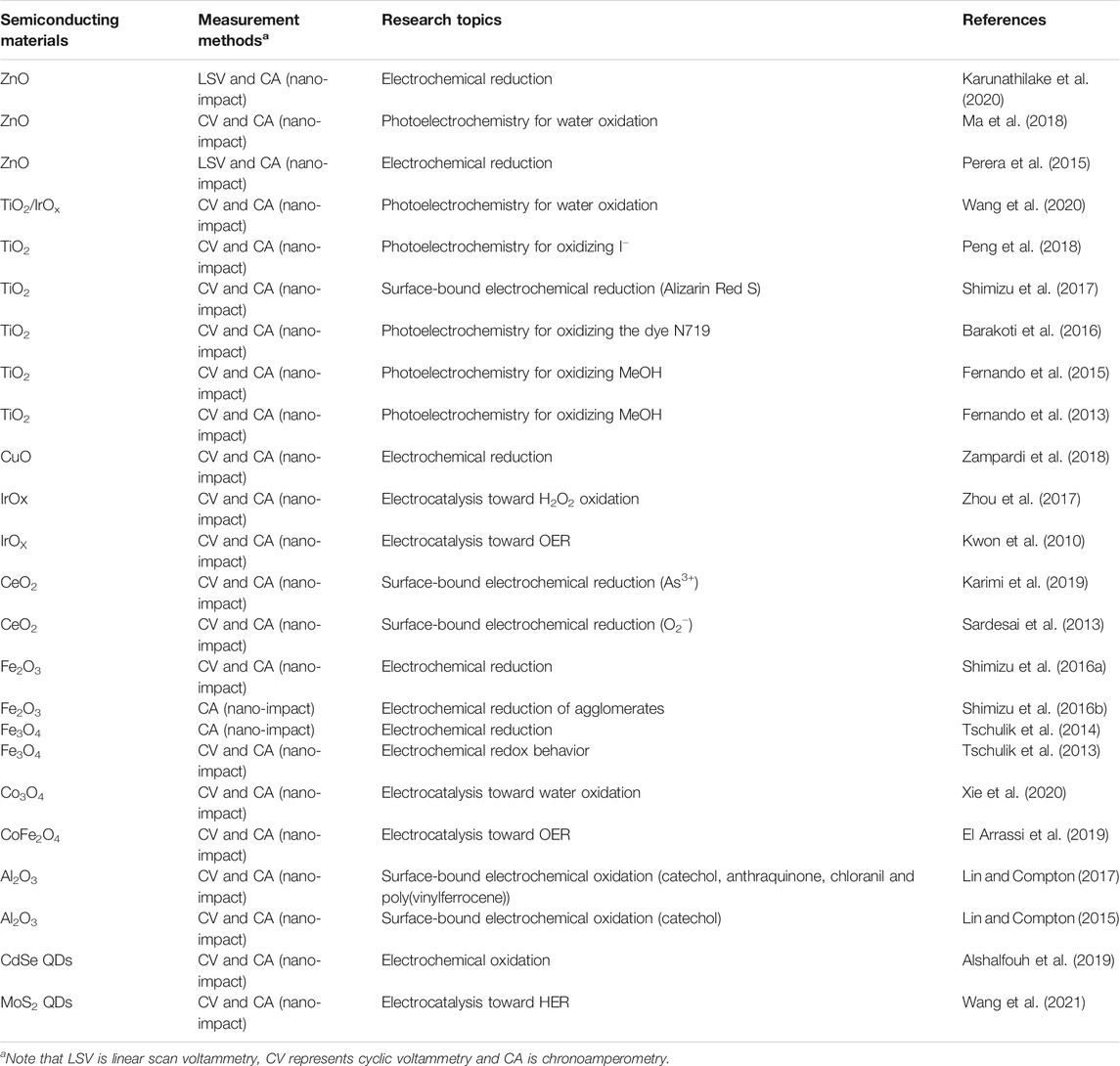
TABLE 1. Literature on single entity electrochemistry and photoelectrochemistry of semiconducting nanoparticles based on the nano-impact method.
Single Entity Electrochemistry of Semiconducting Nanoparticles
Single Entity Electrochemistry of Metal Oxides Nanoparticles
As an important type of semiconducting materials, metal oxide semiconductors (MOS) attain great attention due to its morphological versatility, chemical stability, physicochemical interfacial properties and their ability to combine in composite structures (Santos et al., 2016; Liu et al., 2017; Dong et al., 2017; Zhang et al., 2020). The earliest electrochemical studies on MOS usually focus on the average behavior via ensemble measurements at a macroelectrode with a few milli-meters in diameter, some of which involve the simultaneous collision of the suspended colloids with the electrode surface (Dunn et al., 1981a; Dunn et al., 1981b; Kamat, 1985; Koelle et al., 1985; Gratzel and Frank, 1982) For example, polarography and voltammetry of aqueous SnO2 and TiO2 suspensions was investigated by Heyrovsky et al., in 1995 (Heyrovsky et al., 1995a; Heyrovsky et al., 1995b). Even though collision and adsorption of nanoparticles can take place on a macroelectrode, exceedingly high frequencies of collision and huge background signals associated with the large electrode area make it challenging to individually resolve the collision events. When a microelectrode is employed, both the collision frequency and baseline noise are greatly reduced, thereby resulting in clear resolution of single impact events. With the development of this nano-impact technique, understanding the property and activity of single MOS are attracting increasing interests. In recent years, the electrochemical behavior of single MOS including IrOx, Fe2O3, Fe3O4, CeO2, CuO, Co3O4, TiO2, ZnO and CoFe2O4 etc. has been investigated via nano-impact method (Sardesai et al., 2013; Tschulik and Compton, 2014; Shimizu et al., 2016a; Shimizu et al., 2016b; Zhou et al., 2017; Zampardi, et al., 2018; Peng et al., 2018; Xie et al., 2020; Karunathilake et al., 2020) The specific research topics mainly involve two aspects: the electrochemical redox behavior and electrocatalysis of single MOS, which is summarized and discussed in the following paragraphs respectively. For a direct type of nano-impact, the nanoparticles are usually fully oxidized (or reduced) so that the electrolysis of the nanoparticles is quantitative. The charge (Coulombs) obtained by integrating the current transients reflects (via Faraday’s first Law) the number of atoms (or molecules) in the nanoparticle thus giving its size. A large number of current spikes are easily and rapidly measured so giving a particle size distribution. The method is capable of sizing nanoparticles as small as 5 nm with suitably sensitive home-built but inexpensive equipment (Batchelor-McAuley et al., 2015). In addition to sizing nanoparticles the nano-impact technique is able to simultaneously measure their concentration via the frequency of the observed impacts (Stuart et al., 2012). Furthermore, the potentials at which the current spikes onset are clearly related to the chemical identity of the nanoparticles and recent work has shown that it is able to measure the states of agglomeration/aggregation of the particles (Tschulik et al., 2014) and the porosity of the particles (Jiao et al., 2017). Currently, the direct nano-impact studies on MOS mainly focus on the redox behavior of the MOS themselves Fe3O4 (Tschulik and Compton, 2014), Fe2O3 (Shimizu et al., 2016a; Shimizu et al., 2016b), ZnO (Perera et al., 2015; Karunathilake et al., 2020; Ma et al., 2018) and CuO (Zampardi et al., 2018) and the MOS surface-bound with electroactive species CeO2 (Karimi et al., 2019), TiO2 (Shimizu et al., 2017) and Al2O3 (Lin and Compton 2015, 2017).
Tschulik et al. conducted cathodic and anodic impact experiments for Fe3O4 nanoparticles and opened two independent routes to electrochemical sizing the particles (Figure 1A) (Tschulik et al., 2013). Furthermore, individual Fe3O4 NPs in the presence and absence of a magnetic field was investigated and a significant magnetic field-induced agglomeration of NPs is observed in a magnetic field. More interestingly, dissolution of Fe3O4 NPs is found to be strongly inhibited in a magnetic field; this is likely due to the magnetic field gradient force trapping the produced Fe2+ ions near the NP surface and hence hindering the mass-transport controlled NP dissolution (Tschulik et al., 2014). Shimizu et al. (2016b) achieved the electrochemical sizing for Fe2O3 NPs via conducting reductive impact experiments. It is found that the first electron transfer process is the rate determining step of the reductive dissolution of nanoparticles, and the interfacial proton concentration has strong effect on the overall process (Shimizu et al., 2016a; Shimizu et al., 2016b). A following work demonstrated the rapid and reversible agglomeration/dis-agglomeration process of Fe2O3 NPs and opens a new way of investigating the agglomeration equilibria of mineral nanoparticles in aquatic media (Shimizu et al., 2016a; Shimizu et al., 2016b; Shimizu et al., 2016). Perera et al. investigated the impact experiments of ZnO nanoparticles (NPs) and realised the determination of the redox potential for the ZnO NPs. It is observed that the formal potential is a strong function of NP size (1/r) since smaller NPs are less stable compared to the larger ones and hence relatively easier to be reduced. (Perera et al., 2015). Further understanding on the reduction kinetics and mass transport of ZnO single entities was reported by Karunathilake et al. (Karunathilake et al., 2020). Recently, to understand the environmental fate of CuO nanoparticles and further correctly assess their toxicity, Zampardi et al. employed the nano-impact method to investigate the electrochemical behavior of single copper oxide nanoparticles in the presence of anionic species (Cl− and NO3−) commonly found in real water media. The potentials of in-situ detecting the nanoparticles in real world media is demonstrated (Zampardi et al., 2018).
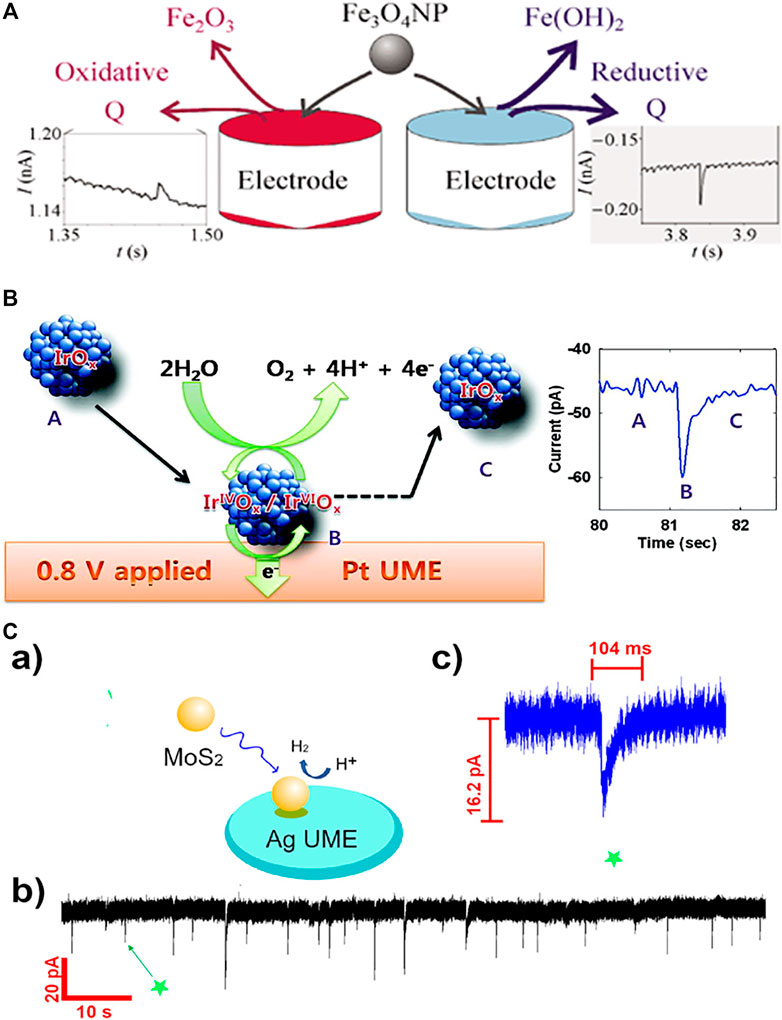
FIGURE 1. (A) The nano-impact measurements of single Fe3O4 magnetite nanoparticles using both anodic particle coulometry (APC) and cathodic particle coulometry (CPC) to independently get the size information of Fe3O4 nanoparticles (Tschulik et al., 2013). Copyright © 2013 Springer (B) Schematic illustration of single IrOx NP collision event and the current enhanced by electrocatalytic water oxidation (Kwon et al., 2010). Copyright © 2010 American Chemical Society (C) (a) Scheme of single MoS2 QD collision at the Ag UME surface; the reaction is switched on when the particle is in contact with the detection electrode. (b) Experimentally obtained current transient applied by Ag UME (diameter 10 μm) held at −700 mV vs SHE. (c) Representative current profile observed in a single QD collision event indicated in (b).Wang et al. (2021). Copyright © 2021 American Chemical Society.
In addition to the redox behavior of single MOS, the MOS absorbed with active species have also been investigated at single particle level. Based on the nano-impact method, Sardesai et al. studied the reduction of surface bound oxygen species on single CeO2 NPs and evaluated antioxidant activity of CeO2 NPs in a simple, rapid and inexpensive approach (Sardesai et al., 2013). Recently, the oxidation and reduction of As3+ loaded CeO2 NPs was reported by using collision electrochemistry and the highest spike frequency at pH 8 suggesting a maximum adsorption capacity. The As concentration in solution was determined by deriving from the measured charge and peak current, as well as the spike frequency (Karimi et al., 2019). In addition, the nano-impact is employed to probe the degree of cluster formation of Alizarin Red S modified rutile (TiO2) nanoparticles based on the reduction of the adsorbed dye molecules (Shimizu et al., 2017). Lin and Compton reported the quantification of the adsorbed catechol on single Al2O3 particles and the individual impact spikes resulting from the catechol oxidation was modeled to derive the charge diffusion coefficient across the particle surface (Lin and Compton, 2015). Adsorption of more redox active species including catechol, anthraquinone, chloranil and poly(vinylferrocene) on alumina particles was also investigated and their surface coverages and charge diffusion coefficients were determined respectively (Lin and Compton, 2017).
The investigation on redox behavior of single MOS and their absorption of redox active species discovers more accurate physiochemical properties for both MOS and the adsorbed redox species. As an alternative to the direct redox reaction of the nanoparticles themselves, mediated (indirect) electron transfer can also take place on the surface of impacting nanoparticles. When catalytically active nanoparticles stochastically collide with an inert microelectrode in a solution of redox molecules, transient current increases may be observed due to enhanced catalytic activity on nanoparticle surfaces. The current response of these collisions may adopt one of two general forms: a current spike or a current step, depending on a few complex factors such as the residence time of the impacting catalytic nanoparticles on the electrode surface and if the deactivation time of nanoparticle. If the desorption or deactivation of the nanoparticle is relatively slow compared to the experimental time, a “step on” in the current-time response is observed, otherwise a “spike” obtained. Some significant applications of the mediated electron transfer for MOS have been reported, examples cover IrOx, Fe2O3, Co3O4 and CoFe2O4 NPs. (Zhou et al., 2017; Kwon et al., 2010; Shimizu et al., 2016a,b; Xie et al., 2020; El Arrassi et al., 2019). The initial studies on the mediated impacts of MOS reported the enhanced current transients from the electrocatalysis of individual impacting IrOx NPs toward oxygen evolution reaction (OER). A current decay rather than steady response was observed, and the obtained current is found to be highly sensitive to the material and surface state of the electrode used (Figure 1B) (Kwon et al., 2010). Coupled with microscopic investigation, the current transients from oxidation of hydrogen peroxide at single IrOx NP further discovers the origins of deactivation of catalytic NPs and the factors affecting the collision dynamics (Zhou et al., 2017). Recently Xie et al. reported the water oxidation on single Co3O4 nanoparticles, a mechanism involving hydrogen peroxide as the initial oxidation product of electron transfer and a following decomposition to form dioxygen was proposed. Single particle electrocatalysis points out the rate-determining step and the limiting kinetics of the reaction (Xie et al., 2020). El Arrassi et al. investigated the OER on single CoFe2O4 NPs and revealed that the current density at single nanoparticle researches as high as several kA·m−2. The analysis of the steady-state current further indicates that the electrocatalytic activity is limited by the diffusion of produced oxygen away from the particle, providing new insights into intrinsic activities of the nanocatalysts (El Arrassi et al., 2019).
Overall, the single entity electrochemistry of MOS covering the redox behavior and electrocatalysis of single MOS has been investigated via the nano-impact technique. The latter has shown the capability to reveal the fundamental physiochemical properties of nanoparticles (sizing, concentration, agglomeration/aggregation state), and to provide in-depth understanding the mechanisms and dynamics of electrochemical processes at nanoscale. However, more investigation on the redox behavior of single MOS in complicate aqueous media is required for finally realising the in-situ electrochemical determination and analysis of solution phase MOS in real world environment. Single entity electrocatalysis should be extended to more metal oxide based electro-catalysts for better understanding the underlying mechanism and kinetics of the important reactions.
Single Entity Electrochemistry of Semiconducting Quantum Dots
In addition to the MOS, semiconducting quantum dots (QDs), especially chalcogenide QDs hold unique optical and electrical properties such as narrow emission absorption and high photo-stability, making them increasingly popular in recent years in the applications of optoelectronic devices, catalysis (Barakat et al., 2005), bio-labeling (Lin et al., 2007), lasers (Supran et al., 2013), sensors (Cho et al., 2015), LEDs (Yang et al., 2015) and photovoltaics (Yang et al., 2017). The research on single entity electrochemistry of QDs starts very recently with the report of CdSe/CdS QDs by Alshalfouh et al., 2019. Together with fluorescence correlation spectroscopy (FCS), electrochemical impact measurements was carried out to understand the reactivity as well as dynamics of CdSe/CdS QDs at a Pt microelectrode surface. The latter was around 1 μm in diameter for matching the size of the optical observation volume. Cyclic voltammetry was used to investigate the oxidation of CdSe/CdS QDs with negatively charged shells. Significantly, it is found that the electro-oxidized QDs are still able to emit light although the emission lifetime decreases. According to this report, different from the widely reported metal NPs in single collision experiment, more collision events are likely required for a small QD to have a complete anodic decomposition (Alshalfouh et al., 2019). According to this report, multiple collision events are required for a QD to have a complete anodic decomposition. What’s more, the intrinsic electrocatalytic activity of single MoS2 quantum dots (QDs) toward HER is just reported by Wang et al. (Figure 1C) (Wang et al., 2021). The current responses obtained at silver ultramicroelectrodes (UMEs) and carbon UMEs were recorded respectively. Current “spikes” with much higher current intensity is observed at Ag UMEs while “steps” obtained at C UMEs, revealing the influence of the substrate-MoS2 interface on HER activity. Very few reports on electrochemistry of single QDs so far is likely due to the following reasons: the small size of the QDs (a few nanometers in diameter) increases the difficulties to be electrochemically detected with the current low-noise potentiostats; the semiconducting nature of the QDs make the electron transfer process more complicate than the metal nanoparticles; the surface chemistry of the QDs imposed uncertainties to the collision process with the electrode surface.
Single Entity Photoelectrochemistry of Semiconducting Nanoparticles
Semiconductor photoelectrochemistry deals with solar energy conversion to electricity or chemical fuels and focuses on the photo-driven reactions at solid/liquid interfaces. To achieve the practical energy conversion applications, the fundamentals of SC nanomaterials such as charge carrier generation, separation, transport, and especially interfacial charge transfer at heterogeneous nanoscale interfaces need to be clarified. Single entity photoelectrochemistry of colloidal bared and sensitized TiO2, and ZnO NPs has been reported so far (Fernando et al., 2013; Fernando et al., 2015; Barakoti et al., 2016; Ma et al., 2018; Peng et al., 2018; Wang et al., 2020). Fernando et al. employed the nano-impact method to detect the stochastic photoelectrochemical currents of individual anatase TiO2 nanoparticles. It is reported that the current steps were not observed for NP suspension under illumination in MeCN while the steps appeared in MeOH. This is due to that NPs under illumination produce valence-band holes that oxidize MeOH. They have also investigated the photoelectrochemical behaviour of the NP with different diameter and reported that the collisions resulting in a current step could be smaller for the semiconductor NP than the metal NPs (Fernando et al., 2013). Furthermore, it has been demonstrated that the sensitivity of the detection can be improved by using a dye (Fernando et al., 2015; Barakoti et al., 2016; Shimizu et al., 2017; Ma et al., 2018; Peng et al., 2018). Specifically, Barakoti et al. (2016) reported the study of dye-sensitized nanoparticles and their agglomerates with stochastic electrochemistry, and under illumination the cathodic steps are observed because of the photo-oxidation of the dye which injects electrons into the TiO2 NP and yields the oxidized dye molecules at particle surface (Barakoti et al., 2016). Peng et al. (2018) developed an ultrasensitive photoelectrochemical method for detecting the photocurrent from single SC nanoparticles by using micrometer-thick nanoparticulate TiO2 filmed Au ultramicroelectrode (TiO2@AuUME). The presence of di-tetrabutylammonium cis-bis(isothiocyanato) bis(2,2′-bipyridyl-4,4′-dicarbox-ylato) ruthenium(II) (N719) makes the TiO2 NPs collect more photons and hence increases photoelectrochemical current. They have reported that the electron transfer into the nano-particulate TiO2 film will takes place when the individual N719@TiO2 nanoparticles stochastically collide with TiO2@AuUME (Figure 2A). Coupled with theoretical simulation, the high-resolution photocurrent measurement provides the quantification of electron transfer of single N719@TiO2 nanoparticle and the further estimation of the electron diffusivity of TiO2@AuUME (Peng et al., 2018). The developed protocol was further used to investigate the photo-electrochemical behavior of single N719@ZnO entity on an Au ultramicroelectrode with different TiO2 film thicknesses (Ma et al., 2018). The photocatalytic properties of N719 at single ZnO entity were quantified, and the influence of the film thickness on the electron transport behavior was estimated with the help of simulation. The latter is in agreement with the experimental results, indicating the successful quantification of single SC NPs photoelectrochemistry. Wang et al. demonstrated the light controlled single nanoparticle collision experiments for Pt@TiO2 NPs with carbon UME and IrOx NPs with a Nb:TiO2 (110) rutile single crystal UME (Figure 2C). The Pt@TiO2 NPs was in-situ electrochemically deposited and their collisions with a carbon UME as the light guide were catalytically amplified by the oxygen evolution reaction (OER). Current blips due to the collision events was observed. In addition, light-controlled collisions of IrOx NPs with a Nb:TiO2 (110) rutile single crystal UME showed photoelectrocatalytic activity of this semiconductor/cocatalyst system. The current spikes can be used to indicate the activities of individual IrOx NPs. Larger current spikes for IrOx NPs than the Pt NPs and Pt@TiO2 NPs are associated with the higher electro-catalytical activity of IrOx NPs toward OER (Wang et al., 2020). Overall, the research on single SC photoelectrochemistry are still very limited and only two types of MOS nanoparticles have been investigated. The research objects are highly worthy to be extended so that some general detection strategies could be developed. In addition, more study on the photocurrent behavior of single SC NPs with different properties is required to better understand their intrinsic performance-structure relationships.
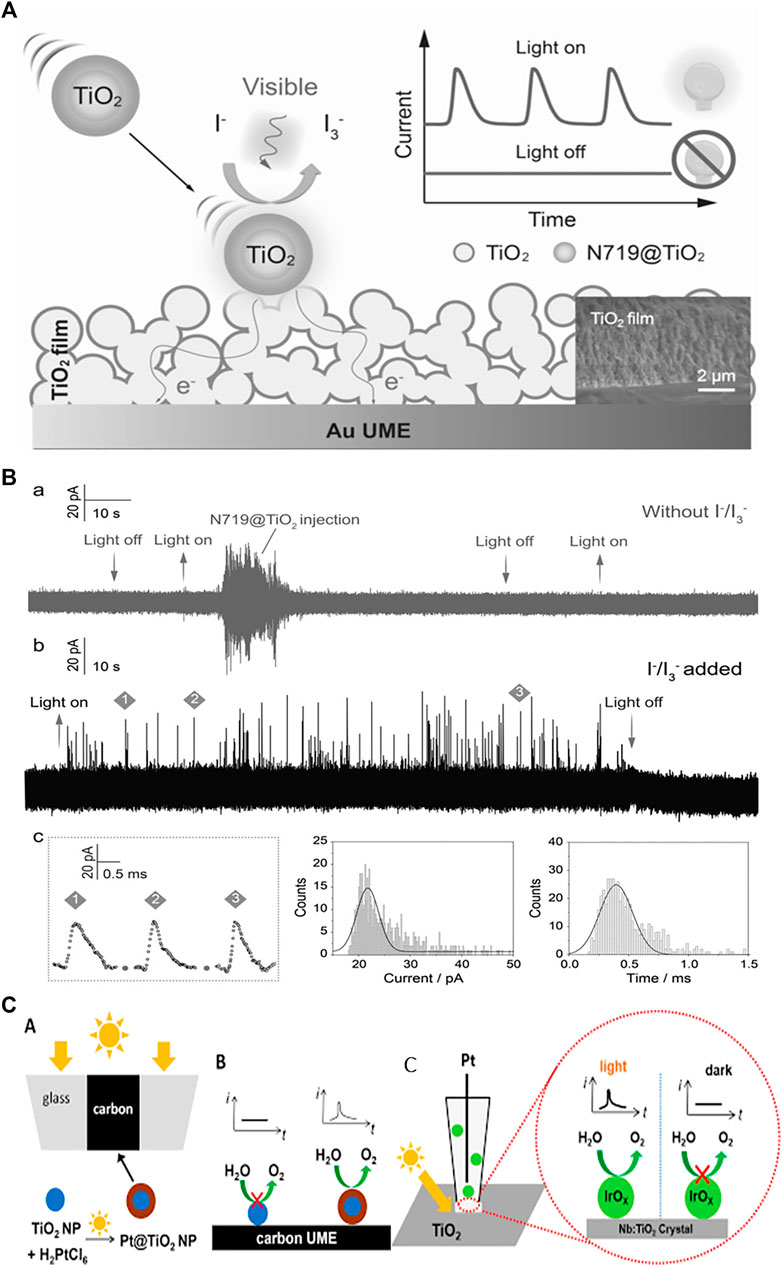
FIGURE 2. (A) Illustration of the photoelectrochemical behavior of a single N719@TiO2 nanoparticle during a collision with a TiO2@Au UME in the presence of I−/I3− redox electrolyte under visible light, thus generating spike like current transients. (B) Amperometric current–time curves of individual N719@TiO2 nanoparticles at +600 mV vs. Ag/AgCl in the absence (a) and in the presence (b) of the I−/I3− redox couple in the electrolyte solution under illumination with a Xe lamp (λ > 450 nm). (c) Expanded portions of the representative photocurrent traces. Histogram of the peak currents and duration time of individual transients. Peng et al. (2018). Copyright © 2018 John Wiley and Sons (C) Light controlled NP collisions: Schematic representation of (A)in Situ Photosynthesis of Pt@TiO2 NPs (B) their catalytically amplified collisions with the carbon UME and (C) Photoelectrochemically amplified collisions of IrOx NPs with a microscopic portions of the Nb doped n-type TiO2 (110) Rutile single crystal surface facing the micropipette orifice Wang et al. (2020). Copyright © 2020 American Chemical Society.
Conclusion
In this mini review the recent research work on the electrochemistry and photoelectrochemistry of single semiconducting nanoparticles are summarized. The redox reactions and electrocatalysis of single metal oxide semiconductor NPs and quantum dots via the nano-impact method are discussed. The technique enables both the discoveries of the fundamental physiochemical properties of nanoparticles, and in-depth understanding for the mechanisms and dynamics of (photo) electrochemical processes at single particle levels. However, currently the nano-impact methodology faces some general challenges such as lack of deeper understanding on the dynamics of NPs during the collision process and how different factors have effect on it. For the SC NPs, the substrate-NPs interface plays far more important roles in the electron transfer process due to their semiconducting nature. The influencing factors likely include the electrode materials, surface chemistry of NPs and media composition, which are still poorly understood to date and need to be carefully investigated and clarified in future. Furthermore, the research on single SC NP photoelectrochemistry is still at early stage and the research objects are highly worthy to be extended so that some general detection and analysis strategies could be developed and the intrinsic (photo) electrochemical activity-structure relationships for more SC NPs could be revealed.
Author Contributions
SM was responsible for database regarding ZnO, TiO2, SnO2 etc. and drafted the manuscript. XL supervised, critically reviewed, edited and finalized the manuscript for submission. All other authors YZ and MM attended part of the work and provided some beneficial advices and discussions on this work.
Funding
The authors acknowledge the grants from the Natural Science Foundation of SZU (860-000002110386), Regional Joint Fund of Guangdong Province (2019A1515111054) and National Natural Science Foundation of China (22004085).
Conflict of Interest
The authors declare that the research was conducted in the absence of any commercial or financial relationships that could be construed as a potential conflict of interest.
References
Alpuche-Aviles, M. A., Gutierrez-Portocarrero, S., and Barakoti, K. K. (2019). Challenges in Semiconductor Single-Entity Photoelectrochemistry. Curr. Opin. Electrochemistry 13, 174–180. doi:10.1016/j.coelec.2018.12.007
Alshalfouh, A., Oezaslan, M., Dosche, C., and Wittstock, G. (2019). Electrochemistry of CdSe Quantum Dots Studied by Single Molecule Spectroscopy. ChemElectroChem 6, 1884–1893. doi:10.1002/celc.201801793
Barakat, M. A., Schaeffer, H., Hayes, G., and Ismat-Shah, S. (2005). Photocatalytic Degradation of 2-chlorophenol by Co-doped TiO2 Nanoparticles. Appl. Catal. B: Environ. 57, 23–30. doi:10.1016/j.apcatb.2004.10.001
Barakoti, K. K., Parajuli, S., Chhetri, P., Rana, G. R., Kazemi, R., Malkiewich, R., et al. (2016). Stochastic Electrochemistry and Photoelectrochemistry of Colloidal Dye-Sensitized Anatase Nanoparticles at a Pt Ultramicroelectrode. Faraday Discuss. 193, 313–325. doi:10.1039/C6FD00100A
Bard, A. J., Zhou, H., and Kwon, S. J. (2010). Electrochemistry of Single Nanoparticles via Electrocatalytic Amplification. Isr. J. Chem. 50, 267–276. doi:10.1002/ijch.201000014
Batchelor-Mcauley, C., Ellison, J., Tschulik, K., Hurst, P. L., Boldt, R., and Compton, R. G. (2015). In Situ nanoparticle Sizing with Zeptomole Sensitivity. Analyst 140, 5048–5054. doi:10.1039/C5AN00474H
Bhargava, R. N., Gallagher, D., and Welker, T. (1994). Doped Nanocrystals of Semiconductors - a New Class of Luminescent Materials. J. Lumin. 60-61, 275–280. doi:10.1016/0022-2313(94)90146-5
Cheng, W., and Compton, R. G. (2014). Electrochemical Detection of Nanoparticles by ‘nano-Impact' Methods. Trac Trends Anal. Chem. 58, 79–89. doi:10.1016/j.trac.2014.01.008
Cho, H., Kwak, J., Lim, J., Park, M., Lee, D., Bae, W. K., et al. (2015). Soft Contact Transplanted Nanocrystal Quantum Dots for Light-Emitting Diodes: Effect of Surface Energy on Device Performance. ACS Appl. Mater. Inter. 7, 10828–10833. doi:10.1021/acsami.5b01738
Dong, S., Tong, M., Zhang, D., and Huang, T. (2017). The strategy of nitrite and immunoassay human IgG biosensors basedon ZnO@ZIF-8 and ionic liquid composite film. Sens. Actuat. B-Chem. 251, 650–657. doi:10.1016/j.snb.2017.05.047
Dunn, W. W., Aikawa, Y., and Bard, A. J. (1981a). Characterization of Particulate Titanium Dioxide Photocatalysts by Photoelectrophoretic and Electrochemical Measurements. J. Am. Chem. Soc. 103, 3456–3459. doi:10.1021/ja00402a033
Dunn, W. W., Aikawa, Y., and Bard, A. J. (1981b). Semiconductor Electrodes: XXXV. Slurry Electrodes Based on Semiconductor Powder Suspensions. J. Electrochem. Soc. 128, 222–224. doi:10.1149/1.2127378
El Arrassi, A., Liu, Z., Evers, M. V., Blanc, N., Bendt, G., Saddeler, S., et al. (2019). Intrinsic Activity of Oxygen Evolution Catalysts Probed at Single CoFe2O4 Nanoparticles. J. Am. Chem. Soc. 141, 9197–9201. doi:10.1021/jacs.9b04516
Fernando, A., Chhetri, P., Barakoti, K. K., Parajuli, S., Kazemi, R., and Alpuche-Aviles, M. A. (2015). Transient Interactions of Agglomerates of Sensitized TiO2 Nanoparticles in Colloidal Suspensions. J. Electrochem. Soc. 163, H3025–H3031. doi:10.1149/2.0041604jes
Fernando, A., Parajuli, S., and Alpuche-Aviles, M. A. (2013). Observation of Individual Semiconducting Nanoparticle Collisions by Stochastic Photoelectrochemical Currents. J. Am. Chem. Soc. 135, 10894–10897. doi:10.1021/ja4007639
Graetzel, M., and Frank, A. J. (1982). Interfacial Electron-Transfer Reactions in Colloidal Semiconductor Dispersions, Kinetic analysis. J. Phys. Chem. 86, 2964–2967. doi:10.1021/j100212a031
Heyrovsky, M., Jirkovsky, J., and Mueller, B. R. (1995a). Polarography and Voltammetry of Aqueous Colloidal SnO2 Solutions. Langmuir 11, 4293–4299. doi:10.1021/la00011a021
Heyrovsky, M., Jirkovsky, J., and Struplova-Bartackova, M. (1995b). Polarography and Voltammetry of Aqueous Colloidal TiO2 Solutions. Langmuir 11, 4300–4308. doi:10.1021/la00011a022
Itoh, T., Iwabuchi, Y., and Kataoka, M. (1988). Study on the Size and Shape of CuCl Microcrystals Embedded in Alkali-Chloride Matrices and Their Correlation with Exciton Confinement. Phys. Stat. Sol. (B) 145, 567–577. doi:10.1002/pssb.2221450222
Jiao, X., Tanner, E. E. L., Sokolov, S. V., Palgrave, R. G., Young, N. P., and Compton, R. G. (2017). Understanding Nanoparticle Porosity via Nanoimpacts and XPS: Electro-Oxidation of Platinum Nanoparticle Aggregates. Phys. Chem. Chem. Phys. 19, 13547–13552. doi:10.1039/C7CP01737E
John, G. C., and Singh, V. A. (1996). Model for the Photoluminescence Behavior of Porous Silicon. Phys. Rev. B 54, 4416–4419. doi:10.1103/physrevb.54.4416
Kamat, P. V. (1985). Photoelectrochemistry in Colloidal Systems. Part 2.-A Photogalvanic Cell Based on TiO2 Semiconductor Colloid. J. Chem. Soc. Faraday Trans. 1 81, 509–518. doi:10.1039/F19858100509
Karimi, A., Andreescu, S., and Andreescu, D. (2019). Single-Particle Investigation of Environmental Redox Processes of Arsenic on Cerium Oxide Nanoparticles by Collision Electrochemistry. ACS Appl. Mater. Inter. 11, 24725–24734. doi:10.1021/acsami.9b05234
Karunathilake, N., Gutierrez‐Portocarrero, S., Subedi, P., and Alpuche‐Aviles, M. A. (2020). Reduction Kinetics and Mass Transport of ZnO Single Entities on a Hg Ultramicroelectrode. ChemElectroChem 7, 2248–2257. doi:10.1002/celc.202000031
Koelle, U., Moser, J., and Graetzel, M. (1985). Dynamics of Interfacial Charge-Transfer Reactions in Semiconductor Dispersions. Reduction of Cobaltoceniumdicarboxylate in Colloidal Titania. Inorg. Chem. 24, 2253–2258. doi:10.1021/ic00208a026
Kwon, S. J., Fan, F.-R. F., and Bard, A. J. (2010). Observing Iridium Oxide (IrOx) Single Nanoparticle Collisions at Ultramicroelectrodes. J. Am. Chem. Soc. 132, 13165–13167. doi:10.1021/ja106054c
Li, X., Batchelor-Mcauley, C., and Compton, R. G. (2019). Silver Nanoparticle Detection in Real-World Environments via Particle Impact Electrochemistry. ACS Sens. 4, 464–470. doi:10.1021/acssensors.8b01482
Li, X., Batchelor-Mcauley, C., Whitby, S. A. I., Tschulik, K., Shao, L., and Compton, R. G. (2016). Single Nanoparticle Voltammetry: Contact Modulation of the Mediated Current. Angew. Chem. Int. Ed. 55, 4296–4299. doi:10.1002/anie.201509017
Lin, C.-A. J., Liedl, T., Sperling, R. A., Fernández-Argüelles, M. T., Costa-Fernández, J. M., Pereiro, R., et al. (2007). Bioanalytics and Biolabeling with Semiconductor Nanoparticles (Quantum Dots). J. Mater. Chem. 17, 1343–1346. doi:10.1039/B618902D
Lin, Q., and Compton, R. G. (2017). Impacts Reveal and Quantify Monolayer Adsorption on Single Alumina Particles. Russ. J. Electrochem. 53, 994–1002. doi:10.1134/S1023193517090087
Lin, Q., and Compton, R. G. (2015). Quantifying Adsorption on Single Alumina Particles via Impact Voltammetry and Current Transient Analysis. J. Phys. Chem. C 119, 23463–23469. doi:10.1021/acs.jpcc.5b06419
Liu, H., Guo, K., Duan, C., Dong, X., and Gao, J. (2017). Hollow TiO2 Modified Reduced Graphene Oxide Microspheres Encapsulating Hemoglobin for a Mediator-free Biosensor. Biosens. Bioelectron. 87, 473–479. doi:10.1016/j.bios.2016.08.089
Ma, H., Ma, W., Chen, J.-F., Liu, X.-Y., Peng, Y.-Y., Yang, Z.-Y., et al. (2018). Quantifying Visible-Light-Induced Electron Transfer Properties of Single Dye-Sensitized ZnO Entity for Water Splitting. J. Am. Chem. Soc. 140, 5272–5279. doi:10.1021/jacs.8b01623
Peng, Y.-Y., Ma, H., Ma, W., Long, Y.-T., and Tian, H. (2018). Single-Nanoparticle Photoelectrochemistry at a Nanoparticulate TiO2 -Filmed Ultramicroelectrode. Angew. Chem. Int. Ed. 57, 3758–3762. doi:10.1002/anie.201710568
Perera, N., Karunathilake, N., Chhetri, P., and Alpuche-Aviles, M. A. (2015). Electrochemical Detection and Sizing of Colloidal ZnO Nanoparticles. Anal. Chem. 87, 777–784. doi:10.1021/ac5037445
Pumera, M. (2014). Impact Electrochemistry: Measuring Individual Nanoparticles. ACS Nano 8, 7555–7558. doi:10.1021/nn503831r
Quinn, B. M., Van't Hof, P. G., and Lemay, S. G. (2004). Time-resolved Electrochemical Detection of Discrete Adsorption Events. J. Am. Chem. Soc. 126, 8360–8361. doi:10.1021/ja0478577
Rees, N. V. (2014). Electrochemical Insight from Nanoparticle Collisions with Electrodes: A Mini-Review. Electrochemistry Commun. 43, 83–86. doi:10.1016/j.elecom.2014.03.018
Santos, L., Silveira, C. M., Elangovan, E., Neto, J. P., Nunes, D., Pereira, L., et al. (2016). Synthesis of WO3 Nanoparticles for Biosensing Applications. Sensors Actuators B: Chem. 223, 186–194. doi:10.1016/j.snb.2015.09.046
Sardesai, N. P., Andreescu, D., and Andreescu, S. (2013). Electroanalytical Evaluation of Antioxidant Activity of Cerium Oxide Nanoparticles by Nanoparticle Collisions at Microelectrodes. J. Am. Chem. Soc. 135, 16770–16773. doi:10.1021/ja408087s
Shimizu, K., Sokolov, S. V., and Compton, R. G. (2016a). Agglomeration Equilibria of Hematite Nanoparticles. Colloid Interf. Sci. Commun. 13, 19–22. doi:10.1016/j.colcom.2016.06.003
Shimizu, K., Sokolov, S. V., Young, N. P., and Compton, R. G. (2017). Particle-impact Analysis of the Degree of Cluster Formation of Rutile Nanoparticles in Aqueous Solution. Phys. Chem. Chem. Phys. 19, 3911–3921. doi:10.1039/C6CP08531H
Shimizu, K., Tschulik, K., and Compton, R. G. (2016b). Exploring the mineral-water Interface: Reduction and Reaction Kinetics of Single Hematite (α-Fe2O3) Nanoparticles. Chem. Sci. 7, 1408–1414. doi:10.1039/C5SC03678J
Singh, V. A., and John, G. C. (1997). Physics of semiconductor nanostructures (K.P. Jain). (New Delhi: Marosa Publishing House), p. 186.
Sokolov, S. V., Eloul, S., Kätelhön, E., Batchelor-Mcauley, C., and Compton, R. G. (2017). Electrode-particle Impacts: a Users Guide. Phys. Chem. Chem. Phys. 19, 28–43. doi:10.1039/C6CP07788A
Stuart, E. J. E., Zhou, Y.-G., Rees, N. V., and Compton, R. G. (2012). Determining Unknown Concentrations of Nanoparticles: the Particle-Impact Electrochemistry of Nickel and Silver. RSC Adv. 2, 6879–6884. doi:10.1039/C2RA20628E
Supran, G. J., Shirasaki, Y., Song, K. W., Caruge, J.-M., Kazlas, P. T., Coe-Sullivan, S., et al. (2013). QLEDs for Displays and Solid-State Lighting. MRS Bull. 38, 703–711. doi:10.1557/mrs.2013.181
Tschulik, K., Batchelor-Mcauley, C., Toh, H.-S., Stuart, E. J. E., and Compton, R. G. (2014). Electrochemical Studies of Silver Nanoparticles: a Guide for Experimentalists and a Perspective. Phys. Chem. Chem. Phys. 16, 616–623. doi:10.1039/C3CP54221A
Tschulik, K., and Compton, R. G. (2014). Nanoparticle Impacts Reveal Magnetic Field Induced Agglomeration and Reduced Dissolution Rates. Phys. Chem. Chem. Phys. 16, 13909–13913. doi:10.1039/C4CP01618A
Tschulik, K., Haddou, B., Omanović, D., Rees, N. V., and Compton, R. G. (2013). Coulometric Sizing of Nanoparticles: Cathodic and Anodic Impact Experiments Open Two Independent Routes to Electrochemical Sizing of Fe3O4 Nanoparticles. Nano Res. 6, 836–841. doi:10.1007/s12274-013-0361-3
Wang, H., Tang, H., and Li, Y. (2021). Intrinsic Electrocatalytic Activity of Single MoS2 Quantum Dot Collision on Ag Ultramicroelectrodes. J. Phys. Chem. C 125, 3337–3345. doi:10.1021/acs.jpcc.0c09644
Wang, L., Schmid, M., and Sambur, J. B. (2019). Single Nanoparticle Photoelectrochemistry: What Is Next? J. Chem. Phys. 151, 180901. doi:10.1063/1.5124710
Wang, Q., Bae, J. H., Nepomnyashchii, A. B., Jia, R., Zhang, S., and Mirkin, M. V. (2020). Light-Controlled Nanoparticle Collision Experiments. J. Phys. Chem. Lett. 11, 2972–2976. doi:10.1021/acs.jpclett.0c00585
Wang, Y., and Herron, N. (1991). Nanometer-sized Semiconductor Clusters: Materials Synthesis, Quantum Size Effects, and Photophysical Properties. J. Phys. Chem. 95, 525–532. doi:10.1021/j100155a009
Xiao, X., and Bard, A. J. (2007). Observing Single Nanoparticle Collisions at an Ultramicroelectrode by Electrocatalytic Amplification. J. Am. Chem. Soc. 129, 9610–9612. doi:10.1021/ja072344w
Xie, R. C., Volokhova, M., Boldin, A., Seinberg, L., Tsujimoto, M., Yang, M., et al. (2020). Electrocatalytic Oxidation of Hydroxide Ions by Co3O4 and Co3O4@SiO2 Nanoparticles Both at Particle Ensembles and at the Single Particle Level. ChemElectroChem 7, 1261–1276. doi:10.1002/celc.202000230
Yang, Y., Zheng, Y., Cao, W., Titov, A., Hyvonen, J., Manders, J. R., et al. (2015). High-efficiency Light-Emitting Devices Based on Quantum Dots with Tailored Nanostructures. Nat. Photon 9, 259–266. doi:10.1038/nphoton.2015.36
Yang, Z., Fan, J. Z., Proppe, A. H., Arquer, F. P. G. d., Rossouw, D., Voznyy, O., et al. (2017). Mixed-quantum-dot Solar Cells. Nat. Commun. 8, 1–9. doi:10.1038/s41467-017-01362-1
Zampardi, G., Thöming, J., Naatz, H., Amin, H. M. A., Pokhrel, S., Mädler, L., et al. (2018). Electrochemical Behavior of Single CuO Nanoparticles: Implications for the Assessment of Their Environmental Fate. Small 14, 1801765. doi:10.1002/smll.201801765
Zhang, B., Wang, H., Xi, J., Zhao, F., and Zeng, B. (2020). In situ formation of inorganic/organic heterojunction photocatalyst of WO3/Au/polydopamine for immunoassay of human epididymal protein 4. Electrochim. Acta 331, 135350. doi:10.1016/j.electacta.2019.135350
Zhou, M., Yu, Y., Hu, K., Xin, H. L., and Mirkin, M. V. (2017). Collisions of Ir Oxide Nanoparticles with Carbon Nanopipettes: Experiments with One Nanoparticle. Anal. Chem. 89, 2880–2885. doi:10.1021/acs.analchem.6b04140
Keywords: semiconducting nanoparticles, single entity, (photo) electrochemistry, metal oxides, quantum dots
Citation: Mathuri S, Zhu Y, Margoni MM and Li X (2021) Semiconducting Nanoparticles: Single Entity Electrochemistry and Photoelectrochemistry. Front. Chem. 9:688320. doi: 10.3389/fchem.2021.688320
Received: 30 March 2021; Accepted: 14 May 2021;
Published: 02 June 2021.
Edited by:
Qianjin Chen, Donghua University, ChinaReviewed by:
Weiguang Ma, Dalian National Laboratory for Clean Energy, Dalian Institute of Chemical Physics, Chinese Academy of Sciences, ChinaMing Zhou, Northeast Normal University, China
Copyright © 2021 Mathuri, Zhu, Margoni and Li. This is an open-access article distributed under the terms of the Creative Commons Attribution License (CC BY). The use, distribution or reproduction in other forums is permitted, provided the original author(s) and the copyright owner(s) are credited and that the original publication in this journal is cited, in accordance with accepted academic practice. No use, distribution or reproduction is permitted which does not comply with these terms.
*Correspondence: Xiuting Li, eGl1dGluZy5saUBzenUuZWR1LmNu