- 1Maragheh University of Medical Sciences, Maragheh, Iran
- 2Polymer Institute, Slovak Academy of Sciences, Bratislava, Slovakia
- 3Russian Institute for Advanced Study, Moscow State Pedagogical University, Moscow, Russian Federation
- 4Department of Surface Engineering, The John Paul II Catholic University of Lublin, Lublin, Poland
- 5Institute of Physics, Slovak Academy of Sciences, Bratislava, Slovakia
- 6Department of Nuclear Chemistry, Faculty of Natural Sciences, Comenius University in Bratislava, Bratislava, Slovakia
- 7Department of Biochemistry, Azerbaijan Medical University, Baku, Azerbaijan
- 8Kidney Research Center, Tabriz University of Medical Sciences, Tabriz, Iran
- 9Department of Chemistry and Biomolecular Science, Clarkson University, Potsdam, NY, United States
- 10Institute of Cell Biology, National Academy of Sciences of Ukraine, Lviv, Ukraine
- 11Department of Biophysics and Biochemistry, Baku State University, Baku, Azerbaijan
- 12Institute of Radiation Problems, National Academy of Sciences of Azerbaijan, Baku, Azerbaijan
- 13Department of Biology and Chemistry, Drohobych Ivan Franko State Pedagogical University, Drohobych, Ukraine
- 14Center of Experimental Orthopaedics, Saarland University Medical Center, Homburg, Germany
Liver fibrosis is a major cause of morbidity and mortality worldwide due to chronic liver damage and leading to cirrhosis, liver cancer, and liver failure. To date, there is no effective and specific therapy for patients with hepatic fibrosis. As a result of their various advantages such as biocompatibility, imaging contrast ability, improved tissue penetration, and superparamagnetic properties, magnetic nanoparticles have a great potential for diagnosis and therapy in various liver diseases including fibrosis. In this review, we focus on the molecular mechanisms and important factors for hepatic fibrosis and on potential magnetic nanoparticles-based therapeutics. New strategies for the diagnosis of liver fibrosis are also discussed, with a summary of the challenges and perspectives in the translational application of magnetic nanoparticles from bench to bedside.
Introduction
The unique intrinsic regenerative properties of the liver have long been known, reaching up to 70% in healthy liver tissue (Michalopoulos and Bhushan, 2021). In fact, it is the only organ with the capability of replacing potentially injured cells via regeneration processes in humans (Michalopoulos and Bhushan, 2021). However, in spite of such a feature, the prevalence of hepatic diseases exhibits an upward trend with a high rate of mortality.
Recurrent and chronic injuries may lead to liver fibrosis which frequently leads to cancer, with an enhanced accumulation of extracellular matrix (ECM) components like type-I and -III collagen, resulting in scar deposition and triggering the disease severity (Fan et al., 2020). The continuous accumulation of ECM disturbs several key functions mediated by the liver like detoxification and results in disturbances in the hepatic blood flow. Moreover, fibrosis is associated with the recruitment of inflammatory cytokines that can augment tumor development (Stolfi et al., 2021). If left untreated, cirrhosis may occur subsequently to fibrosis which is connected with portal hypertension, liver failure, hepatic encephalopathy, as well as the elevated risk of hepatocellular carcinoma (HCC) (Stolfi et al., 2021). Any stimulus that can disturb hepatic-sensitive cells can result in liver injury. Genetic factors and secondary liver damage due to the presence of other diseases such as cholestatic disorders, viral hepatitis, autoimmune diseases, and chronic alcohol abuse can also induce liver fibrosis. Nonalcoholic steatohepatitis (NASH) and nonalcoholic fatty liver disease (NALFD) stand for the main etiologies of the disease. Obesity and demographic alterations resulting from aging also increased the prevalence of hepatic fibrosis (Nascimbeni et al., 2020). NAFLD is considered the major cause of chronic liver diseases and the top indication of liver transplantation (Mikulic and Mrzljak, 2020). Other factors such as the infiltration of inflammatory cells can promote the progression of NASH, NAFLD, and fibrosis. The severity of fibrosis has been connected with the mortality associated with liver diseases as demonstrated in numerous longitudinal clinical investigations. Consistently, the efficiency for the assessment of pharmacological options against NAFLD is their effect on liver fibrosis that might also exert a positive outcome on non-hepatic disorders (Polyzos et al., 2020). Experts estimate that the mortality rate of liver-related diseases will significantly rise in the upcoming years (Mikulic and Mrzljak, 2020). Fibrosis may be characterized a dysregulated wound healing process, resulting in the generation of tissue scars (Simkin et al., 2020). The presence of bridging fibers between portal regions is the signs of advanced stages of liver fibrosis (Chang and Li, 2020).
The inability of conventional treatments to deliver adequate concentrations of drugs into the liver has restricted their application. Targeted delivery of pharmaceutics to the liver tissue via the application of nanotechnology has grabbed great attention in the recent years (Mikulic and Mrzljak, 2020; Nascimbeni et al., 2020; Polyzos et al., 2020). Different types nanoparticle systems have been utilized in the treatment of liver fibrosis. The unique specifications of these systems are due to their size, shape, structure and surface features. Polymers, liposomes, and distinct moieties have been used as nanomedicine formulations in the treatment of hepatic diseases. These systems have efficiently delivered different therapeuticall agents to the liver cells.
Magnetic nanoparticles (MNPs) are promising nanomaterials with a wide range of applications in drug delivery, tissue engineering, and magnetic resonance imaging (MRI) due to their superparamagnetic effects. The use of inorganic NPs has been an appealing strategy to carry and deliver drugs to the special tissues. A metal and/or a metal oxide core covered with an organic layer is the characterization of these particles which provides unique electrical, optical and magnetic properties. The current review summarizes potential targets and the application of emerging MNPs for theranostic purposes against hepatic fibrosis. Approaches in the implementation of MNPs also discussed.
Molecular Mechanism of Liver Fibrosis and Recent Clinical Trials
Fibrogenesis, the process of fibrosis development, can be assessed from the cellular point of view. Zajicek and coworkers (Zajicek et al., 1985) first reported that hepatocyte regeneration occurs in a streaming fashion. The gradual streaming of hepatocytes located at the portal space towards the hepatic vein occurs and then the apoptotic machinery removes these cells, a process lasting about 200 days in rats (Zajicek et al., 1985). During the progression of liver fibrosis, hepatic stellate cell (HSC)-derived ECM fills the empty spaces resulting from the traveling of hepatocytes. In healthy subjects, fresh hepatocytes replenish these spaces. Hepatocytes secrete molecules which act as danger signals for other cell types during the apoptosis process (Jiang et al., 2021). These signals activate immune cells which further release other factors that trigger the apoptosis of liver cells (Figure 1). All these processes surge the fibrogenic response. The initiation of the inflammatory response in NAFLD is evoked by innate immune cells which express pattern recognition receptors (PRR) to sense inflammatory mediators and danger-/pathogen-associated molecular pattern molecules (DAMP, PAMP, respectively) (Sosa et al., 2020). Recent investigations clarified the role of cholangiocytes in liver diseases especially in cholestatic liver injury (Zhou, 2020). The proliferation of cholangiocytes has been considered as an important inducer of liver fibrosis in biliary atresia (Chusilp et al., 2020). A long non-coding RNA has been identified to provoke this proliferation and thus may represent a potential target to treat fibrosis (Xiao et al., 2019). The production of bile acids decreases as a result of cholangiocyte injuries through toxic liver damages. Extracellular vesicles (EVs) and microRNAs are pivotal factors in regulating the metabolism of cyclic adenosine monophosphate (cAMP) in cholangiocytes (Sato et al., 2018). In addition to cholangiocytes, mast cells were implicated in the development of liver fibrosis. Mast cell-deficient animals have lower degrees of liver fibrosis. The effect of mast cells may be mediated through their stimulatory roles in the proliferation of cholangiocytes and in the further activation of HSCs (Meurer et al., 2020). Specific inhibition of mast cells may therefore be another target of intervention to prevent fibrosis. Yet, the reported beneficial effects of mast cells brought discrepancies in this context (Bradding and Pejler, 2018). A complex combination of cells of activation phases may be formed by hepatic macrophages that are classified as residential macrophages (Kupffer cells) and monocyte-derived macrophages (MoMF). Cell sorting implementations could separate these cells (Qiu et al., 2019). For instance, single cell RNA sequencing successfully identified several subpopulations of HSCs (Krenkel et al., 2019). The aforementioned quiescent HSCs generate a homogenous community categorized by high platelet-derived growth factor receptor beta (PDGFR-β) expression. Activated HSCs or myofibroblasts (MFBs) are consequently subdivided into populations expressing collagens, alpha-smooth muscle actin (α-SMA), and/or immune-related markers. MFBs express S100 calcium binding protein A6 (S100A6) as a general marker (Krenkel et al., 2019). The transdifferentiation of quiescent and vitamin A sorting HSCs in MFBs, leading to cell proliferation and collagen expression, is a fundamental step in the process of fibrogenesis (Trautwein et al., 2015). Extracellular signals derived from different cell types such as macrophages or hepatocytes can result in the activation of HSCs. Subsequently, the activation of HSCs was attributed to different cellular processes such as autophagy, oxidative stress, endoplasmic reticulum stress, and disturbances in metabolic pathways (Fan et al., 2020). The bidirectional cascade of fibrosis was evidenced in several studies regarding the regression of fibrosis (Parola and Pinzani, 2019). Since HSCs are responsible for excessive matrix generation, they could also be considered as valuable targets against fibrosis. Blocking the production of collagen types by specific inhibitors was suggested as novel therapeutical options (Gonçalves da Silva et al., 2021). It has been reported that, if the inflammatory is prevented, the anti-inflammatory and restorative functions of macrophages persevere and influence the hepatic niche (Novo et al., 2020). MFBs could become senescent, turn into quiescent-resembled HSCs or be eliminated via cell death processes during the regression of fibrosis (Okina et al., 2020). Certain types of macrophages produce matrix metalloproteinases (MMPs) in order to degrade the excessive ECM.
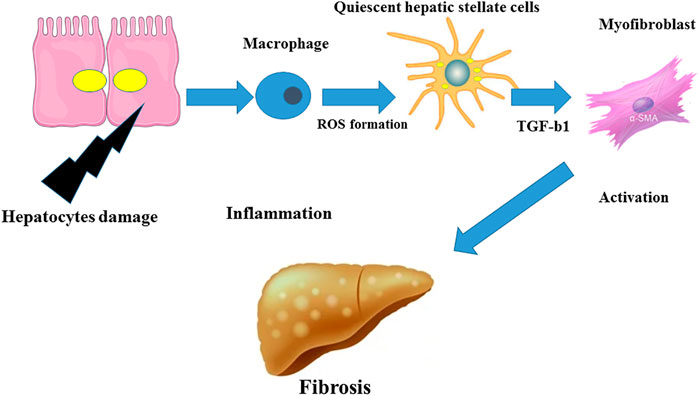
FIGURE 1. Inflammation in fibrosis. The inflammatory response could be induced by hepatocyte damage leading to the activation of macrophages and to a production of ROS and TGF-β1 and to the differentiation of quiescent HSCs in MFBs which in turn may trigger liver fibrosis.
Currently, there are few therapeutic opportunities for liver fibrosis and the introduction of efficient options needs to be addressed. Suppression of viruses, dietary alterations, decreasing body weight in NAFLD, and induction of fibrosis regression are proposed to stop the disease progression (Kohla et al., 2020; Sheka et al., 2020; Tandon and Berzigotti, 2020). The current clinical trials mostly did not focus on the direct modification of the mechanisms of the disease progressions but investigated other mechanistic approaches such as the causative agents in the underlying liver damage, the received signals from other organs, metabolic pathways, immune system, and cell death processes. Hepatocyte death is considered a key mechanism of liver fibrosis (An et al., 2020). Therefore, prohibition of cell death was proposed as a potential treatment option (Schuster-Gaul et al., 2020). Cell death is classified as necrosis or apoptosis. Necrosis itself was categorized in necroptosis, autophagy, or ferroptosis (Aizawa et al., 2020). However, a general blockage of cell death may be challenging since apoptosis is involved in the regression of fibrosis (Aizawa et al., 2020; Chang and Li, 2020). Moreover, induction of cell death is needed to enhance the death of tumor cells. Cancerous cell overcome apoptosis during oncogenesis processes, helping them to escape the immune system.
Nonetheless, several drugs were introduced to manage liver fibrosis, with the goal to specifically target hepatocyte cells. Oxidative stress stimulates the activity of the apoptosis-signal-regulating kinase (ASK1) which in turn increases hepatotoxicity, inflammation, and fibrosis (Loomba et al., 2018). Selonsertib (GS-4997, Gilead) an ASK1 inhibitor was investigated in NASH patients but the results were not fully satisfactory. Obeticholic acid (OCA) and elafibranor (ELA) are other proposed drugs which target the metabolism in the liver. OCA, a semi-synthetic bile acid was formerly approved in treating primary biliary cholangitis (PBC) and induces the activation of the nuclear bile acid receptor farnesoid X receptor (FXR) which in turn results in attenuation of bile acids. The drug has a significant effect on FXR (>100-fold) compared with endogenous bile acid, decreasing the generation of bile acid and the uptake of lipids and glucose from food (Fickert et al., 2009). ELA as an insulin sensitizer induces the activation of peroxisome-proliferator-activated receptors (PPAR) and increases the functional of endogenous insulin. It also prohibits steatosis via prevention of fat deposition in liver cells and eliminates glucose from the peripheral blood. Current research mostly aims to use a combination of OCA/ELA in the treatment of liver fibrosis (Roth et al., 2019). Cenicriviroc (CVC) is another drug suggested for the treatment of liver fibrosis but its mechanism of action consists in the inhibition of chemokines including CCR2 and CCR5 and thus in preventing lymphocyte and monocyte recruitment. The anti-fibrotic function of CVC was observed in vivo (Lefebvre et al., 2016). The effect of an oral drug in this context was investigated in a clinical study referred to as CENATUR (Friedman et al., 2016). The AURORA study is another phase III clinical trial in the treatment of NASH. There are also promising results from phase I and II clinical trials investigating the effects of emricasan, VX-166 from Vertex, and nivocasa. FXR metabolism is also being targeted using drugs such as tropifexor, cilofexor, AKN-083, and INT-767 in different clinical trials. Moreover, lanifibranor and saroglitazar target the PPAR pathway parallel to ELA, but acting on different ligands. Lanifibranor acts on PPARα/γ/δ whereas saroglitazar targets PPARα/γ. Another drug, BMS-986036, a synthetic polyethylene glycolated (PEGylated) fibroblast growth factor 21 (FGF-21), mimics the activity of liver-derived hormone FGF-21 which controls the activation of fatty acids (Fisher et al., 2014). The beneficial effects of insulin modulators such as liraglutide and semaglutide include a decreased insulin resistance and improvement of NASH (Armstrong et al., 2016). Acetyl-coenzyme A carboxylase inhibitors such as GS-0976 or PF-05221304 block the synthesis of lipids in the liver (Harrison et al., 2018). Finally, imitating gut-related signals could be another treatment strategy. NGM282 NGMBio as an analog of gut-derived hormone FGF-19 regulates the synthesis of bile acid, diminishes fat deposition in liver cells, and improves fibrosis (Harrison et al., 2018).
Importance of HSCs in Liver Fibrosis
Targeted cell-specific therapy was first introduced in the treatment of cancer but met a number of hurdles (Ledford, 2016), and targeting liver fibrosis might also face similar challenges. However, HSCs are definitely key target cells in this context (Josan et al., 2015). Yet, since they are located in the perisinusoidal space, it is difficult to deliver drugs to HSCs. HSCs are activated in response to inflammatory mediators upon liver damage and subsequently differentiate in MFBs (Li et al., 2015). Collagen and other ECM proteins such as MMPs are secreted by activated HSCs, leading to a remodeling of the liver structure (Nielsen et al., 2019). Therefore, inactivation of HSCs is one of the strategic approaches in the introduction of advanced fibrosis restoration. Different receptors such as PPAR (Palomer et al., 2018), platelet-derived growth factor receptor (PDGFR) (Josan et al., 2015), mannose-6-phosphate/insulin-like growth factor II receptor (M6P/IGF-II) (Ungefroren et al., 2018), and integrins (Peng et al., 2016) are expressed on HSCs and may thus be potential targets for pharmacological agents. Targeting M6P/IGFII with human serum albumin consisting of nanoparticles with doxorubicin was reported by Greupink et al. (Greupink et al., 2006). However, different side effects of targeting M6P/IGFII shifted the focus toward PDGRR which is significantly upregulated in fibrosis (Greupink et al., 2006). Systemic administration of interferon gamma (IFN-γ) also has some unwanted effects, yet a fusion protein of IFN-γ and PDGFβR bicyclic peptide was shown to inhibit liver fibrosis in vivo (Bansal et al., 2014), although a low bioavailability was an important drawback in this intervention. Van Dijke et al. (van Dijk et al., 2018) fabricated biodegradable microspheres with the capability of sustain the release of proteins. Integrin proteins that stimulate the activation of the transforming growth factor beta (TGF-β) and thus promote liver fibrosis are considered as potential targeting moieties (Masuzaki et al., 2021). Inhibition of αv integrins by a small molecule (CWHM-12) efficiently decreased lung and liver fibrosis (Santos and Lagares, 2018). In this regard integrin alpha 11 (IαV) is the key isotype of integrins involved in liver fibrosis as it controls the MFB phenotype and fibrogenic pathway, in particular the hedgehog signaling cascade (Bansal et al., 2017). However, integrins are implicated in many other pivotal functions of the body.
The anti-fibrotic function of the relaxin hormone was reported through binding to its receptor peptide 1 family of relaxins (RXFP1) on HSCs (Nikitin et al., 2018). Relaxin can modulate ECM remodeling and thus interfere with the secretion and degradation of its constituents (Palomer et al., 2018). Effects of relaxin on liver fibrosis were documented (Bennett et al., 2017) by preventing fibrosis through induction of an acute alteration in the liver microcirculation confirmed by morphological changes detected in non-parenchymal sinusoidal cells (Feijóo-Bandín et al., 2017). Relaxin can also reduce the content of collagen in the liver (Gracia-Sancho et al., 2015). Yet, relaxin has a short half-life and induces vasodilation upon frequent treatment in chronic fibrosis situation (Ng et al., 2018).
Magnetic Nanoparticles
Nanomedicine allowed to produce pharmacological agents at nanoscaled dimensions using nanotechnology (Wu et al., 2020). Nanomedicines can be categorized into two major groups based on the nature of the used (organic or inorganic) material. The small size of nanomaterials enables them to easily disperse in aqueous solutions which is important to administer nanodrugs in the peripheral blood (Wu et al., 2020). Nanomaterials possess a high surface-to-volume ratio that provides a large surface with the capability to be functionalized with several ligands which in turn may adapt the pharmacokinetic properties of the material. For instance, the circulation period of nanomaterials may be modified with PEG (Banshoya et al., 2020). Also, specific ligands for cellular receptors provide specific attachment to particular cell types. Conjugation of drugs to the surface of nanoparticles which control on-demand release of the drug is another prominent specification of nanomedicine (Thomas et al., 2010). Additionally, labeled materials may be applied in theranostics for several diseases (Mukherjee et al., 2020). MNPs are specific types of nanomaterials that are highly sensitive to the magnetic field. Interaction of MNPs with the magnetic field which easily penetrates through the human body enables them as valuable biomedical apparatuses. Magnetic Fe3O4 and γ-Fe2O3 are the most commonly used MNPs in biomedicine. The cubic inverted spinel construction of bulk magnetite leads to a ferromagnetic behavior of MNPs at room temperature. The nanomagnetite can be rapidly oxidized in maghemite which exhibits similar ferromagnetic specifications and spinel shape with valence electrons. Superparamagnetic iron oxide nanoparticles (SPIONs) are below 30 nm iron-oxide-based MNPs that act in the full name (SPM) regime at room temperature (Angelakeris, 2017). Ultra-small SPIONs (USPIONs) are particles with a size below 10 nm, showing diminished magnetization and elevated anisotropy due to the impact of non-collinear spins at the surface (Angelakeris, 2017). The use of SPIONs and USPIONs in biomedical applications has been in the center of great attention in particular for MRI (Figure 2) (Iv et al., 2015; Sharifi et al., 2015; Hameed and Bhattarai, 2020). The adjustable anisotropy of MNPs is required in distinct applications such as magnetic hyperthermia (Morales et al., 2018). Implementation of variations in the chemical composition of ferrits by doping the spinel shape of ferrite with transitional metals may be performed in order to tune the magnetic anisotropy (Silva et al., 2019).
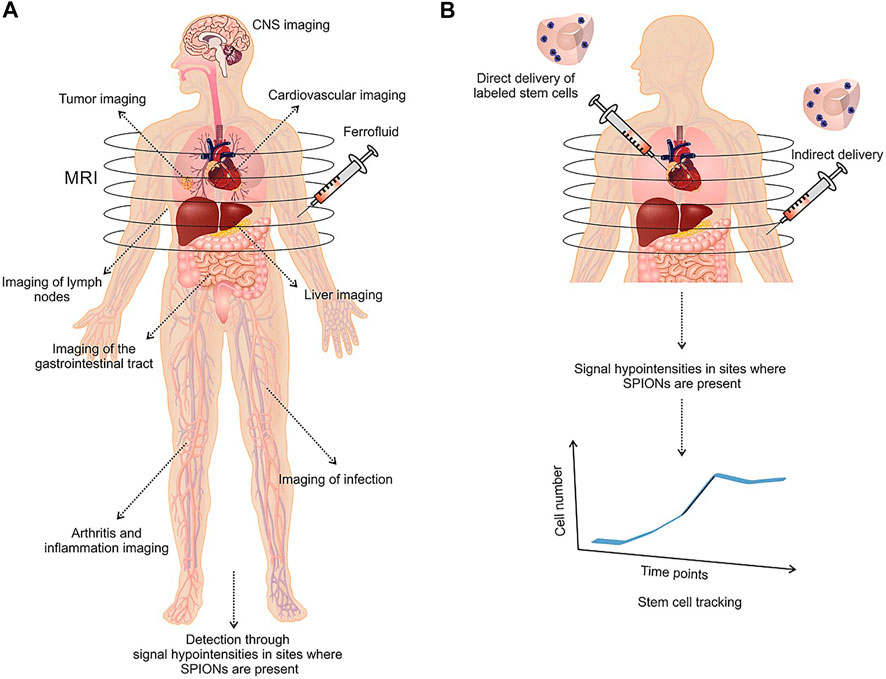
FIGURE 2. Medical applications of SPION-based MRI in liver, cardiovascular, inflammation, gastrointestinal tract, CNS, and tumor imaging (A). Application of SPION‐labeled stem cells as a monitoring regenerative therapy in tracking of stem cells after transplantation (B). Reproduced with permission (Sharifi et al., 2015) Copyright 2015, Wiley.
Synthesis of Magnetic Nanoparticles
Top-down and bottom-up approaches are important strategies for the generation of MNPs. The top-down method starts using bulk material then processed via different techniques including laser ablation and lithography (Xiao et al., 2017; Jiang et al., 2020). The bottom-up approach starts using metal ions in solution through chemical strategies as the most commonly applied method. Although lithography can successfully control the shape of nanoscaled structures, its application in large-scale production remains challenging (Lan, 2018). The synthesis of complex structures like core-shell MNPs with laser ablation is possible with a broad degree of freedom to control the variations of the material, target, environment, laser regime, and external stimulatory signals (Xiao et al., 2017). Other advantages include the lack of requirement for high pressure, temperature, or organometallic precursors to generate MNPs with high magnetic specification (Svetlichnyi et al., 2017). Therefore, the laser ablation technique is promising for the setting of higher standards in the synthesis of nanomaterials. However, the combination of the aforementioned methods is among the most commonly applied strategies. For instance, the permanent magnet construction is performed with the well-known ball milling technique of MNP synthesis (Yue et al., 2017). It can also be used in the fabrication of MNPs at industrial scale, although it remains arduous to control the size and shape of the particles. Nanoparticle agglomeration is an unwanted effect which might be problematic in biomedical application of nanomaterials (Yue et al., 2017). However, the ball milling apparatus in combination with chemistry could be applied in the mentioned mechanochemical process. Bellusci et al. (Bellusci et al., 2018) prepared MNP nanocomposites in a benzene-1,3,5-tricarboxylic acid matrix containing doxorubicin via mechanochemical processing. In this nanocomposite, the high surface area of the porous material enhanced its loading rate and magnetic specifications, permitting the introduction of excellent diagnostic systems. Different steps may be included in the synthesis of typical chemical methods, in particular burst nucleation and a subsequent propagation of nanocrystals, i.e. Ostwald ripening (Sadighian et al., 2020). The size of the particles may be controlled by alterations in temperature, solvents, and other situations as well as the manipulation of the Ostwald process (Sadighian et al., 2020). The resulting nanoparticles must have exact and predefined size, structure, and phase composition (Roca et al., 2019; Salvador et al., 2019). A four-word analysis including strengths, weaknesses, opportunities, and threats (SWOT) was proposed to monitor the common methods to produce nanoparticles (Salvador et al., 2019). The efficiency of co-precipitation, thermal decomposition (high temperature decomposition - HTD), microemulsion, microfluidic synthesis, and dual-particles formation containing different materials was evaluated. Large amounts of MNPs through alkalization of metal salt solution can be obtained using the co-precipitation method. Also, ferrite MNPs or well-crystalized iron oxide were successfully produced with this technique. However, the poor control of size distribution and shape, as well as the reduced quality of the crystals in smaller particles are critical drawbacks of the co-precipitation method (Daffé et al., 2018). High pressure and temperature through hydrothermal routs and solvothermal methods were employed to yield advanced co-precipitation strategies (Huang et al., 2018). Another interesting approach is the cost-effective polyol process that can easily be adjusted to produce large amounts of MNPs with different morphologies (Hemery et al., 2017; Franceschin et al., 2018). On the other hand, the HTD technique may be used to fabricate highly crystalized and narrow size distributed MNPs (Tomitaka et al., 2019) with high degree of magnetization that may be valuable in diagnostic methods (Chen et al., 2018). The limitations of this method according to the SWOT analysis are nevertheless time-consuming processes and expensive protocols (Salvador et al., 2019). Changing the experimental conditions may allow to control the shape of the particles (Salvador et al., 2019). It was shown that cubic MNPs exhibit higher magnetization compared with spherical particles, possibly due to lower levels of disturbed spins on the surface (Noh et al., 2012). Also, cubic MNPs have a higher binding ability resulting from their higher contact area of planar interface (Danai et al., 2021). Additionally, stronger signals are produced via cubic MNPs. Different techniques of co-precipitation as well as HTD are considered to be the most commonly used strategies to produce MNPs (Katz, 2019; Salvador et al., 2019). In addition to being cost-effective and easy, the resultant hydrophilic surfaces allow to functionalize MNPs by co-precipitation. In the second approach, HTD is an efficient technique to produce well-defined shape and narrow size distributed MNPs. However, high cost reagents and lower levels of reaction products with hydrophobic surfaces that provide the possibility of functionalization in further steps both make this technique the currently more attractive approach in the field. Table 1 summarizes the advantages and disadvantages of different synthesis methods of MNPs.
Application of Magnetic Nanoparticles in the Clinic and Perspectives
The most efficient iron-oxide-based drug is the FDA-approved feraheme® (ferumoxytol) in iron-deficient anemic patients and for cases of chronic kidney diseases. Iron is a natural agent that is eliminated from the body via normal metabolic pathways (Toth et al., 2017). The structure of feraheme® consists in non-stoichiometric magnetite MNPs coated with carboxydextran (Iv et al., 2015). Feraheme® is also applied in MRI and magnetic resonance angiography (MRA) as an off-label agent (Hood et al., 2019). The uptake of MNPs via macrophages was studied in high-resolution 3D-maps of pancreatic inflammation in vivo (Gaglia et al., 2015). The results indicated that the inflamed lesions of pancreas had higher MNP uptake. The distinct specifications of the inflammatory response on noninvasive imaging should be therefore carefully taken into account. A recent investigation showed the capability of iron oxide to generate reactive oxygen species (ROS) and its implication in the treatment of leukemia (Trujillo-Alonso et al., 2019).
Oral administration of iron oxide in more common compared with intravenous delivery. The low pH level of the gastrointestinal (GI) tract that can degrade iron oxide is still an important barrier against its oral administration (Huang et al., 2015). Coatings may therefore be needed to overcome this problem. Coatings such as silica oxide and gold which are stable in the GI tract could be used for this purpose. Mesenchymal stromal cells (MSCs) provide promising opportunities for the treatment of various disorders including liver fibrosis and HCC (Hu et al., 2019; Kang et al., 2020). Also, MNP-labeled MSCs may be used in diagnostic approaches. Faidah et al. (Faidah et al., 2017) showed that the viability (growth) of MSCs was not associated with the use of MNPs in a rat model of cirrhosis. Overexpression of the hepatocyte growth factor (HGF) in MSCs improved the recovery of hepatocytes in a fibrosis model (Lai et al., 2016). Subsequently, labeling of MSCs with MNPs resulted in an increment of cells in MRI-related imaging (Lai et al., 2016), confirming the role of MNPs in the diagnosis of liver fibrosis via MRI. The off-label application of feraheme® is currently growing. The evaluation of the stages of liver fibrosis is a key issue in the diagnosis of patients. Liver biopsy is currently the gold standard method, while imaging strategies such as relaxometry and elastography have not received much attention yet (Guimaraes et al., 2016). It was shown that different MRI-assessable differences are present in patients at different stages of liver fibrosis. However, a dual echo turbo-spin echo method can be used to quantify T2 parameters. Moreover, different stages of liver fibrosis may be distinguished via these techniques (Guimaraes et al., 2016). Iron oxide core nanoparticles can represent the superparamagnetic specifications of T2 or negative contrast agents (Wu et al., 2015). Thus, several types of synthesis, shapes, and nanoparticle coatings with magnetic cores are currently being tested. Nevertheless, there are other obstacles from other perspectives. In a study conducted by Li et al. (Li et al., 2018), immunofluorescence markers including indocyanine green were conjugated with Fe3O4. Then, early staged live fibrosis was detected by targeting the ligand for integrin avb3. Importantly, the accumulation of iron in tissues may become harmful. For instance, it was reported that administration of a single high dose of MNPs induced septic shock response and increased serum levels of liver function enzymes. Also, high doses of MNPs upregulated the expression of genes related to liver cirrhosis compared with normal doses of MNPS (Wei et al., 2016; Zhang et al., 2016). The effects of MNPs on macrophages were also investigated and it was shown that iron overload induces cellular apoptosis via activation of the c-Jun N-terminal kinase (JNK) pathway (Lunov et al., 2010). Iron overload can therefore exerts detrimental effects on the liver that contains large numbers of macrophages.
Hybrid Magnetic Nanomaterials
Although iron oxide displays a moderate toxicity, its biodistribution, biotransformation, and circulation period in blood needs to be monitored (Van de Walle et al., 2020). The hybridization of iron oxide MNPs with different agents such as polymers, noble metals, silica dioxide, and non-magnetic oxides could pave the way to lessen their cytotoxicity (Lavorato et al., 2018; Efremova et al., 2018; Nguyen et al., 2018). PEG was most frequently used as an organic coating for MNPs. Higher molecular weight PEG can significantly increase the blood half-life of MNPs (El-Boubbou, 2018). The pharmacokinetic behavior of MNPs could be substantially affected after coating since their surface charge might be changed (Alphandéry, 2019). A longer half-life is observed in negatively charged MNPs (Alphandéry, 2019). Modification of the surface of MNPs with genes, drugs, and biomolecules may be also via coating. Moreover, hybrid materials exhibit other functionalities, for instance, gold nanoparticles show the surface plasmon resonance phenomenon altering the optical specifications of the material that can be used in photothermal therapy detection (Dheyab et al., 2020). Nanohybrids of gold and magnetite were fabricated in Janus-like MNPs (Efremova et al., 2018). Nanohybrids do not only provide two district surfaces for conjugation of two different molecules but also result in elevated contrast in MRI and real-time delivery of drugs through intravital fluorescence microscopy (Prasad et al., 2018). Citrate-coated ultrasmall SPIONs with highly water dispersibility and smaller size (12 nm) showed acceptable MRI contrast in liver fibrosis imaging and may be applied as platforms for multifunctional nanoprobes in the future. This particle showed good efficacy and biocompatibility in cell labeling and also represented an enhanced MRI contrast in a rat model of liver fibrosis. As shown in Figure 3, the quality of contrast significantly increased upon injection of aforementioned SPIONs (Saraswathy et al., 2014) (Figure 3). Lipid-based nanoparticles have been classified as more prevalent nanomedicines in the market (Ickenstein and Garidel, 2019). Thus, a combination of SPIONs and liposomes offered great advantages in this regard. The use of magnetoliposomes in the induction of nucleic acid release using a magnetic field may also be useful in drug delivery (Dwivedi et al., 2020). The release of DNA from hybrid nanomaterials could be increased via SPIONs (Magro et al., 2017). The increasing number of synthesized hybrid nanomaterials enhances the hope in application of novel means of drug delivery in the future.
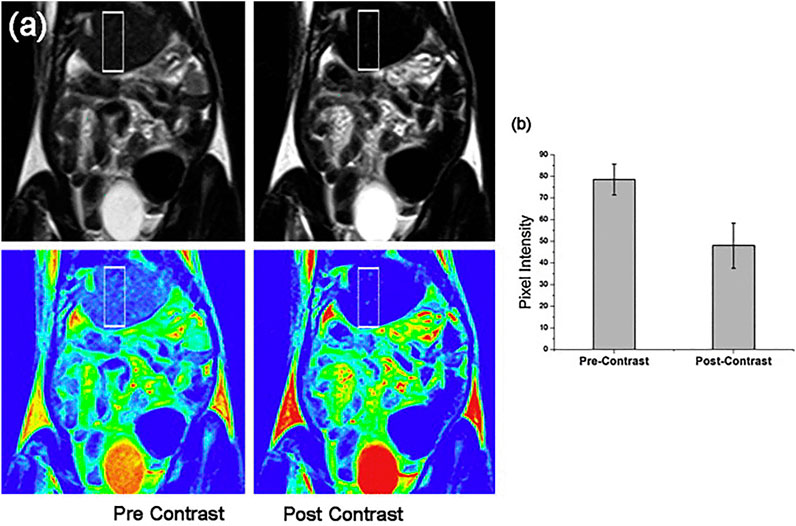
FIGURE 3. Comparison of pre- and post-contrast in vivo MRI of the liver in a region of interest from fibrosed areas (above) and in colorized images of the original MRI (below) after 10 min of application of citrate-coated ultrasmall SPIONs (A). The graph of pixel intensity in pre- and post-contrast of liver is presented in (B). Reproduced with permission (Saraswathy et al., 2014) Copyright 2014, Elsevier B.V.
Magnetic Nanoparticles for Liver Fibrosis Theranostics
The behavior and distribution of nanomaterials are significantly in association with their size. Small particles in the circulation incline towards aggregation or to be decorated with a protein corona and, due to their charge, to produce an electrical double layer (Ramachandran et al., 2020). In this context, dynamic light scattering (DLS) can easily measure the total size of the particles (Ramachandran et al., 2020). Intravenous administration of MNPs leads to the circulation of the particles in the lumen of blood vessels and thus to their interaction with macrophages of the reticuloendothelial system (Alphandéry, 2019). USPIONs exhibit longer in-blood circulation time compared with SPIONs (El-Boubbou, 2018). Also, previous in vitro/in vivo studies revealed that FGF-2-conjugated SPIONs effectively amended HSC activation as a promising therapeutic method for the treatment of liver fibrosis. This was proved with different molecular experiments. The immunofluorescent images, gene expression and western blot analysis showed FGF2-SPIONs plummeted both TGFβ-induced a-SMA and collagen I expression. also, pAkt was downregulated in TGF-b activated LX2 cells (Figure 4) (Kurniawan et al., 2020).
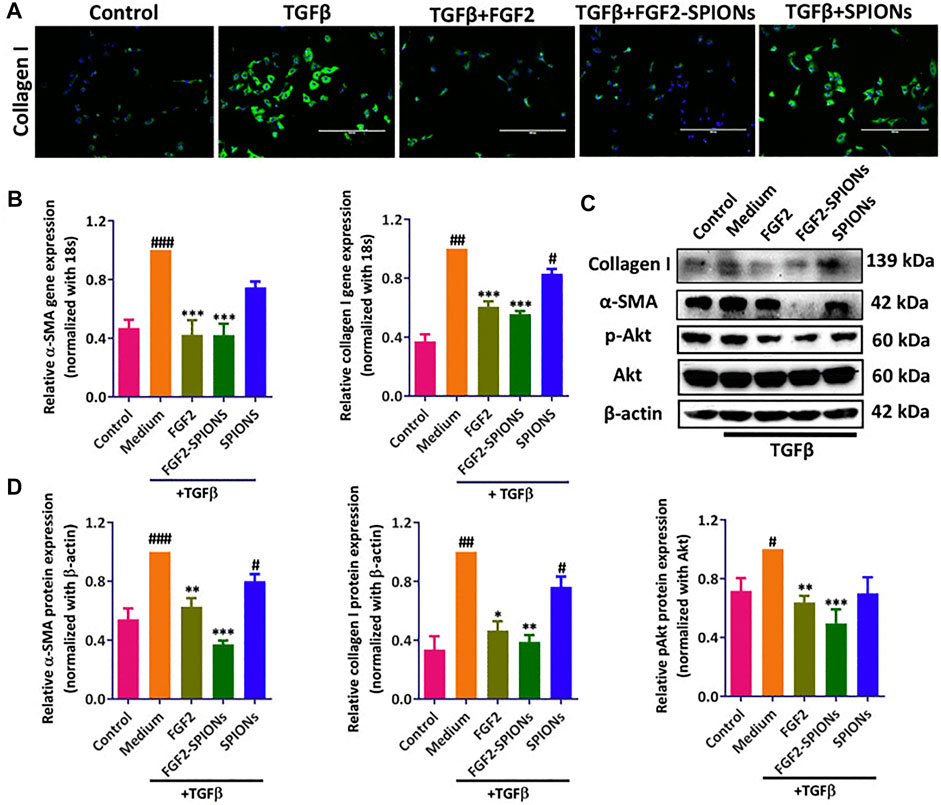
FIGURE 4. Effects of FGF-2-conjugated SPIONs on HSC activation in vitro and in vivo. (A) Immunofluorescent detection of type-I collagen in control and TGF-β-stimulated LX2 cells in control, FGF-2-, FGF-2-SPION-, or SPION-treated groups. (B) Expression levels of a-SMA and type-I collagen in control, FGF-2-, FGF-2-SPION-, or SPION-treated groups. (C) Western-blot analysis of type-I collagen, pAkt, Akt, a-SMA, and ß-actin in control, FGF-2-, FGF-2-SPION-, or SPION-treated groups. (D) Quantitative analysis of Western-blot results. Reproduced under the terms and conditions of the Creative Commons Attribution 4.0 International License (Kurniawan et al., 2020). Copyright 2020, Elsevier.
As mentioned above, the size of MNPs may affect their pharmacokinetic properties and biodistribution. MNPs lower than 15 nm are easily filtered by the renal system while MNPs larger than 100 nm accumulate in hepatocytes and those of 100–150 nm are trapped by Kupffer cells and splenic macrophages (Guleria et al., 2018). Spleen and liver as the initial sites of the accumulation of MNPs could be targeted with such particles (Quini et al., 2017). The median size of 10–50 nm is supposed to exert optimal properties, including longer circulation time (Setua et al., 2018). However, other properties such as hydrophobic/hydrophilic features and zeta potential of the particles interfere with their biodistribution (Socoliuc et al., 2020). The MRI enhancement of liver disorders was achieved by a non-specific biodistribution of MNPs (Dadfar et al., 2019). The majority (80%) of non-specific SPIONs which were delivered via an intravenous route were internalized by Kupffer cells (Dadfar et al., 2019). Thus, the produced MR-contrast via MNPs allows to trace hepatic tumor cells (Dadfar et al., 2019).
There are four important non-exhaustive areas where MNPs paly crucial roles. Application of the magnetic field for binding-related cell capturing is the first important concept. Cancer treatment was significantly improved using chimeric antigen receptor (CAR) T cells based on genetic engineering of isolated T cells to form autologous donors that further target specific antigens (Levine, 2015). This technology is currently approved in treating B cell lymphoma, but different clinical studies evaluated its effectiveness against liver cancer (Li et al., 2020). It was shown that macrophages play a chief role in the modulation of adverse effects of CAR T cell-based therapy (Giavridis et al., 2018). The manipulation of other immune cells such as natural killer cells was also addressed (Rezvani et al., 2017). A key technology by cell sorting in closed systems is microfluidics (Liu et al., 2016) using MNPs to provide lab-on-chip devices. Magnetic fields provided powerful systems in tissue engineering processes, in particular the rearrangement of different cell layers (Omelyanchik et al., 2018). Also, establishment of 3D cell culture arrays which enable cellular patterning for an assessment of the impact of fibroblasts and their infiltration properties are provided by magnetic fields (Shim and Hur, 2017). MNPs can be localized in particular parts of the body using magnetic fields which permit to selectively transmit distinct payload to immune cells depending on the phagocytic uptake to injured areas of the liver (Rieck et al., 2019). The spatial concentration of MNPs may also be regulated via MRI apparatuses (Pi et al., 2018).
Mechanical cell control is a second area of application of MNPs. The evaluation of the stiffness of biological molecules and intracellular organelles might be possible in a near future. In this context, magnetic tweezers may be used as potential tools to measure the mechanical forces of biomolecules (Wang et al., 2019). Directed cell apoptosis may be triggered using low-frequency magnetic fields (Zamay et al., 2017). Modulation of stem cell differentiation of the behaviors of single cells was previously regulated in the concept of mechanical cell control (Du et al., 2017). Magnetic tweezer-based manipulations as prominent biophysical methods are useful in single-molecule unfolding, determination of forced-regulated processes in viable cell and rheology measurements (Lipfert et al., 2010).
Drug delivery is a third application of MNPs. In addition to the native toxic effects of MNPs and their thermomagnetic effects, targeted drug delivery with the aid of these particles is a potential means to eliminate injured or infected cells (He et al., 2018). Magnetism-based application in the field of drug delivery has several benefits such as an increased release of drugs from a single carrier from porous materials, an azo-functionalized MNP-based remote control of drug release, and the enhancement of drug release from magnetically regulated nanocarriers (Fuller et al., 2019).
The fourth application of magnetism is its advantage in imaging techniques. Non-invasive assessment of elastin or collagen in liver fibrosis may be visualized by MRI-based methods (Salarian et al., 2020). Early treatment of liver fibrosis is connected with the early diagnosis of the disease although no established marker of fibrosis on early stages is present. Performing liquid biopsy via detection of N-terminal propeptide of type-III collagen (Pro-C3) might be promising in early diagnosis of liver fibrosis (Piazzolla and Mangia, 2020). Pro-C3 screening was used to detect hepatitis C and to predict the progression of liver fibrosis (Nielsen et al., 2015). Peripheral microRNAs, long non-coding RNA, and microbiomes may be considered as other types of biomarkers (Zhang et al., 2019; Li et al., 2020). The findings of biomarker screening are often confirmed by non-invasive imaging methods. Currently, there is an emerging need for the development of novel techniques in non-invasive diagnosis of liver fibrosis. A targeted USPION-based contrast agent was applied for the MRI-associated detection of fibrosis stages in vivo (Saraswathy et al., 2014). The degree of the interactions of protons whose relaxation period changes in the presence of a magnetic field with MNPs determine the MRI contrast level (Gossuin et al., 2016). Moreover, magnetic particle imaging (MPI) is capable of directly imaging the distribution of MNPs. The technique was first represented in 2005 (Nikitin et al., 2018; Sehl et al., 2020). Since MPI exhibits enhanced temporal and spatial resolution compared with MRI, it may represent a promising system for the detection of liver diseases (Sehl et al., 2020). The use of MNPs as biosensors might be another interesting methodology (Gessner et al., 2021). Elastin is increased in later stages of liver fibrosis and imaging probes of elastin can detect late stages of the diseases (Nagórniewicz et al., 2019). USPIONs can bind to the tripeptide arginine-glycine-aspartic acid (RGD) expressed on HSCs and thus, predict liver fibrosis stages via MRI (Zhang et al., 2016). Nanohybrid-based multimodal imaging is another groundbreaking progress to detect liver diseases (Li et al., 2018). The targeting of RGD and fluorescence imaging was increased using USPION-SiO2 conjugates with indocyanine green dye. In theranostic applications, a drug should also be used (Bartneck et al., 2012). Relaxin-conjugated MNPs substantially decreased the differentiation, migration, and contractile capability of HSCs (Nagórniewicz et al., 2019). Due to the unique properties of MNPs, their careful application with targeting distinct receptors on HSCs may still potentially pave the way for future adapted treatments against liver fibrosis (Figure 5) (Nagórniewicz et al., 2019).
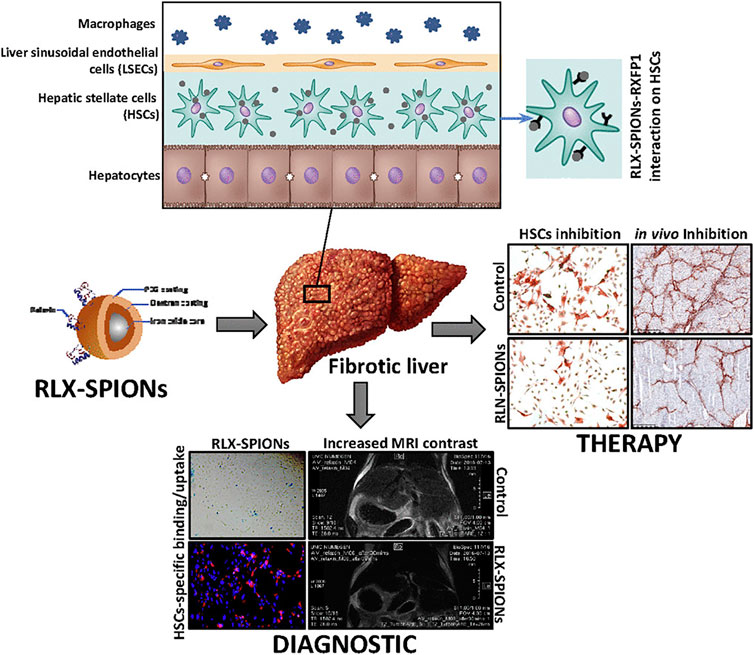
FIGURE 5. The role of engineered Relaxin in the treatment and diagnosis of liver cirrhosis. Reproduced with permission (Nagórniewicz et al., 2019). Copyright 2019, Elsevier Inc.
Outlook and Future Prospects
Unique advantages of Nano medicines as technological drivers of innovation are owed to advanced, targeted, and blood-brain-crossing theranostics platforms. The capability of MNPs to respond external magnetic fields turns them into versatile particles which can be functionalized with bioactive compounds. Diminished magnetization modalities with special surface modifications, the dissociations of functionalized domain with magnetic core, the distances between magnetic field and target site, and potential cytotoxicities are current challenges of magnetic nanotechnology. Importantly, the plausible tissue-toxicities of MNPs should be evaluated. Also, by enhanced nanotoxicology determinations, mechanistic strategies and clinical relevance should be taken into account.
Conclusion
This review focused on a description of molecular mechanisms and most important factors of deleterious liver diseases such as fibrosis and on MNPs as a promising tool for modern nanomedicine. These nanoscaled materials exhibit a great potential for theranostics of fibrosis due to their unique properties such as good biocompatibility, superparamagneticity, large surface area, high stability, and imaging contrast. Possible complications during the transfer of magnetic nanotechnologies from laboratory models to clinical usage were also discussed.
Author Contributions
All of authors (AE, AlA, AyA, HŠ, OnŠ, HA, EA, OlS, RK, TK, and MC) have read, approved and made substantial contributions for the manuscript. None of the original material contained in this manuscript has been previously published nor is currently under review for publication elsewhere.
Funding
This work was supported in part by the Maragheh University of Medical Sciences, Tabriz University of Medical Sciences, Ministry of Education and Science of Ukraine (projects Nos. 0118U000297 and 0119U100671), National Academy of Sciences of Ukraine in the framework of the Scientific-Technical Program “Smart sensor devices of a new generation based on modern materials and technologies” (project No. 13), National Research Foundation of Ukraine (project No. 48/02.2020), Slovak Grant Agency VEGA (projects Nos. 2/0157/17 and 2/0029/20), and Slovak Research and Development Agency (project No. APVV-16-0369). RK was supported by the Russian Institute for Advanced Study, Moscow State Pedagogical University in the framework of the VI International Scientific Grants Programme 2021.
Conflict of Interest
The authors declare that the research was conducted in the absence of any commercial or financial relationships that could be construed as a potential conflict of interest.
Acknowledgments
AE and TK also acknowledge the SAIA (Slovak Academic Information Agency) for scholarships in the PI-SAS and IP-SAS, respectively, in the framework of the National Scholarship Programme of the Slovak Republic. We acknowledge support by the Saarland University within the funding programme Open Access Publishing.
References
Aizawa, S., Brar, G., and Tsukamoto, H. (2020). Cell Death and Liver Disease. Gut and Liver 14 (1), 20–29. doi:10.5009/gnl18486
Alphandéry, E. (2019). Biodistribution and Targeting Properties of Iron Oxide Nanoparticles for Treatments of Cancer and Iron Anemia Disease. Nanotoxicology 13 (5), 573–596. doi:10.1080/17435390.2019.1572809
An, P., Wei, L.-L., Zhao, S., Sverdlov, D. Y., Vaid, K. A., Miyamoto, M., et al. (2020). Hepatocyte Mitochondria-Derived Danger Signals Directly Activate Hepatic Stellate Cells and Drive Progression of Liver Fibrosis. Nat. Commun. 11 (1), 2362. doi:10.1038/s41467-020-16092-0
Angelakeris, M. (2017). Magnetic Nanoparticles: A Multifunctional Vehicle for Modern Theranostics. Biochim. Biophys. Acta (Bba)—Gen. Subjects 1861 (6), 1642–1651. doi:10.1016/j.bbagen.2017.02.022
Armstrong, M. J., Gaunt, P., Aithal, G. P., Barton, D., Hull, D., Parker, R., et al. (2016). Liraglutide Safety and Efficacy in Patients with Non-alcoholic Steatohepatitis (LEAN): A Multicentre, Double-Blind, Randomised, Placebo-Controlled Phase 2 Study. Lancet 387 (10019), 679–690. doi:10.1016/S0140-6736(15)00803-X
Bansal, R., Nakagawa, S., Yazdani, S., Van Baarlen, J., Venkatesh, A., Koh, A. P., et al. (2017). Integrin Alpha 11 in the Regulation of the Myofibroblast Phenotype: Implications for Fibrotic Diseases. Exp. Mol. Med. 49 (11), e396. doi:10.1038/emm.2017.213
Bansal, R., Prakash, J., De Ruiter, M., and Poelstra, K. (2014). Targeted Recombinant Fusion Proteins of IFNγ and Mimetic IFNγ with PDGFβR Bicyclic Peptide Inhibits Liver Fibrogenesis In Vivo and Mimetic IFN with PDGFβR Bicyclic Peptide Inhibits Liver Fibrogenesis in In Vivo. PLoS One 9 (2), e89878. doi:10.1371/journal.pone.0089878
Banshoya, K., Nakamura, T., Tanaka, T., and Kaneo, Y. (2020). Coenzyme Q10-Polyethylene Glycol Monostearate Nanoparticles: An Injectable Water-Soluble Formulation. Antioxidants 9 (1), 86. doi:10.3390/antiox9010086
Bartneck, M., Ritz, T., Keul, H. A., Wambach, M., Bornemann, J., Gbureck, U., et al. (2012). Peptide-functionalized Gold Nanorods Increase Liver Injury in Hepatitis. ACS Nano 6 (10), 8767–8777. doi:10.1021/nn302502u
Bellusci, M., Guglielmi, P., Masi, A., Padella, F., Singh, G., Yaacoub, N., et al. (2018). Magnetic Metal-Organic Framework Composite by Fast and Facile Mechanochemical Process. Inorg. Chem. 57 (4), 1806–1814. doi:10.1021/acs.inorgchem.7b02697
Bennett, R. G., Simpson, R. L., and Hamel, F. G. (2017). Serelaxin Increases the Antifibrotic Action of Rosiglitazone in a Model of Hepatic Fibrosis. World J. Gastroenterol. 23 (22), 3999. doi:10.3748/wjg.v23.i22.3999
Bradding, P., and Pejler, G. (2018). The Controversial Role of Mast Cells in Fibrosis. Immunol. Rev. 282 (1), 198–231. doi:10.1111/imr.12626
Chang, Y., and Li, H. (2020). Hepatic Antifibrotic Pharmacotherapy: Are We Approaching Success?. J. Clin. Transl. Hepatol. 8 (2), 222–229. doi:10.14218/JCTH.2020.00026
Chen, Y., Ding, X., Zhang, Y., Natalia, A., Sun, X., Wang, Z., et al. (2018). Design and Synthesis of Magnetic Nanoparticles for Biomedical Diagnostics. Quant. Imaging Med. Surg. 8 (9), 957–970. doi:10.21037/qims.2018.10.07
Chusilp, S., Lee, C., Li, B., Lee, D., Yamoto, M., Ganji, N., et al. (2020). A Novel Model of Injured Liver Ductal Organoids to Investigate Cholangiocyte Apoptosis with Relevance to Biliary Atresia. Pediatr. Surg. Int. 36 (12), 1471–1479. doi:10.1007/s00383-020-04765-2
Dadfar, S. M., Roemhild, K., Drude, N. I., von Stillfried, S., Knüchel, R., Kiessling, F., et al. (2019). Iron Oxide Nanoparticles: Diagnostic, Therapeutic and Theranostic Applications. Adv. Drug Deliv. Rev. 138, 302–325. doi:10.1016/j.addr.2019.01.005
Daffé, N., Choueikani, F., Neveu, S., Arrio, M.-A., Juhin, A., Ohresser, P., et al. (2018). Magnetic Anisotropies and Cationic Distribution in CoFe2O4 Nanoparticles Prepared by Co-precipitation Route: Influence of Particle Size and Stoichiometry. J. Magnetism Magn. Mater. 460, 243–252. doi:10.1016/j.jmmm.2018.03.041
Danai, P. E., Michael, P., Gabriella, F., Francesca, M., Kalska-Szostko, B., Giorgos, P., et al. (2021). Synthesis and Characterization of Modified Magnetic Nanoparticles as Theranostic Agents: In vitro Safety Assessment in Healthy Cells. Toxicol. Vitro 72, 105094. doi:10.1016/j.tiv.2021.105094
Dheyab, M. A., Aziz, A. A., Jameel, M. S., Khaniabadi, P. M., Mehrdel, B., and Khaniabadi, B. M. (2020). Gold-coated Iron Oxide Nanoparticles as a Potential Photothermal Therapy Agent to Enhance Eradication of Breast Cancer Cells. J. Phys. Conf. Ser. 1497, 012003. doi:10.1088/1742-6596/1497/1/012003
Du, V., Luciani, N., Richard, S., Mary, G., Gay, C., Mazuel, F., et al. (2017). A 3D Magnetic Tissue Stretcher for Remote Mechanical Control of Embryonic Stem Cell Differentiation. Nat. Commun. 8 (1), 400. doi:10.1038/s41467-017-00543-2
Dwivedi, P., Kiran, S., Han, S., Dwivedi, M., Khatik, R., Fan, R., et al. (2020). Magnetic Targeting and Ultrasound Activation of Liposome-Microbubble Conjugate for Enhanced Delivery of Anticancer Therapies. ACS Appl. Mater. Inter. 12 (21), 23737–23751. doi:10.1021/acsami.0c05308
Efremova, M. V., Naumenko, V. A., Spasova, M., Garanina, A. S., Abakumov, M. A., Blokhina, A. D., et al. (2018). Magnetite-gold Nanohybrids as Ideal All-In-One Platforms for Theranostics. Sci. Rep. 8 (1), 11295. doi:10.1038/s41598-018-29618-w
El-Boubbou, K. (2018). Magnetic Iron Oxide Nanoparticles as Drug Carriers: Clinical Relevance. Nanomedicine 13 (8), 953–971. doi:10.2217/nnm-2017-0336
Faidah, M., Noorwali, A., Atta, H., Ahmed, N., Habib, H., Damiati, L., et al. (2017). Mesenchymal Stem Cell Therapy of Hepatocellular Carcinoma in Rats: Detection of Cell Homing and Tumor Mass by Magnetic Resonance Imaging Using Iron Oxide Nanoparticles. Adv. Clin. Exp. Med. 26 (8), 1171–1178. doi:10.17219/acem/67563
Fan, J., Shi, Y., and Peng, Y. (2020). Autophagy and Liver Diseases. Autophagy: Biology and Diseases. Springer.
Fan, Q.-Q., Zhang, C.-L., Qiao, J.-B., Cui, P.-F., Xing, L., Oh, Y.-K., et al. (2020). Extracellular Matrix-Penetrating Nanodrill Micelles for Liver Fibrosis Therapy. Biomaterials 230, 119616. doi:10.1016/j.biomaterials.2019.119616
Feijóo-Bandín, S., Aragón-Herrera, A., Rodríguez-Penas, D., Portolés, M., Roselló-Lletí, E., Rivera, M., et al. (2017). Relaxin-2 in Cardiometabolic Diseases: Mechanisms of Action and Future Perspectives. Front. Physiol. 8, 599. doi:10.3389/fphys.2017.00599
Fickert, P., Fuchsbichler, A., Moustafa, T., Wagner, M., Zollner, G., Halilbasic, E., et al. (2009). Farnesoid X Receptor Critically Determines the Fibrotic Response in Mice but Is Expressed to a Low Extent in Human Hepatic Stellate Cells and Periductal Myofibroblasts. Am. J. Pathol. 175 (6), 2392–2405. doi:10.2353/ajpath.2009.090114
Fisher, F. M., Chui, P. C., Nasser, I. A., Popov, Y., Cunniff, J. C., Lundasen, T., et al. (2014). Fibroblast Growth Factor 21 Limits Lipotoxicity by Promoting Hepatic Fatty Acid Activation in Mice on Methionine and Choline-Deficient Diets. Gastroenterology 147 (5), 1073–1083. doi:10.1053/j.gastro.2014.07.044
Franceschin, G., Gaudisson, T., Menguy, N., Dodrill, B. C., Yaacoub, N., Grenèche, J.-M., et al. (2018). Exchange-Biased Fe3−xO4-CoO Granular Composites of Different Morphologies Prepared by Seed-Mediated Growth in Polyol: From Core-Shell to Multicore Embedded Structures. Part. Part. Syst. Charact. 35 (8), 1800104. doi:10.1002/ppsc.201800104
Friedman, S., Sanyal, A., Goodman, Z., Lefebvre, E., Gottwald, M., Fischer, L., et al. (2016). Efficacy and Safety Study of Cenicriviroc for the Treatment of Non-alcoholic Steatohepatitis in Adult Subjects with Liver Fibrosis: CENTAUR Phase 2b Study Design. Contemp. Clin. Trials 47, 356–365. doi:10.1016/j.cct.2016.02.012
Fuller, E. G., Sun, H., Dhavalikar, R. D., Unni, M., Scheutz, G. M., Sumerlin, B. S., et al. (2019). Externally Triggered Heat and Drug Release from Magnetically Controlled Nanocarriers. ACS Appl. Polym. Mater. 1 (2), 211–220. doi:10.1021/acsapm.8b00100
Gaglia, J. L., Harisinghani, M., Aganj, I., Wojtkiewicz, G. R., Hedgire, S., Benoist, C., et al. (2015). Noninvasive Mapping of Pancreatic Inflammation in Recent-Onset Type-1 Diabetes Patients. Proc. Natl. Acad. Sci. U.S.A 112 (7), 2139–2144. doi:10.1073/pnas.1424993112
Gessner, I., Fries, J. W. U. J., Brune, V., and Mathur, S. (2021). Magnetic Nanoparticle-Based Amplification of microRNA Detection in Body Fluids for Early Disease Diagnosis. J. Mater. Chem. B. 9 (1), 9–22. doi:10.1039/D0TB02165B
Giavridis, T., van der Stegen, S. J. C., Eyquem, J., Hamieh, M., Piersigilli, A., and Sadelain, M. (2018). CAR T Cell-Induced Cytokine Release Syndrome Is Mediated by Macrophages and Abated by IL-1 Blockade. Nat. Med. 24 (6), 731–738. doi:10.1038/s41591-018-0041-7
Gonçalves da Silva, E. F., Costa, B. P., Nassr, M. T., de Souza Basso, B., Bastos, M. S., Antunes, G. L., et al. (2021). Therapeutic Effect of Uridine Phosphorylase 1 (UPP1) Inhibitor on Liver Fibrosis In Vitro and In Vivo. Eur. J. Pharmacol. 890, 173670. doi:10.1016/j.ejphar.2020.173670
Gossuin, Y., Orlando, T., Basini, M., Henrard, D., Lascialfari, A., Mattea, C., et al. (2016). NMR Relaxation Induced by Iron Oxide Particles: Testing Theoretical Models. Nanotechnology 27 (15), 155706. doi:10.1088/0957-4484/27/15/155706
Gracia-Sancho, J., Maeso-Díaz, R., Fernández-Iglesias, A., Navarro-Zornoza, M., and Bosch, J. (2015). New Cellular and Molecular Targets for the Treatment of Portal Hypertension. Hepatol. Int. 9 (2), 183–191. doi:10.1007/s12072-015-9613-5
Greupink, R., Bakker, H. I., van Goor, H., de Borst, M. H., Beljaars, L., and Poelstra, K. (2006). Mannose-6-phosphate/insulin-like Growth Factor-II Receptors May Represent a Target for the Selective Delivery of Mycophenolic Acid to Fibrogenic Cells. Pharm. Res. 23 (8), 1827–1834. doi:10.1007/s11095-006-9025-2
Guimaraes, A. R., Siqueira, L., Uppal, R., Alford, J., Fuchs, B. C., Yamada, S., et al. (2016). T2 Relaxation Time Is Related to Liver Fibrosis Severity. Quant. Imaging Med. Surg. 6 (2), 103–114. doi:10.21037/qims.2016.03.02
Guleria, A., Priyatharchini, K., and Kumar, D. (2018). Biomedical Applications of Magnetic Nanomaterials. Applications of Nanomaterials. Elsevier.
Hameed, S., and Bhattarai, P. (2020). Nanosized Magnetic Particles for Cancer Theranostics. Biopolymer-Based Formulations. Elsevier.
Harrison, S. A., Rinella, M. E., Abdelmalek, M. F., Trotter, J. F., Paredes, A. H., Arnold, H. L., et al. (2018). NGM282 for Treatment of Non-alcoholic Steatohepatitis: A Multicentre, Randomised, Double-Blind, Placebo-Controlled, Phase 2 Trial. The Lancet 391 (10126), 1174–1185. doi:10.1016/S0140-6736(18)30474-4
He, S., Zhang, H., Liu, Y., Sun, F., Yu, X., Li, X., et al. (2018). Maximizing Specific Loss Power for Magnetic Hyperthermia by Hard-Soft Mixed Ferrites. Small 14 (29), 1800135. doi:10.1002/smll.201800135
Hemery, G., Keyes, A. C., Garaio, E., Rodrigo, I., Garcia, J. A., Plazaola, F., et al. (2017). Tuning Sizes, Morphologies, and Magnetic Properties of Monocore versus Multicore Iron Oxide Nanoparticles through the Controlled Addition of Water in the Polyol Synthesis. Inorg. Chem. 56 (14), 8232–8243. doi:10.1021/acs.inorgchem.7b00956
Hood, M. N., Blankholm, A. D., and Stolpen, A. (2019). The Rise of Off-Label Iron-Based Agents in Magnetic Resonance Imaging. J. Radiol. Nurs. 38 (1), 38–41. doi:10.1016/j.jradnu.2018.11.004
Hu, C., Zhao, L., Duan, J., and Li, L. (2019). Strategies to Improve the Efficiency of Mesenchymal Stem Cell Transplantation for Reversal of Liver Fibrosis. J. Cel. Mol. Med. 23 (3), 1657–1670. doi:10.1111/jcmm.14115
Huang, J., Shu, Q., Wang, L., Wu, H., Wang, A. Y., and Mao, H. (2015). Layer-by-layer Assembled Milk Protein Coated Magnetic Nanoparticle Enabled Oral Drug Delivery with High Stability in Stomach and Enzyme-Responsive Release in Small Intestine. Biomaterials 39, 105–113. doi:10.1016/j.biomaterials.2014.10.059
Huang, Z., Qi, J., Zhou, M., Gong, Y., Shi, Q., Yang, M., et al. (2018). A Facile Solvothermal Method for High-Quality Gd2Zr2O7 Nanopowder Preparation. Ceramics Int. 44 (2), 1334–1342. doi:10.1016/j.ceramint.2017.08.154
Ickenstein, L. M., and Garidel, P. (2019). Lipid-based Nanoparticle Formulations for Small Molecules and RNA Drugs. Expert Opin. Drug Deliv. 16 (11), 1205–1226. doi:10.1080/17425247.2019.1669558
Iv, M., Telischak, N., Feng, D., Holdsworth, S. J., Yeom, K. W., and Daldrup-Link, H. E. (2015). Clinical Applications of Iron Oxide Nanoparticles for Magnetic Resonance Imaging of Brain Tumors. Nanomedicine 10 (6), 993–1018. doi:10.2217/nnm.14.203
Jiang, C., Oshima, D., Iwata, S., Pong, P. W. T., and Kato, T. (2020). Patterned Arrays of Assembled Nanoparticles Prepared by Interfacial Assembly and Femtosecond Laser Fabrication. J. Nanopart. Res. 22 (1), 1–12. doi:10.1007/s11051-019-4718-8
Jiang, Y., Xiang, C., Zhong, F., Zhang, Y., Wang, L., Zhao, Y., et al. (2021). Histone H3K27 Methyltransferase EZH2 and Demethylase JMJD3 Regulate Hepatic Stellate Cells Activation and Liver Fibrosis. Theranostics 11 (1), 361–378. doi:10.7150/thno.46360
Josan, S., Billingsley, K., Orduna, J., Park, J. M., Luong, R., Yu, L., et al. (2015). Assessing Inflammatory Liver Injury in an Acute CCl4model Using Dynamic 3D Metabolic Imaging of Hyperpolarized [1-13C]pyruvate. NMR Biomed. 28 (12), 1671–1677. doi:10.1002/nbm.3431
Kang, S. H., Kim, M. Y., Eom, Y. W., and Baik, S. K. (2020). Mesenchymal Stem Cells for the Treatment of Liver Disease: Present and Perspectives. Gut and Liver 14 (3), 306–315. doi:10.5009/gnl18412
Katz, E. (2019). Synthesis, Properties and Applications of Magnetic Nanoparticles and Nanowires-A Brief Introduction. Magnetochemistry 5 (4), 61. doi:10.3390/magnetochemistry5040061
Kohla, M. A. S., Fayoumi, A. E., Akl, M., Abdelkareem, M., Elsakhawy, M., Waheed, S., et al. (2020). Early Fibrosis Regression by Shear Wave Elastography after Successful Direct-Acting Anti-HCV Therapy. Clin. Exp. Med. 20 (1), 143–148. doi:10.1007/s10238-019-00597-0
Krenkel, O., Hundertmark, J., Ritz, T., Weiskirchen, R., and Tacke, F. (2019). Single Cell RNA Sequencing Identifies Subsets of Hepatic Stellate Cells and Myofibroblasts in Liver Fibrosis. Cells 8 (5), 503. doi:10.3390/cells8050503
Kurniawan, D. W., Booijink, R., Pater, L., Wols, I., Vrynas, A., Storm, G., et al. (2020). Fibroblast Growth Factor 2 Conjugated Superparamagnetic Iron Oxide Nanoparticles (FGF2-SPIONs) Ameliorate Hepatic Stellate Cells Activation In Vitro and Acute Liver Injury In Vivo. J. Controlled Release 328, 640–652. doi:10.1016/j.jconrel.2020.09.041
Lai, L., Chen, J., Wei, X., Huang, M., Hu, X., Yang, R., et al. (2016). Transplantation of MSCs Overexpressing HGF into a Rat Model of Liver Fibrosis. Mol. Imaging Biol. 18 (1), 43–51. doi:10.1007/s11307-015-0869-x
Lan, H. (2018). Micro/Nanolithography-A Heuristic Aspect on the Enduring Technology. London: IntechOpen, Chap. 3, 43–68. doi:10.5772/intechopen.72860 Large-Area Nanoimprint Lithography and Applications
Lavorato, G., Lima, E., Vasquez Mansilla, M., Troiani, H., Zysler, R., and Winkler, E. (2018). Bifunctional CoFe2O4/ZnO Core/Shell Nanoparticles for Magnetic Fluid Hyperthermia with Controlled Optical Response. J. Phys. Chem. C 122 (5), 3047–3057. doi:10.1021/acs.jpcc.7b11115
Ledford, H. (2016). Bankruptcy Filing Worries Developers of Nanoparticle Cancer Drugs. Nature 533 (7603), 304–305. doi:10.1038/533304a
Lefebvre, E., Moyle, G., Reshef, R., Richman, L. P., Thompson, M., Hong, F., et al. (2016). Antifibrotic Effects of the Dual CCR2/CCR5 Antagonist Cenicriviroc in Animal Models of Liver and Kidney Fibrosis. PLoS One 11 (6), e0158156. doi:10.1371/journal.pone.0158156
Levine, B. L. (2015). Performance-enhancing Drugs: Design and Production of Redirected Chimeric Antigen Receptor (CAR) T Cells. Cancer Gene Ther. 22 (2), 79–84. doi:10.1038/cgt.2015.5
Li, D., He, L., Guo, H., Chen, H., and Shan, H. (2015). Targeting Activated Hepatic Stellate Cells (aHSCs) for Liver Fibrosis Imaging. EJNMMI Res. 5 (1), 71. doi:10.1186/s13550-015-0151-x
Li, D., Li, N., Zhang, Y.-F., Fu, H., Feng, M., Schneider, D., et al. (2020). Persistent Polyfunctional Chimeric Antigen Receptor T Cells that Target Glypican 3 Eliminate Orthotopic Hepatocellular Carcinomas in Mice. Gastroenterology 158 (8), 2250–2265. doi:10.1053/j.gastro.2020.02.011
Li, Y., Shang, W., Liang, X., Zeng, C., Liu, M., Wang, S., et al. (2018). The Diagnosis of Hepatic Fibrosis by Magnetic Resonance and Near-Infrared Imaging Using Dual-Modality Nanoparticles. RSC Adv. 8 (12), 6699–6708. doi:10.1039/C7RA10847H
Li, Z., Ni, M., Yu, H., Wang, L., Zhou, X., Chen, T., et al. (2020). Gut Microbiota and Liver Fibrosis: One Potential Biomarker for Predicting Liver Fibrosis. Biomed. Res. Int. 2020, 1–15. doi:10.1155/2020/3905130
Lipfert, J., Kerssemakers, J. W. J., Dekker, N. H., and Dekker, N. H. (2010). Magnetic Torque Tweezers: Measuring Torsional Stiffness in DNA and RecA-DNA Filaments. Nat. Methods 7 (12), 977–980. doi:10.1038/nmeth.1520
Liu, F., Kc, P., Zhang, G., and Zhe, J. (2016). Microfluidic Magnetic Bead Assay for Cell Detection. Anal. Chem. 88 (1), 711–717. doi:10.1021/acs.analchem.5b02716
Loomba, R., Lawitz, E., Mantry, P. S., Jayakumar, S., Caldwell, S. H., Arnold, H., et al. (2018). The ASK1 Inhibitor Selonsertib in Patients with Nonalcoholic Steatohepatitis: A Randomized, Phase 2 Trial. Hepatology 67 (2), 549–559. doi:10.1002/hep.29514
Lunov, O., Syrovets, T., Büchele, B., Jiang, X., Röcker, C., Tron, K., et al. (2010). The Effect of Carboxydextran-Coated Superparamagnetic Iron Oxide Nanoparticles on C-Jun N-Terminal Kinase-Mediated Apoptosis in Human Macrophages. Biomaterials 31 (19), 5063–5071. doi:10.1016/j.biomaterials.2010.03.023
Magro, M., Martinello, T., Bonaiuto, E., Gomiero, C., Baratella, D., Zoppellaro, G., et al. (2017). Covalently Bound DNA on Naked Iron Oxide Nanoparticles: Intelligent Colloidal Nano-Vector for Cell Transfection. Biochim. Biophys. Acta (Bba) - Gen. Subjects 1861 (11), 2802–2810. doi:10.1016/j.bbagen.2017.07.025
Masuzaki, R., Ray, K. C., Roland, J., Zent, R., Lee, Y. A., and Karp, S. J. (2021). Integrin β1 Establishes Liver Microstructure and Modulates Transforming Growth Factor β during Liver Development and Regeneration1 Establishes Liver Microstructure and Modulates Transforming Growth Factor during Liver Development and Regeneration. Am. J. Pathol. 191 (2), 309–319. doi:10.1016/j.ajpath.2020.10.011
Meurer, S. K., Karsdal, M. A., and Weiskirchen, R. (2020). Advances in the Clinical Use of Collagen as Biomarker of Liver Fibrosis. Expert Rev. Mol. Diagn. 20 (9), 947–969. doi:10.1080/14737159.2020.1814746
Michalopoulos, G. K., and Bhushan, B. (2021). Liver Regeneration: Biological and Pathological Mechanisms and Implications. Nat. Rev. Gastroenterol. Hepatol. 18, 40–55. doi:10.1038/s41575-020-0342-4
Mikulic, D., and Mrzljak, A. (2020). Liver Transplantation and Aging. Wjt 10 (9), 256–266. doi:10.5500/wjt.v10.i9.256
Morales, I., Costo, R., Mille, N., Da Silva, G., Carrey, J., Hernando, A., et al. (2018). High Frequency Hysteresis Losses on γ-Fe2O3 and Fe3O4: Susceptibility as a Magnetic Stamp for Chain Formation. Nanomaterials 8 (12), 970. doi:10.3390/nano8120970
Mukherjee, S., Liang, L., and Veiseh, O. (2020). Recent Advancements of Magnetic Nanomaterials in Cancer Therapy. Pharmaceutics 12 (2), 147. doi:10.3390/pharmaceutics12020147
Nagórniewicz, B., Mardhian, D. F., Booijink, R., Storm, G., Prakash, J., and Bansal, R. (2019). Engineered Relaxin as Theranostic Nanomedicine to Diagnose and Ameliorate Liver Cirrhosis. Nanomedicine: Nanotechnology, Biol. Med. 17, 106–118. doi:10.1016/j.nano.2018.12.008
Nascimbeni, F., Lugari, S., Cassinerio, E., Motta, I., Cavicchioli, A., Dalla Salda, A., et al. (2020). Liver Steatosis Is Highly Prevalent and Is Associated with Metabolic Risk Factors and Liver Fibrosis in Adult Patients with Type 1 Gaucher Disease. Liver Int. 40 (12), 3061–3070. doi:10.1111/liv.14640
Ng, H. H., Leo, C. H., Parry, L. J., and Ritchie, R. H. (2018). Relaxin as a Therapeutic Target for the Cardiovascular Complications of Diabetes. Front. Pharmacol. 9, 501. doi:10.3389/fphar.2018.00501
Nguyen, T., Mammeri, F., and Ammar, S. (2018). Iron Oxide and Gold Based Magneto-Plasmonic Nanostructures for Medical Applications: A Review. Nanomaterials 8 (3), 149. doi:10.3390/nano8030149
Nielsen, M. J., Karsdal, M. A., Krag, A., and Leeming, D. J. (2019). Extracellular Matrix Remodeling with Focus on Biochemical Markers in Liver Fibrosis: Limitations and Possibilities. The Human Gut-Liver-Axis in Health and Disease. Springer.
Nielsen, M. J., Veidal, S. S., Karsdal, M. A., Ørsnes-Leeming, D. J., Vainer, B., Gardner, S. D., et al. (2015). Plasma Pro-C3 (N-Terminal Type III Collagen Propeptide) Predicts Fibrosis Progression in Patients with Chronic Hepatitis C. Liver Int. 35 (2), 429–437. doi:10.1111/liv.12700
Nikitin, M. P., Orlov, A. V., Sokolov, I. L., Minakov, A. A., Nikitin, P. I., Ding, J., et al. (2018). Ultrasensitive Detection Enabled by Nonlinear Magnetization of Nanomagnetic Labels. Nanoscale 10 (24), 11642–11650. doi:10.1039/C8NR01511B
Noh, S.-h., Na, W., Jang, J.-t., Lee, J.-H., Lee, E. J., Moon, S. H., et al. (2012). Nanoscale Magnetism Control via Surface and Exchange Anisotropy for Optimized Ferrimagnetic Hysteresis. Nano Lett. 12 (7), 3716–3721. doi:10.1021/nl301499u
Novo, E., Bocca, C., Foglia, B., Protopapa, F., Maggiora, M., Parola, M., et al. (2020). Liver Fibrogenesis: Un Update on Established and Emerging Basic Concepts. Arch. Biochem. Biophys. 689, 108445. doi:10.1016/j.abb.2020.108445
Okina, Y., Sato-Matsubara, M., Matsubara, T., Daikoku, A., Longato, L., Rombouts, K., et al. (2020). TGF-β1-driven Reduction of Cytoglobin Leads to Oxidative DNA Damage in Stellate Cells during Non-alcoholic Steatohepatitis. J. Hepatol. 73 (4), 882–895. doi:10.1016/j.jhep.2020.03.051
Omelyanchik, A., Levada, E., Ding, J., Lendinez, S., Pearson, J., Efremova, M., et al. (2018). Design of Conductive Microwire Systems for Manipulation of Biological Cells. IEEE Trans. Magn. 54 (6), 1–5. doi:10.1109/TMAG.2018.2819823
Palomer, X., Barroso, E., Pizarro-Delgado, J., Peña, L., Botteri, G., Zarei, M., et al. (2018). Pparβ/δ: A Key Therapeutic Target in Metabolic Disorders/: a Key Therapeutic Target in Metabolic Disorders. Ijms 19 (3), 913. doi:10.3390/ijms19030913
Parola, M., and Pinzani, M. (2019). Liver Fibrosis: Pathophysiology, Pathogenetic Targets and Clinical Issues. Mol. Aspects Med. 65, 37–55. doi:10.1016/j.mam.2018.09.002
Peng, Z.-W., Ikenaga, N., Liu, S. B., Sverdlov, D. Y., Vaid, K. A., Dixit, R., et al. (2016). Integrin αvβ6 Critically Regulates Hepatic Progenitor Cell Function and Promotes Ductular Reaction, Fibrosis, and Tumorigenesisv6 Critically Regulates Hepatic Progenitor Cell Function and Promotes Ductular Reaction, Fibrosis, and Tumorigenesis. Hepatology 63 (1), 217–232. doi:10.1002/hep.28274
Pi, S., Liu, W., and Jiang, T. (2018). Real-time and Quantitative Isotropic Spatial Resolution Susceptibility Imaging for Magnetic Nanoparticles. Meas. Sci. Technol. 29 (3), 035402. doi:10.1088/1361-6501/aa9a55
Piazzolla, V. A., and Mangia, A. (2020). Noninvasive Diagnosis of NAFLD and NASH. Cells 9 (4), 1005. doi:10.3390/cells9041005
Polyzos, S. A., Kang, E. S., Boutari, C., Rhee, E.-J., and Mantzoros, C. S. (2020). Current and Emerging Pharmacological Options for the Treatment of Nonalcoholic Steatohepatitis. Metabolism 111, 154203. doi:10.1016/j.metabol.2020.154203
Prasad, R., Chauhan, D. S., Yadav, A. S., Devrukhkar, J., Singh, B., Gorain, M., et al. (2018). A Biodegradable Fluorescent Nanohybrid for Photo-Driven Tumor Diagnosis and Tumor Growth Inhibition. Nanoscale 10 (40), 19082–19091. doi:10.1039/C8NR05164J
Qiu, X., Li, Z., Han, X., Zhen, L., Luo, C., Liu, M., et al. (2019). Tumor-derived Nanovesicles Promote Lung Distribution of the Therapeutic Nanovector through Repression of Kupffer Cell-Mediated Phagocytosis. Theranostics 9 (9), 2618–2636. doi:10.7150/thno.32363
Quini, C. C., Próspero, A. G., Calabresi, M. F. F., Moretto, G. M., Zufelato, N., Krishnan, S., et al. (2017). Real-time Liver Uptake and Biodistribution of Magnetic Nanoparticles Determined by AC Biosusceptometry. Nanomedicine: Nanotechnology, Biol. Med. 13 (4), 1519–1529. doi:10.1016/j.nano.2017.02.005
Ramachandran, P., Lee, C. Y., Doong, R.-A., Oon, C. E., Kim Thanh, N. T., and Lee, H. L. (2020). A Titanium Dioxide/nitrogen-Doped Graphene Quantum Dot Nanocomposite to Mitigate Cytotoxicity: Synthesis, Characterisation, and Cell Viability Evaluation. RSC Adv. 10 (37), 21795–21805. doi:10.1039/D0RA02907F
Rezvani, K., Rouce, R., Liu, E., and Shpall, E. (2017). Engineering Natural Killer Cells for Cancer Immunotherapy. Mol. Ther. 25 (8), 1769–1781. doi:10.1016/j.ymthe.2017.06.012
Rieck, S., Heun, Y., Heidsieck, A., Mykhaylyk, O., Pfeifer, A., Gleich, B., et al. (2019). Local Anti-angiogenic Therapy by Magnet-Assisted Downregulation of SHP2 Phosphatase. J. Controlled Release 305, 155–164. doi:10.1016/j.jconrel.2019.05.031
Roca, A. G., Gutiérrez, L., Gavilán, H., Fortes Brollo, M. E., Veintemillas-Verdaguer, S., and Morales, M. d. P. (2019). Design Strategies for Shape-Controlled Magnetic Iron Oxide Nanoparticles. Adv. Drug Deliv. Rev. 138, 68–104. doi:10.1016/j.addr.2018.12.008
Roth, J. D., Veidal, S. S., Fensholdt, L. K. D., Rigbolt, K. T. G., Papazyan, R., Nielsen, J. C., et al. (2019). Combined Obeticholic Acid and Elafibranor Treatment Promotes Additive Liver Histological Improvements in a Diet-Induced Ob/ob Mouse Model of Biopsy-Confirmed NASH. Sci. Rep. 9 (1), 9046. doi:10.1038/s41598-019-45178-z
Sadighian, J. C., Crawford, M. L., Suder, T. W., and Wong, C. Y. (2020). Surface Ligation Stage Revealed through Polarity-dependent Fluorescence during Perovskite Nanocrystal Growth. J. Mater. Chem. C 8 (21), 7041–7050. doi:10.1039/C9TC06545H
Salarian, M., Ibhagui, O. Y., and Yang, J. J. (2020). Molecular Imaging of Extracellular Matrix Proteins with Targeted Probes Using Magnetic Resonance Imaging. WIREs Nanomed Nanobiotechnol 12 (4), e1622. doi:10.1002/wnan.1622
Salvador, M., Moyano, A., Martínez-García, J. C., Blanco-López, M. C., and Rivas, M. (2019). Synthesis of Superparamagnetic Iron Oxide Nanoparticles: SWOT Analysis towards Their Conjugation to Biomolecules for Molecular Recognition Applications. J. Nanosci. Nanotechnol. 19 (8), 4839–4856. doi:10.1166/jnn.2019.16931
Santos, A., and Lagares, D. (2018). Matrix Stiffness: The Conductor of Organ Fibrosis. Curr. Rheumatol. Rep. 20 (1), 2. doi:10.1007/s11926-018-0710-z
Saraswathy, A., Nazeer, S. S., Jeevan, M., Nimi, N., Arumugam, S., Harikrishnan, V. S., et al. (2014). Citrate Coated Iron Oxide Nanoparticles with Enhanced Relaxivity for In Vivo Magnetic Resonance Imaging of Liver Fibrosis. Colloids Surf. B: Biointerfaces 117, 216–224. doi:10.1016/j.colsurfb.2014.02.034
Sato, K., Meng, F., Giang, T., Glaser, S., and Alpini, G. (2018). Mechanisms of Cholangiocyte Responses to Injury. Biochim. Biophys. Acta (Bba) - Mol. Basis Dis. 1864 (4), 1262–1269. doi:10.1016/j.bbadis.2017.06.017
Schuster-Gaul, S., Geisler, L. J., McGeough, M. D., Johnson, C. D., Zagorska, A., Li, L., et al. (2020). ASK1 Inhibition Reduces Cell Death and Hepatic Fibrosis in an Nlrp3 Mutant Liver Injury Model. JCI Insight 5 (2), e123294. doi:10.1172/jci.insight.123294
Sehl, O. C., Gevaert, J. J., Melo, K. P., Knier, N. N., and Foster, P. J. (2020). A Perspective on Cell Tracking with Magnetic Particle Imaging. Tomography 6 (4), 315–324. doi:10.18383/j.tom.2020.00043
Setua, S., Jaggi, M., Yallapu, M. M., Chauhan, S. C., Danilushkina, A., Lee, H., et al. (2018). Targeted and Theranostic Applications for Nanotechnologies in Medicine. Nanotechnologies in Preventive and Regenerative Medicine. Elsevier.
Sharifi, S., Seyednejad, H., Laurent, S., Atyabi, F., Saei, A. A., and Mahmoudi, M. (2015). Superparamagnetic Iron Oxide Nanoparticles Forin Vivomolecular and Cellular Imaging. Contrast Media Mol. Imaging 10 (5), 329–355. doi:10.1002/cmmi.1638
Sheka, A. C., Adeyi, O., Thompson, J., Hameed, B., Crawford, P. A., and Ikramuddin, S. (2020). Nonalcoholic Steatohepatitis. JAMA 323 (12), 1175–1183. doi:10.1001/jama.2020.2298
Shim, J. K., and Hur, W. (2017). Cellular Uptake of Fibroin Micro/nanoparticles as a Method for Three-Dimensional Culture under Magnetic Levitation Conditions. Proceedings of the 2nd World Congress on Recent Advances in Nanotechnology (RAN’17), 115. Spain: Barcelona. doi:10.11159/nddte17.115April 4-6, 2017), Paper NoNDDTE
Silva, F. G. d., Depeyrot, J., Campos, A. F. C., Aquino, R., Fiorani, D., and Peddis, D. (2019). Structural and Magnetic Properties of Spinel Ferrite Nanoparticles. J. Nanosci. Nanotechnol. 19 (8), 4888–4902. doi:10.1166/jnn.2019.16877
Simkin, J., Dawson, L. A., Simkin, M., and Muneoka, K. (2020). Healing Power: The Mammalian Macrophage in Skeletal Regeneration, Scar Formation, and Regenerative Medicine. J. Immunol. Regenerative Med. 7, 100026. doi:10.1016/j.regen.2019.100026
Socoliuc, V., Peddis, D., Petrenko, V. I., Avdeev, M. V., Susan-Resiga, D., Szabó, T., et al. (2020). Magnetic Nanoparticle Systems for Nanomedicine-A Materials Science Perspective. Magnetochemistry 6 (1), 2. doi:10.3390/magnetochemistry6010002
Sosa, R. A., Rossetti, M., Naini, B. V., Groysberg, V. M., Kaldas, F. M., Busuttil, R. W., et al. (2020). Pattern Recognition Receptor-Reactivity Screening of Liver Transplant Patients. Ann. Surg. 271 (5), 922–931. doi:10.1097/SLA.0000000000003085
Stolfi, C., Troncone, E., Marafini, I., and Monteleone, G. (2021). Role of TGF-Beta and Smad7 in Gut Inflammation, Fibrosis and Cancer. Biomolecules 11 (1), 17. doi:10.3390/biom11010017
Svetlichnyi, V. A., Shabalina, A. V., Lapin, I. N., Goncharova, D. A., Velikanov, D. A., and Sokolov, A. E. (2017). Characterization and Magnetic Properties Study for Magnetite Nanoparticles Obtained by Pulsed Laser Ablation in Water. Appl. Phys. A. 123 (12), 763. doi:10.1007/s00339-017-1390-7
Tandon, P., and Berzigotti, A. (2020). Management of Lifestyle Factors in Individuals with Cirrhosis: A Pragmatic Review. Seminars in Liver Disease. Thieme Medical Publishers.
Thomas, C. R., Ferris, D. P., Lee, J.-H., Choi, E., Cho, M. H., Kim, E. S., et al. (2010). Noninvasive Remote-Controlled Release of Drug Molecules In Vitro Using Magnetic Actuation of Mechanized Nanoparticles. J. Am. Chem. Soc. 132 (31), 10623–10625. doi:10.1021/ja1022267
Tomitaka, A., Kaushik, A., Kevadiya, B. D., Mukadam, I., Gendelman, H. E., Khalili, K., et al. (2019). Surface-engineered Multimodal Magnetic Nanoparticles to Manage CNS Diseases. Drug Discov. Today 24 (3), 873–882. doi:10.1016/j.drudis.2019.01.006
Toth, G. B., Varallyay, C. G., Horvath, A., Bashir, M. R., Choyke, P. L., Daldrup-Link, H. E., et al. (2017). Current and Potential Imaging Applications of Ferumoxytol for Magnetic Resonance Imaging. Kidney Int. 92 (1), 47–66. doi:10.1016/j.kint.2016.12.037
Trautwein, C., Friedman, S. L., Schuppan, D., and Pinzani, M. (2015). Hepatic Fibrosis: Concept to Treatment. J. Hepatol. 62 (1), S15–S24. doi:10.1016/j.jhep.2015.02.039
Trujillo-Alonso, V., Pratt, E. C., Zong, H., Lara-Martinez, A., Kaittanis, C., Rabie, M. O., et al. (2019). FDA-approved Ferumoxytol Displays Anti-leukaemia Efficacy against Cells with Low Ferroportin Levels. Nat. Nanotechnol. 14 (6), 616–622. doi:10.1038/s41565-019-0406-1
Ungefroren, H., Gieseler, F., Kaufmann, R., Settmacher, U., Lehnert, H., and Rauch, B. (2018). Signaling Crosstalk of TGF-Β/alk5 and PAR2/PAR1: A Complex Regulatory Network Controlling Fibrosis and Cancer/ALK5 and PAR2/PAR1: A Complex Regulatory Network Controlling Fibrosis and Cancer. Ijms 19 (6), 1568. doi:10.3390/ijms19061568
Van de Walle, A., Kolosnjaj-Tabi, J., Lalatonne, Y., and Wilhelm, C. (2020). Ever-evolving Identity of Magnetic Nanoparticles within Human Cells: The Interplay of Endosomal Confinement, Degradation, Storage, and Neocrystallization. Acc. Chem. Res. 53 (10), 2212–2224. doi:10.1021/acs.accounts.0c00355
van Dijk, F., Teekamp, N., Beljaars, L., Post, E., Zuidema, J., Steendam, R., et al. (2018). Pharmacokinetics of a Sustained Release Formulation of PDGFβ-Receptor Directed Carrier Proteins to Target the Fibrotic Liver-Receptor Directed Carrier Proteins to Target the Fibrotic Liver. J. Controlled Release 269, 258–265. doi:10.1016/j.jconrel.2017.11.029
Wang, X., Ho, C., Tsatskis, Y., Law, J., Zhang, Z., Zhu, M., et al. (2019). Intracellular Manipulation and Measurement with Multipole Magnetic Tweezers. Sci. Robot. 4 (28), eaav6180. doi:10.1126/scirobotics.aav6180
Wei, Y., Zhao, M., Yang, F., Mao, Y., Xie, H., and Zhou, Q. (2016). Iron Overload by Superparamagnetic Iron Oxide Nanoparticles Is a High Risk Factor in Cirrhosis by a Systems Toxicology Assessment. Sci. Rep. 6 (1), 29110. doi:10.1038/srep29110
Wu, L.-P., Wang, D., and Li, Z. (2020). Grand Challenges in Nanomedicine. Mater. Sci. Eng. C 106, 110302. doi:10.1016/j.msec.2019.110302
Wu, W., Wu, Z., Yu, T., Jiang, C., and Kim, W.-S. (2015). Recent Progress on Magnetic Iron Oxide Nanoparticles: Synthesis, Surface Functional Strategies and Biomedical Applications. Sci. Tech. Adv. Mater. 16 (2), 023501. doi:10.1088/1468-6996/16/2/023501
Xiao, J., Liu, P., Wang, C. X., and Yang, G. W. (2017). External Field-Assisted Laser Ablation in Liquid: An Efficient Strategy for Nanocrystal Synthesis and Nanostructure Assembly. Prog. Mater. Sci. 87, 140–220. doi:10.1016/j.pmatsci.2017.02.004
Xiao, Y., Liu, R., Li, X., Gurley, E. C., Hylemon, P. B., Lu, Y., et al. (2019). Long Noncoding RNA H19 Contributes to Cholangiocyte Proliferation and Cholestatic Liver Fibrosis in Biliary Atresia. Hepatology 70 (5), 1658–1673. doi:10.1002/hep.30698
Yue, M., Zhang, X., and Liu, J. P. (2017). Fabrication of Bulk Nanostructured Permanent Magnets with High Energy Density: Challenges and Approaches. Nanoscale 9 (11), 3674–3697. doi:10.1039/C6NR09464C
Zajicek, G., Oren, R., and Weinreb, M. (1985). The Streaming Liver. Liver 5 (6), 293–300. doi:10.1111/j.1600-0676.1985.tb00252.x
Zamay, T. N., Zamay, G. S., Belyanina, I. V., Zamay, S. S., Denisenko, V. V., Kolovskaya, O. S., et al. (2017). Noninvasive Microsurgery Using Aptamer-Functionalized Magnetic Microdisks for Tumor Cell Eradication. Nucleic Acid Ther. 27 (2), 105–114. doi:10.1089/nat.2016.0634
Zhang, C., Liu, H., Cui, Y., Li, X., Zhang, Z., Zhang, Y., et al. (2016). Molecular Magnetic Resonance Imaging of Activated Hepatic Stellate Cells with Ultrasmall Superparamagnetic Iron Oxide Targeting Integrin αvβ₃ for Staging Liver Fibrosis in Rat Model. Int. J. Nanomedicine 11, 1097–1108. doi:10.2147/IJN.S101366
Zhang, K., Han, Y., Hu, Z., Zhang, Z., Shao, S., Yao, Q., et al. (2019). SCARNA10, a Nuclear-Retained Long Non-coding RNA, Promotes Liver Fibrosis and Serves as a Potential Biomarker. Theranostics 9 (12), 3622–3638. doi:10.7150/thno.32935
Zhou, T. (2020). Mesenchymal Phenotype of Cholangiocytes Plays an Integral Role in the Regulation of Biliary Senescence and Liver Fibrosis in Cholestatic Liver Diseases. Doctoral Dissertation. Bryan-College Station, TX: Texas A&M UniversityAvailable electronically from https://hdl.handle.net/1969.1/191950.
Keywords: liver fibrosis, magnetic nanoparticles, nanomedicine, theranostics, drug delivery
Citation: Eftekhari A, Arjmand A, Asheghvatan A, Švajdlenková H, Šauša O, Abiyev H, Ahmadian E, Smutok O, Khalilov R, Kavetskyy T and Cucchiarini M (2021) The Potential Application of Magnetic Nanoparticles for Liver Fibrosis Theranostics. Front. Chem. 9:674786. doi: 10.3389/fchem.2021.674786
Received: 02 March 2021; Accepted: 03 May 2021;
Published: 14 May 2021.
Edited by:
Raghvendra Singh Yadav, Tomas Bata University in Zlín, CzechiaReviewed by:
Jie Shen, Zhejiang University City College, ChinaBaolin Zhang, Guilin University of Technology, China
Zhang Sheng Jian, Fudan University, China
Copyright © 2021 Eftekhari, Arjmand, Asheghvatan, Švajdlenková, Šauša, Abiyev, Ahmadian, Smutok, Khalilov, Kavetskyy and Cucchiarini. This is an open-access article distributed under the terms of the Creative Commons Attribution License (CC BY). The use, distribution or reproduction in other forums is permitted, provided the original author(s) and the copyright owner(s) are credited and that the original publication in this journal is cited, in accordance with accepted academic practice. No use, distribution or reproduction is permitted which does not comply with these terms.
*Correspondence: Elham Ahmadian, YWhtYWRpYW4uZWxoYW1AeWFob28uY29t; Taras Kavetskyy, a2F2ZXRza3l5QHlhaG9vLmNvbQ==; Magali Cucchiarini, bW1jdWNjaGlhcmluaUBob3RtYWlsLmNvbQ==
†These authors have contributed equally to this work