- 1The National and Local Joint Engineering Research Center for Biomanufacturing of Chiral Chemicals, Zhejiang University of Technology, Hangzhou, China
- 2Key Laboratory of Bioorganic Synthesis of Zhejiang, College of Biotechnology and Bioengineering, Zhejiang University of Technology, Hangzhou, China
L-methionine is an important natural amino acid with broad application prospects. A novel gene encoding the enzyme with the ability to catalyze O-succinyl-L-homoserine (OSH) to L-methionine was screened and characterized. The recombinant O-succinyl-L-homoserine sulfhydrylase from Thioalkalivibrio sulfidiphilus (tsOSHS) exhibited maximum activity at 35°C and pH 6.5. OSHS displayed an excellent thermostability with a half-life of 21.72 h at 30°C. Furthermore, the activity of OSHS increased 115% after Fe2+ added. L-methionine was obtained with a total yield reaching 42.63 g/L under the concentration of O-succinyl-L-homoserine 400 mM (87.6 g/L). These results indicated that OSHS is a potential candidate for applying in the large-scale bioproduction of L-methionine.
Highlights
- An enzyme with high activity that can convert O-succinyl-L-homoserine to L-methionine was screened.
- The protein sequence of the enzyme was identified.
- The enzymatic properties of the enzyme were studied and it has high thermostability.
- O-succinyl-L-homoserine sulfhydrylase was used in the L-methionine catalysis with high substrate concentration.
Introduction
L-amino acid is the smallest component of protein, which is the basic life functional unit. L-amino acid has many important functions, such as providing nutrition for organism, protecting organism through immune system, participating in physiological metabolism process. In addition, with the understanding of L-amino acids, they are more and more used in food, pharmaceutical, cosmetics, feed additives and many other fields (Krämer, 2004). Among them, L-methionine is an important sulfur-containing essential amino acid, which participates in protein synthesis (Zhu et al., 2020). L-methionine is also the main methyl donor in many physiological metabolism processes, and the first limiting amino acid in animal nutrition (Tang et al., 2020). Much attentions have been paid to L-methionine as its tremendous demand used in various fields (Willke, 2014).
At present, L-methionine is mainly produced by chemical method. Methylthiopropionaldehyde was firstly synthesized from methyl mercaptan and acrolein, then hydantoin was synthesized from methylthiopropionaldehyde, HCN, NH3 and CO2. Hydantoin was then hydrolyzed to potassium methionine in the presence of KHCO3. Finally, methionine was obtained by acidification (Franke et al., 2003). In 1850, A. Strecker proposed that cyanohydrin was synthesized from acrolein, methyl mercaptan and hydrocyanic acid, and then hydrolyzed to hydroxymethionine and ammonium sulfate in the presence of sulfuric acid (Schonberg and Moubacher, 1952). In addition, the biological production of L-methionine was studied. Huang et al. rationally modified the metabolic pathway of E. coli W3110 by metabolic engineering technology to realize the production of L-methionine with a yield of 9.75 g/L (Huang et al., 2017). It has also been reported that E. coli MG1655 was developed to produce 35 g/L of L-methionine (Brazeau et al., 2013).
Besides, enzyme catalysis is also an important production route to obtain high yield of L-methionine. L-N-carbamoyl hydrolase was used to catalyze the cleavage of amide bond of N-carbamoyl methionine to produce L-methionine with the highest yield of 34.4% (Yamada et al., 1979). N-acetylamino acid racemase and amidase were coupled for racemization and transformation simultaneously to produce L-methionine with D, L-N-acetylmethionine as the substrate, the yield could reach 99% (Tokuyama and Hatano, 1996).
CJ Cheiljedang Co., Ltd. developed a route coupling fermentation and enzymatic catalysis, which used sucrose as a substrate to produce O-succinyl-L-homoserine (Hong et al., 2014) and O-acetyl-L-homoserine through fermentation. Then, it reacted with methyl mercaptan under the action of sulfhydrylase to produce L-methionine (Kim et al., 2015). At present, this route has the highest L-methionine production capacity through biological methods, and has already been industrialized.
Pyridoxal-5′-Phosphate (PLP)-dependent enzymes can catalyze various reactions in amino acid metabolism, including transamination, racemization, decarboxylation, and side chain elimination and replacement. At present, there are many enzymes belonging to the PLP-dependent enzyme superfamily (Christen and Mehta, 2001; Eliot and Kirsch, 2003). Among them, cystathionine synthase plays an important role in the sulfur metabolism pathways in plants, animals, and microorganisms (Zuhra et al., 2020). Cysthionine synthase could be divided into cysthionine β-synthase (α, β-elimination) and cysthionine-γ-synthase(α, γ-elimination) due to their different catalytic sites. Although both can catalyze the biosynthesis of L-cysthionine, they belong to the different folding types of PLP-dependent enzymes (Aitken and Kirsch, 2005). Cysthionine-β-Synthase (CBS) is the first and rate-limiting enzyme in the transsulfurization reaction and paticipated in many enzymatic processes (Mudd et al., 1965; Braunstein et al., 1969; Tudball and Reed, 1975; Kraus and Rosenberg, 1983). One of the features that distinguishes CBS from the other PLP-dependent enzymes is its N-terminus containing a heme-binding site (Zuhra et al., 2020).
Cysthionine-γ-Synthase (CGS) only existed in plants and bacteria (Aitken and Kirsch, 2005). It is a particularly interesting member of the γ-subfamily of fold-type I as it catalyzes a γ-replacement reaction that is unique among PLP-dependent enzymes (Brzovic et al., 1990). It is exhibited as the first specific enzyme for the methionine biosynthetic pathway. Nowadays, CGS has been extensively purified and characterized in Salmonella thyphimurium, Escherichia coli, and Bacillus Sphcericus (Kaplan and Flavin, 1966; Holbrook et al., 1990). However, the characterization of enzymatic properties directly applied to the synthesis of L-methionine in vitro has not been reported yet.
Therefore, in this study, 12 sequences with 50–90% homology were synthesized and overexpressed in E. coli BL21 (DE3). The sequence with the highest conversion rate was screened, and the enzymatic properties of OSHS were studied for the first time. OSHS with high thermostability, high activity, and high substrate tolerance and other characteristics, was exhibited the potentiality to be a biocatalyst for industrial production of L-methionine.
Materials and Methods
Chemicals
O-succinyl-L-homoserine and sodium methyl mercaptan were purchased from J&K Chemical Technology (Shanghai, China). L-methionine and PLP were purchased from Aladdin reagent Co. Ltd. (Shanghai, China). Kanamycin and isopropyl-β-D-thiogalactopyranoside (IPTG) were obtained from Sigma Chemical Co. (St. Louis, MO, USA). All other chemicals were of analytical grade and were commercially available.
Plasmids, Bacterial Strains, and Culture Conditions
E. coli DH5α (TSINGKE Biotech Co., Hangzhou, China) was used as the host for gene cloning, and E. coli BL21(DE3) was the host for protein expression. The plasmid pET28b was used as the expression vector in E. coli. The E. coli was grown in Luria Bertani (LB) medium (yeast extract 5 g/L, peptone 10 g/L, NaCl 10 g/L) with 50 mg/L kanamycin.
Screening, Identification, and Purification
Twelve genes were synthesized artificially according to the sequence deposited in the NCBI Protein database under accession number WP_016715902.1 (Kim et al., 2015). For expression purpose, the genes were subcloned to the expression vector pET28b between restriction sites NcoI and XhoI, and then the recombinant plasmids were transformed into the E. coli strain BL21(DE3). After the recombinant E. coli cells were cultivated in LB medium containing kanamycin (50 mg/L) at 37°C for 4 h, IPTG was added for induction and the cells were cultured at 28°C for 12 h. Recombinant OSHS was expressed intracellularly, and the pellet was collected by centrifugation at 12,000 rpm and 4°C for 10 min. To purify the recombinant OSHS, the centrifuged cell pellet was washed twice with 0.85% NaCl solution, and then resuspended in 50 mM sodium phosphate buffer (pH 7.0), and sonicated in ice bath. After sonication, the cell lysate was centrifuged at 8,000 rpm for 30 min at 4°C to remove cell debris. Then the supernatant was filtered through a 0.22 μm filter. The filtrate was further purified by a chelating agarose fast-flow resin column (1.0 × 10.0 cm) containing Ni2+, and the binding buffer (50 mM sodium phosphate buffer, pH 7.0) was used for balance. Then, proteins were eluted with a linear imidazole gradient of 20–500 mM in 50 mM sodium phosphate buffer (pH 7.0). The protein was analyzed by sodium dodecyl sulfate polyacrylamide gel electrophoresis (SDS-PAGE) to determine the protein purity. The protein concentration was determined by the protein determination kit (Keygen Biotech, China). Thereafter, the purified enzyme was used in characterization experiments. Its kinetics and catalytic properties were determined.
Enzyme Assay
The enzyme activity of recombinant OSHS was determined by measuring the conversion rate of OSH to L-methionine and the process was depicted by the reaction equation (Scheme 1). The system was carried out in a 500 μL system, which contained 50 mM Phosphate Buffered Saline (PBS) (pH 7.0), 50 mM OSH, 40 μL sodium methyl mercaptan and 15 μg purified enzyme at 30°C for 5 min. One unit (1U) is defined as the amount of enzyme catalyzing the production of 1 μM L-methionine from OSH per minute at 30°C. The L-methionine content was determined by amino acid analyzer.
Effects of pH and Temperature on the Activity of OSHS
The influence of pH on the activity of the purified enzyme was performed at different pH (4.5–9.0). 100 mM OSH and 5% (v/v) sodium methyl mercaptan was biocatalyzed at 30°C for 10 min in three buffers with different pH range, citric acid-sodium citrate (pH 4.5–6.0), Na2HPO4-NaH2PO4 (pH 6.0-8), and Tris-HCl (pH 8.0–9.0). The pH stability was determined by measuring the residual activity after incubating the enzyme in buffers at pH 4.5–9.0 at 4°C for up to 10 h.
To investigate the optimal temperature, the reactions were carried out with 100 mM OSH, 5% (v/v) sodium methyl mercaptan, pH 7.0 adjusted with 50 mM PBS, and the temperature range was 20–60°C. The thermostability was analyzed by measuring the residual enzyme activity of the purified OSHS, which was incubated at 20 to 60°C for 25 h. The half-life of the enzyme was determined by calculating the change in Ln (relative activity) over time.
Effects of Metal Ions and Organic Solvents on OSHS Activity
The effect of metal ions (CaCl2, MgSO4, FeCl3, FeCl2, CuSO4, MnCl2. ZnSO4, NiCl2, AlCl3, CoCl2) on purified OSHS activity were investigated at final concentrations of 1 and 5 mM, respectively. Effects of SDS, EDTA were investigated using the final concentration of 1 and 5 mM, respectively. All reactions were performed with 50 mM OSH, 10 mM PLP and 5% (v/v) sodium methyl mercaptan at 30°C, and the pH was adjusted to 6.5 with 50 mM PBS. The concentration of purified OSHS in the reaction was 30 mg/L.
Kinetic Analysis
The kinetic parameters Km (Michaelis-Menten constant) and Vmax (maximum initial reaction rate) of OSHS were determined by using OSH as the substrate in the PBS at an initial rate ranging from 1 to 500 mM (pH 6.5). Before adding purified tsOSHS (30 mg/L), the reaction mixture was pre-incubated at 30°C for 10 min. The catalytic reaction was performed as a standard enzyme activity determination. Km and kcat were calculated by a non-linear regression method based on a function of the initial velocity data plotted against the substrate concentration.
Biosynthesis of L-methionine Using Recombinant OSHS
Enzymatic synthesis of L-methionine was carried out in 200 of 500 mL shake flask under the optimum conditions. Samples were taken regularly to measure the concentration of L-methionine until the reaction reached equilibrium.
Purified OSHS enzyme with a final concentration of 30 mg/L was used to study the effect of substrate concentration on the enzymatic hydrolysis reaction in various concentrations of OSH (10–400 mM) in a reaction system with 10 mM PLP and 10% (v/v) sodium methyl mercaptan. The reactions were carried out at 30°C for 240 min. Under the condition of 400 mM OSH, 10 mM PLP and 10% (v/v) sodium methyl mercaptan, 30–100 mg/L purified OSHS was used to evaluate the effect of catalyst dosage on the enzymatic reaction. The reactions were carried out at 30°C for 240 min. Under the condition of 400 mM OSH, 100 mg/L purified OSHS and 10% (v/v) sodium methyl mercaptan, 1–20 mM PLP was used to investigate the effect of coenzyme on the catalyst. The reactions were carried out at 30°C for 140 min.
Analytical Methods and Statistical Analysis
All the reactions were terminated using 100 μL 6 M HCl (10% v/v) for 10 min. The samples were diluted 100-fold and then centrifuged for 5 min at 12,000 rpm. Supernatant was collected and used for assay. Amino acids were detected using an automatic amino acid analyzer (SYKAM S-433D, SYKAM, München, BY, Germany) (El-Naggar et al., 2017; Huang et al., 2017). All experiments were replicated three times.
Results and Discussion
Gene Selection and Sequence Analysis
In order to obtain an enzyme could convert OSH into L-methionine, gene mining approach was used to identify novel enzyme with high yield and the potential for industrial application. A reported OSHS from Pseudomonas could convert OSH into L-methionine (Kim et al., 2015) was used to perform a BLAST search in the nation center for biotechnology information database. The identity and conversion rate were listed in Table 1. Among them, WP_095206569.1 showed the highest conversion rate. Therefore, the protein sequence of this enzyme was aligned with the reported OSHS (WP_016715902.1) and one of the eCGS (KFF54031.1) (Figure 1). It was found that the three sequences shared the same conservative site in Y46, R48, R361, K198, and showed different in Y101 which was not directly involved in catalysis (Jaworski et al., 2012). Besides, the sequence analysis indicated that tsOSHS had a predicted molecular weight of 42736.1 Da and a predicted isoelectric point of 5.85.
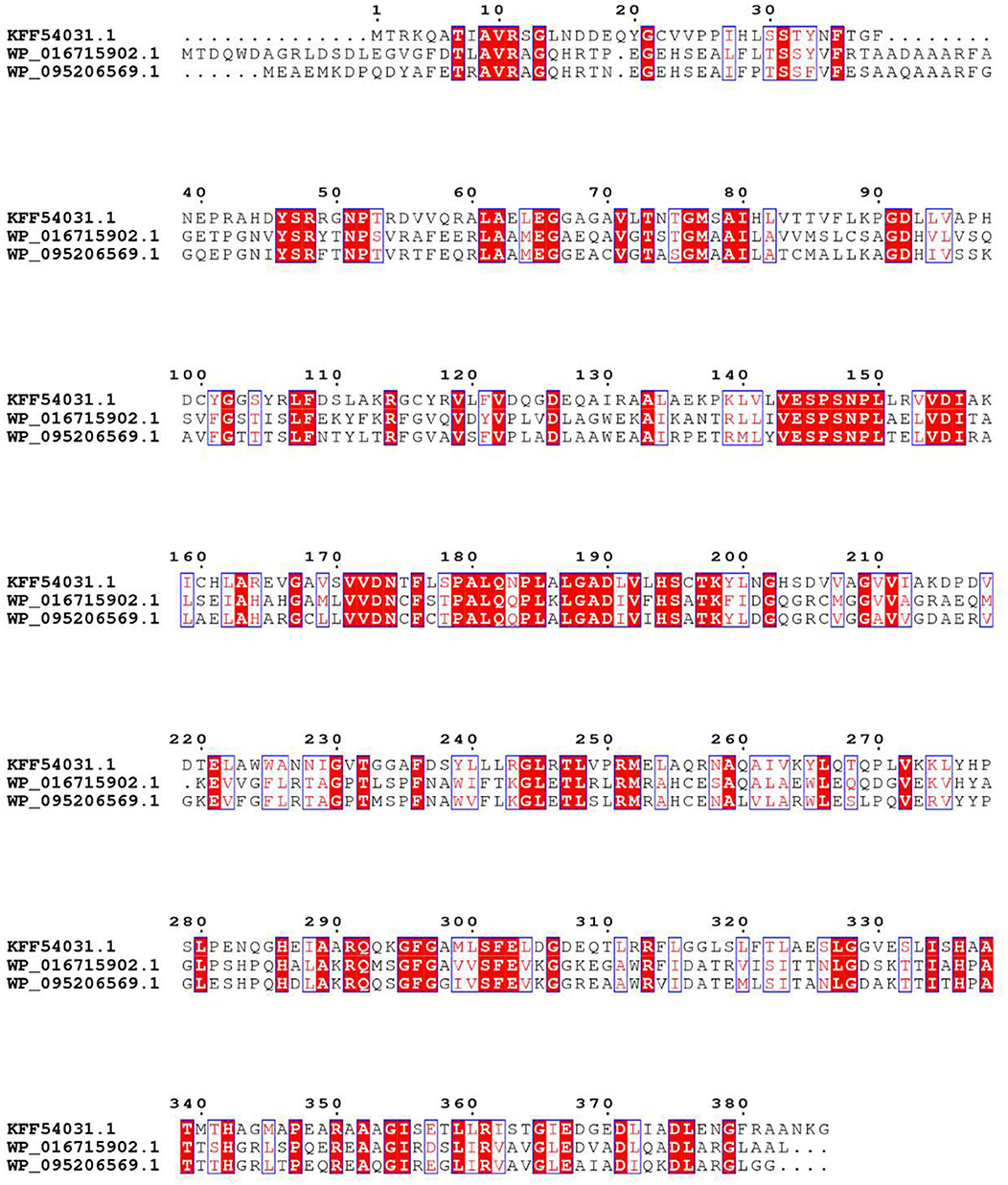
Figure 1. Alignment of the amino acid sequences. The origins and their GenBank NO.s are as follows: E. coli (KFF54031.1); Pseudomonas (WP_016715902.1); T. sulfidiphilus (WP_095206569.1).
Phylogenetic Analysis of tsOSHS
OSHS showed 71% amino acid sequence identity with OSHS from Thioalkalivibrio sulfidiphilus (GenBank accession number WP_095206569.1). Blast analysis showed that tsOSHS belong to cystathionine synthase family and PLP-dependent enzyme superfamily which used PLP as the coenzyme for enzymatic reaction (Figure 2). The cystathionine synthase family can catalyze the α, β elimination and α, γ elimination. However, there is no corresponding report on the characteristics of OSHS in this reaction. Thirty sequences belonged to the cystathionine synthase were collected to analyze their phylogenetic relationship. The phylogenetic tree is divided into two obvious clusters, namely β elimination and γ elimination.
α, β-elimination mechanism is usually carried out through a quinone intermediate, in which the carbanion formed upon abstraction of the Cα-proton is stabilized by delocalization into the pyridine ring of the PLP (Aitken and Kirsch, 2005). This transient intermediate can be detected when the elimination of the group is slow. All enzymes of the transsulfuration and reverse transsulfuration pathway except cysteine β-synthase are members of this subfamily. The structures of E. coli cysthionine-γ-Synthase (eCGS), yeast cystathionine c-lyase (yCGL) may originated from O-acetyl-L-homoserine sulfhydrylase, which were similar that their active sites located at the interface of subunits, and are composed of residues from two subunits (Messerschmidt et al., 2003). Phylogenetic analysis showed that tsOSHS is a member of the PLP-dependent enzyme superfamily cysteine γ-synthase.
Gene Synthesis, Expression and Purification of OSHS
In order to functionally characterize the target enzyme, the synthesized tsOSHS gene was cloned into the pET28b expression vector. The 6 × His tag was added to the carbon end of the protein for affinity-based enzyme purification. The recombinant tsOSHS was purified from the supernatant of the cell lysate on a Ni-NTA column by one-step affinity chromatography. SDS-PAGE of the purified enzyme gave a single band showed a molecule of ~40 kDa (Supplementary Figure 1), which corresponded to the molecular weight calculated from the amino acid sequence obtained. The purified tsOSHS (318.2 U/mg) was then used for enzymatic characterization.
Effects of pH and Temperature on tsOSHS Activities
tsOSHS has high activity and stability in slight acid neutral buffer solution from pH 6.5 to 7.5, and the relative activity reached optimum at pH 6.5 (Figure 3), which indicated that tsOSHS has high activity in a certain pH range and has the potential of industrial application. However, the enzyme activity of tsOSHS decreased significantly when pH was higher than 7.5. In addition, the enzyme activity of tsOSHS in PBS was higher than that in other buffers. As shown in Figure 3, tsOSHS still maintained more than 80% activity in the pH range of 6.5–8.5 after 10 h incubation. The highest stability was achieved at pH 7.0 and the stability decreased significantly when pH was lower than 6.5, which indicated that the enzyme was unstable in acidic conditions. It could be found from the above results that the optimum pH and the pH maintain enzyme stability were more biased toward alkaline conditions. However, the enzyme activity reached the highest under neutral conditions. It could be explained that tsOSHS is derived from T. sulfidiphilus which is an alkaliphilic strain can maintain good stability in alkaline environment. In this study, tsOSHS showed better activity in neutral environment when it catalyzing OSH convert to L-methionine, as the substrate hydrolyzed spontaneously in alkaline environment which would cause that the detected enzyme activity lower than the actual enzyme activity.
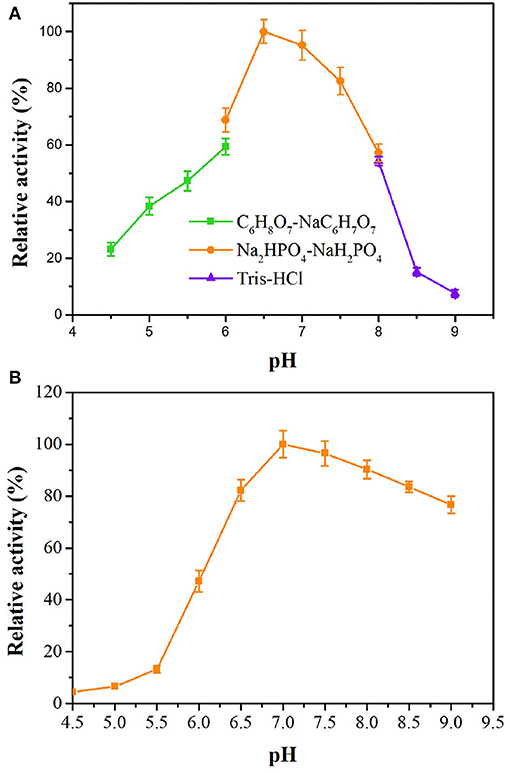
Figure 3. Effects of pH on enzyme activity of recombinant OSHS. The reactions were performed at 30°C for 10 min with 100 mM OSH and 5% (v/v) sodium methyl mercaptan. (A) pH optimization; (B) pH stability, the enzyme was incubated in buffers at pH 4.5–9.0 at 4°C for up to 10 h.
As shown in Figure 4, tsOSHS had high enzyme activity at 25–45°C. The optimal temperature was 35°C, and there was still 91% of the optimal enzyme activity at 40°C. When the temperature increased over 45°C, the enzyme activity decreased rapidly, and almost no enzyme activity was detected at 60°C. The residual enzyme activity of tsOSHS was detected after incubation at 25–45°C in PBS (pH6.5). Accordingly, the half-life (t1/2) values were determined to be 21.72, 18.68, 16.05, and 7.32 h, respectively (Figure 4). These results indicated that tsOSHS had an excellent thermostability at 30–40°C, which make it a potential candidate for biocatalytic process development.
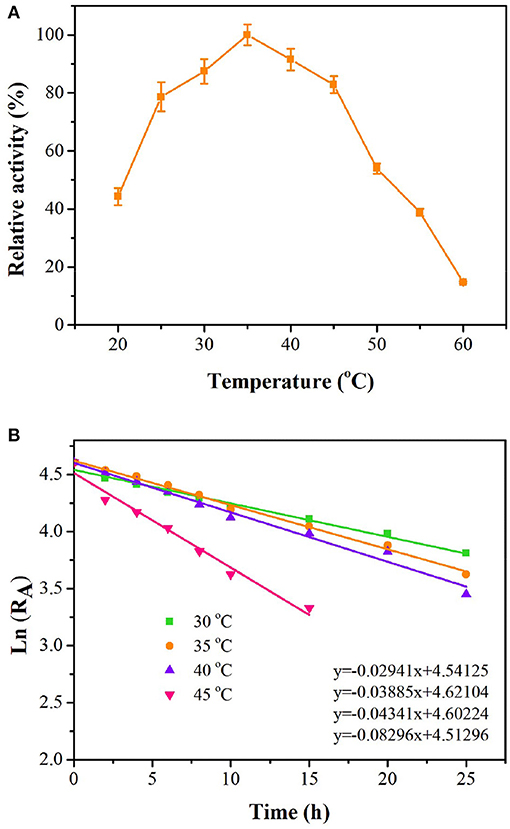
Figure 4. Effects of temperature on enzyme activity of recombinant OSHS. The reactions were performed at pH 7.0 for 10 min with 100 mM OSH and 5% (v/v) sodium methyl mercaptan. (A) Temperature optimization; (B) temperature stability, the enzyme was incubated in buffer at pH 7.0 at 20–60°C for up to 25 h.
Effects of Metal Ions and Organic Solvents
In order to explore whether tsOSHS has the properties of metalloenzymes, the effects of metal ions and organic solvents on enzyme activity were studied. The activity of purified tsOSHS affected by metal ions and organic solvents in the reaction were shown in Supplementary Figure 2. The activity of tsOSHS decreased with the increase of metal ion concentration. When the concentration of Cu2+ was 1 mM, the enzyme was seriously inhibited. When the concentration of Cu2+ and Al3+ was 5 mM, the enzyme was almost completely inhibited. Ni2+, Co2+, and Zn2+ also had a significant inhibitory effect on enzyme activity at 5 mM. In contrast, when the final concentration of Fe2+ was 1 mM, the activity increased 115%, but decreased when the concentration was 5 mM. And the effect of Fe2+ concentration on the relative activity of tsOSH was studied (Supplementary Figure 3). At the same time, the effects of 1 mM surfactant SDS and metal chelating agent EDTA on tsOSHS pre-treated with 1 mM Fe2+ were studied. The relative enzyme activity was 63 and 90%, respectively, which indicated that the enzyme activity showed inhibition under the reaction of organic solvents. Apparently, according to the above experiment results, various metal ions had almost no positive effect on tsOSHS, and the addition of metal chelating agents had no obvious inhibitory effect. Nowadays, there is no report showing that CGS has the characteristics of metalloenzymes. Therefore, it could be determined that tsOSHS is not a metalloenzyme.
Kinetic Parameters
As shown in Supplementary Figure 4, the kinetic parameters were calculated through non-linear fitting results by plotting the initial velocity and OSH concentration. It was found that the Vmax, Km, and kcat values of tsOSHS were 44.57 μmol min−1 mg−1, 72.52 mM and 29.71 s−1, respectively. It's higher than the Km and kcat of CGS from other sources producing cystathionine, such as 2.5 mM for A. thaliana CGS (Stephane et al., 1998), 3.02 mM for H. pylori CGS, and 1.29 mM for eCGS (Kong et al., 2008). It could be related to the structure of the sulfur donor. Compared with cysteine, methyl mercaptan used in this study leads to a smaller steric hindrance, so that the reaction of γ-elimination might be easier to happen.
Biosynthesis of L-methionine Using Recombinant Purified tsOSHS
The effects of substrate concentration, enzyme concentration and PLP concentration on L-methionine production were studied. The yield of L-methionine was increased with the increasing of OSH concentration, and 38.25 g/L of L-methionine was obtained at 400 mM OSH reaching maximum yield (Figure 5A). With the extension of reaction time, the accumulation of L-methionine gradually slowed down and stabilized. When the substrate concentration was 400 mM, the conversion rate of OSH was 64.25% at 240 min and L-methionine was accumulated slowly. The results showed that the 30 mg/L tsOSHS could not completely catalyze 400 mM OSH. Therefore, in the following experiment, the concentration of tsOSHS in 400 mM OSH was optimized. As shown in Figure 5B, when the enzyme concentration increased from 30 to 100 mg/L, the accumulation rate of L-methionine increased. After about 120 min, 42.3 g/L-methionine was obtained, and the conversion rate increased from 64.17 to 71%. In addition, PLP is the coenzyme of tsOSHS and an important rate-limiting factor, which could affect the catalytic activity of tsOSHS. As shown in Figure 5C, when the concentration of PLP was lower than 10 mM, the accumulation amount and accumulation speed of L-methionine varied with the PLP concentration, indicating that the coenzyme concentration was not enough to maintain the catalytic activity of tsOSHS at this concentration. Besides, PLP not only has a catalytic role but also participate in the stability of CGS (Stephane et al., 1998). Therefore, when the PLP concentration increased, the conversion rate was significantly increased. When the concentration of PLP was over 10 mM, the yield of L-methionine and accumulation speed was close and the highest yield of L-methionine was 42.63 g/L. This indicated that when the concentration of PLP reached 10 mM, the ratio of enzyme and coenzyme was suitable for catalyzing the L-methionine production, and continued to increase the coenzyme concentration has almost no effect on the catalytic activity of the enzyme.
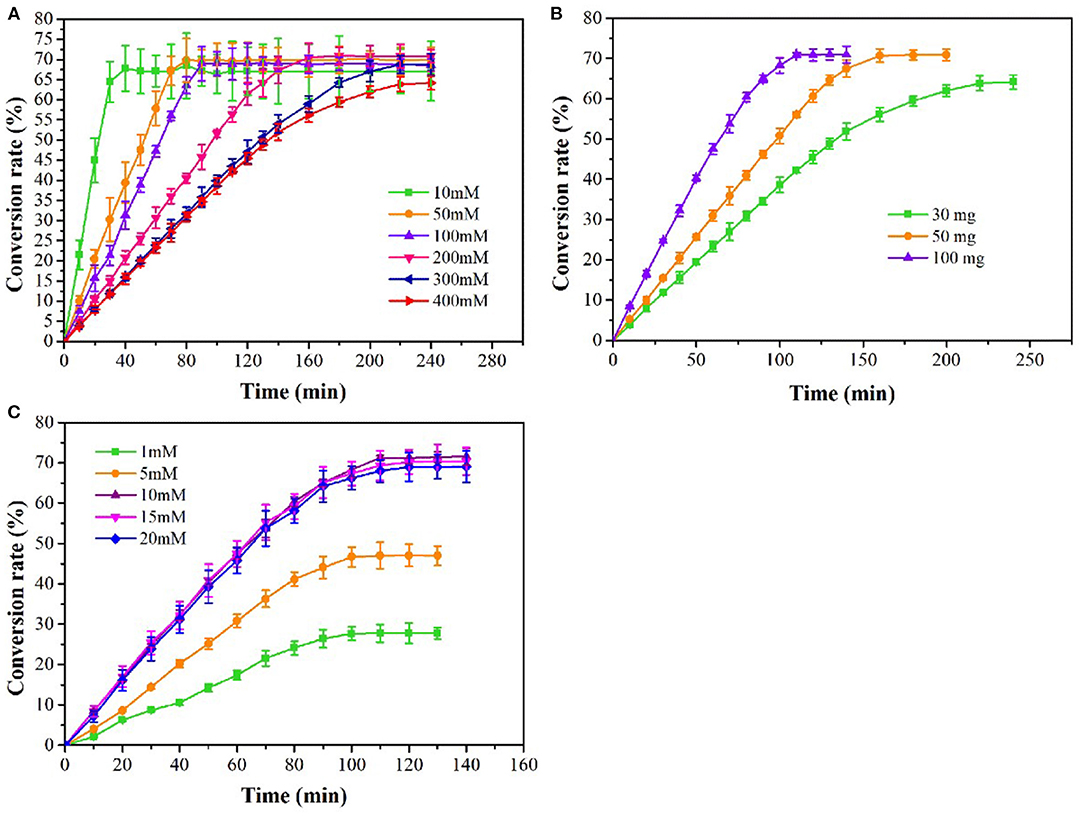
Figure 5. Effects on the production of L-methionine at different coditions. (A) The production of L-methionine at various substrate concentrations with 30 mg/L tsOSHS. The reactions were performed in the PBS (pH6.5) at 30°C with 10 mM PLP and 10% (v/v) sodium methyl mercaptan; (B) The production of L-methionine at various OSHS concentrations with 400 mM OSH. The reactions were performed in the PBS (pH 6.5) at 30°C with 10 mM PLP and 10% (v/v) sodium methyl mercaptan; (C) The production of L-methionine at various PLP concentrations with 400 mM OSH and 100 mg/L tsOSHS and 5% (v/v) sodium methyl mercaptan. The reactions were performed in the PBS (pH 6.5) at 30°C.
Conclusions
In this study, a cystathionine synthase family tsOSHS with the activity to catalyze OSH to L-methionine screened from NCBI by blast was synthesized and expressed in E. coli BL21 (DE3). The recombinant enzyme was purified by one-step affinity chromatography on Ni-NTA column. The properties of purified tsOSHS were studied. The recombinant tsOSHS reached highest activity at pH 6.5 and 35°C. It exhibited excellent thermostability with a half-life of 21.72 h at 30°C. Trace Fe2+ could promote the activity of tsOSHS, but the promotion is limited. Under the optimal conditions, the substrate concentration, enzyme amount and coenzyme amount were optimized, and finally 42.63 g/L L-methionine was obtained, and the conversion rate was 71%.
In conclusion, this is the first report on the synthesis, expression and enzymatic properties of OSHS which used in the L-methionine production and the high substrate tolerance, high conversion rate, high productivity (511.56 g/L/d) and high yield of L-methionine demonstrated the high potential of tsOSHS in large-scale biosynthesis of L-methionine.
Data Availability Statement
The original contributions presented in the study are included in the article/Supplementary Material, further inquiries can be directed to the corresponding author.
Author Contributions
Z-QL and Y-GZ initiated and supervised the project. W-YZ carried out all data analyses. W-YZ and KN wrote and modified the manuscript. PL and Y-HF conceived the study, participated in designing, and coordinating the study. All authors read and approved the final manuscript.
Funding
This research was supported by the National Natural Science Foundation of China (Nos. 31971342 and 31700095).
Conflict of Interest
The authors declare that the research was conducted in the absence of any commercial or financial relationships that could be construed as a potential conflict of interest.
Supplementary Material
The Supplementary Material for this article can be found online at: https://www.frontiersin.org/articles/10.3389/fchem.2021.672414/full#supplementary-material
References
Aitken, S. M., and Kirsch, J. F. (2005). The enzymology of cystathionine biosynthesis: strategies for the control of substrate and reaction specificity. Arch. Biochem. Biophys. 433, 166–175. doi: 10.1016/j.abb.2004.08.024
Braunstein, A. E., Goryachenkova, E. V., and Lac, N. D. (1969). Reactions catalysed by serine sulfhydrase from chicken liver. Biochim. Biophys. Acta. 171, 366–368. doi: 10.1016/0005-2744(69)90173-9
Brazeau, B., Change, J.-S., Cho, K. M., Cho, Y. W., DeSouza, M., Jessen, H. J., et al. (2013). Compositions and Methods of Producing Methionine. U.S. Patent No 9150893. Washington, DC: U.S. Patent and Trademark Office.
Brzovic, P., Holbrook, E. L., Greene, R. C., and Dunn, M. F. (1990). Reaction mechanism of Escherichia coli cystathionine gamma-synthase: direct evidence for a pyridoxamine derivative of vinylglyoxylate as a key intermediate in pyridoxal phosphate dependent gamma-elimination and gamma-replacement reactions. Biochemistry 29, 442–451. doi: 10.1021/bi00454a020
Christen, P., and Mehta, P. K. (2001). From cofactor to enzymes. The molecular evolution of pyridoxal-5′-phosphate-dependent enzymes. Chem. Rec. 1, 436–447. doi: 10.1002/tcr.10005
Eliot, A. C., and Kirsch, J. F. (2003). Avoiding the road less traveled: how the topology of enzyme–substrate complexes can dictate product selection. Acc. Chem. Res. 36, 757–765. doi: 10.1021/ar0202767
El-Naggar, E. A., Deraz, S. F., Soliman, H. M., El-Deeb, N. M., and El-Shweihy, N. M. (2017). Purification, characterization and amino acid content of cholesterol oxidase produced by Streptomyces aegyptia NEAE 102. BMC Biotechnol. 17:76. doi: 10.1186/s12866-017-0988-4
Franke, I., Resch, A., Dassler, T., Maier, T., and Bock, A. (2003). YfiK from Escherichia coli promotes export of O-acetylserine and cysteine. J. Bacteriol. 185, 1161–1166. doi: 10.1128/JB.185.4.1161-1166.2003
Holbrook, E., Greene, R., and Krueger, J. (1990). Purification and properties of cystathionine gamma-synthase from overproducing strains of Escherichia coli. Biochemistry 29, 435–442. doi: 10.1021/bi00454a019
Hong, K. K., Kim, J. H., Yoon, J. H., Park, H. M., Choi, S. J., Song, G. H., et al. (2014). O-Succinyl-L-homoserine-based C4-chemical production: succinic acid, homoserine lactone, gamma-butyrolactone, gamma-butyrolactone derivatives, and 1,4-butanediol. J. Ind. Microbiol. Biot. 41, 1517–1524. doi: 10.1007/s10295-014-1499-z
Huang, J. F., Liu, Z. Q., Jin, L. Q., Tang, X. L., Shen, Z. Y., Yin, H. H., et al. (2017). Metabolic engineering of Escherichia coli for microbial production of L-methionine. Biotechnol. Bioeng. 114, 843–851. doi: 10.1002/bit.26198
Jaworski, A. F., Lodha, P. H., Manders, A. L., and Aitken, S. M. (2012). Exploration of the active site of Escherichia coli cystathionine γ-synthase. Protein Sci. 21, 1662–1671. doi: 10.1002/pro.2135
Kaplan, M. M., and Flavin, M. (1966). Cystathionine γ-synthetase of salmonella structural properties of a new enzyme in bacterial methionine biosynthesis. J. Biol. Chem. 241, 5781–5789. doi: 10.1016/S0021-9258(18)96341-6
Kim, S.-Y., Choi, K.-M., Shin, Y.-U., Um, H.-W., Choi, K.-O., Chang, J.-S., et al. (2015). Microorganism Producing L-Methionine Precursor and Method of Producing L-Methionine and Organic Acid From the L-Methionine Precursor. U.S. Patent No 8426171. Washington, DC: U.S. Patent and Trademark Office.
Kong, Y., Wu, D., Bai, H., Han, C., Chen, J., Chen, L., Hu, L., Jiang, H., and Shen, X. (2008). Enzymatic characterization and inhibitor discovery of a new cystathionine {gamma}-synthase from Helicobacter pylori. J. Biochem. 143, 59–68. doi: 10.1093/jb/mvm194
Krämer, R. (2004). Production of amino acids: physiological and genetic approaches. Food Biotechnol. 18, 171–216. doi: 10.1081/FBT-200025664
Kraus, J. P., and Rosenberg, L. E. (1983). Cystathionine β-synthase from human liver: improved purification scheme and additional characterization of the enzyme in crude and pure form. Arch. Biochem. Biophys. 222, 44–52. doi: 10.1016/0003-9861(83)90500-3
Messerschmidt, A., Worbs, M., Steegborn, C., Wahl, M. C., Huber, R., Laber, B., et al. (2003). Determinants of enzymatic specificity in the cys-met-metabolism PLP-dependent enzymes family: crystal structure of cystathionine ⋎-lyase from yeast and intrafamiliar structure comparison. Biol. Chem. 384, 373–386. doi: 10.1515/BC.2003.043
Mudd, S. H., Finkelstein, J. D., Irreverre, F., and Laster, L. (1965). Transsulfuration in mammals. Microassays and tissue distributions of three enzymes of the pathway. J. Biol. Chem. 240, 4382–4392. doi: 10.1016/S0021-9258(18)97073-0
Schonberg, A., and Moubacher, R. (1952). The strecker degardation of α-amino acid. Chem. Rev. 50, 261–277. doi: 10.1021/cr60156a002
Stephane, R., Bertrand, G., Dominique, J., and Roland, D. (1998). Cystathionine γ-synthase from Arabidopsis thaliana: purification and biochemical characterization of the recombinant enzyme overexpressed in Escherichia coli. Biochem. J. 331, 639–648. doi: 10.1042/bj3310639
Tang, X. L., Chen, L. J., Du, X. Y., Zhang, B., Liu, Z. Q., and Zheng, Y. G. (2020). Regulation of homoserine O-succinyltransferase for efficient production of L-methionine in engineered Escherichia coli. J. Biotechnol. 309, 53–58. doi: 10.1016/j.jbiotec.2019.12.018
Tokuyama, S., and Hatano, K. (1996). Overexpression of the gene for N-acylamino acid racemase from Amycolatopsis sp. TS-1-60 in Escherichia coli and continuous production of optically active methionine by a bioreactor. Appl. Microbiol. Biotechnol. 44, 774–777. doi: 10.1007/BF00178617
Tudball, N., and Reed, M. A. (1975). Purification and properties of cystathionine synthase from human liver. Biochem. Biophys. Res. Commun. 67, 550–555. doi: 10.1016/0006-291X(75)90847-5
Willke, T. (2014). Methionine production–a critical review. Appl. Microbiol. Biotechnol. 98, 9893–9914. doi: 10.1007/s00253-014-6156-y
Yamada, H., Takahashi, S., Sumino, K., Fukumitsu, H., and Yoneda, K. (1979). Process for Preparing L-Methionine. U.S. Patent No 4148688. Washington, DC: U.S. Patent and Trademark Office.
Zhu, W. Y., Niu, K., Liu, P., Fan, Y. H., Liu, Z. Q., and Zheng, Y. G. (2020). Enhanced O-succinyl-l-homoserine production by recombinant Escherichia coli DeltaIJBB*TrcmetL/pTrc-metA(fbr) -Trc-thrA(fbr) -yjeH via multilevel fermentation optimization. J. Appl. Microbiol. doi: 10.1111/jam.14884. [Epub ahead of print].
Keywords: O-succinyl-L-homoserine sulfhydrylase, cysthionine γ-synthase, L-methionine, biosyntheis, characterization
Citation: Zhu W-Y, Niu K, Liu P, Fan Y-H, Liu Z-Q and Zheng Y-G (2021) Identification and Characterization of an O-Succinyl-L-Homoserine Sulfhydrylase From Thioalkalivibrio sulfidiphilus. Front. Chem. 9:672414. doi: 10.3389/fchem.2021.672414
Received: 25 February 2021; Accepted: 23 March 2021;
Published: 14 April 2021.
Edited by:
Gao-Wei Zheng, East China University of Science and Technology, ChinaReviewed by:
Jun Huang, Zhejiang University of Science and Technology, ChinaYunpeng Bai, East China University of Science and Technology, China
Zhiming Rao, Jiangnan University, China
Copyright © 2021 Zhu, Niu, Liu, Fan, Liu and Zheng. This is an open-access article distributed under the terms of the Creative Commons Attribution License (CC BY). The use, distribution or reproduction in other forums is permitted, provided the original author(s) and the copyright owner(s) are credited and that the original publication in this journal is cited, in accordance with accepted academic practice. No use, distribution or reproduction is permitted which does not comply with these terms.
*Correspondence: Zhi-Qiang Liu, bWljcm9saXVAemp1dC5lZHUuY24=