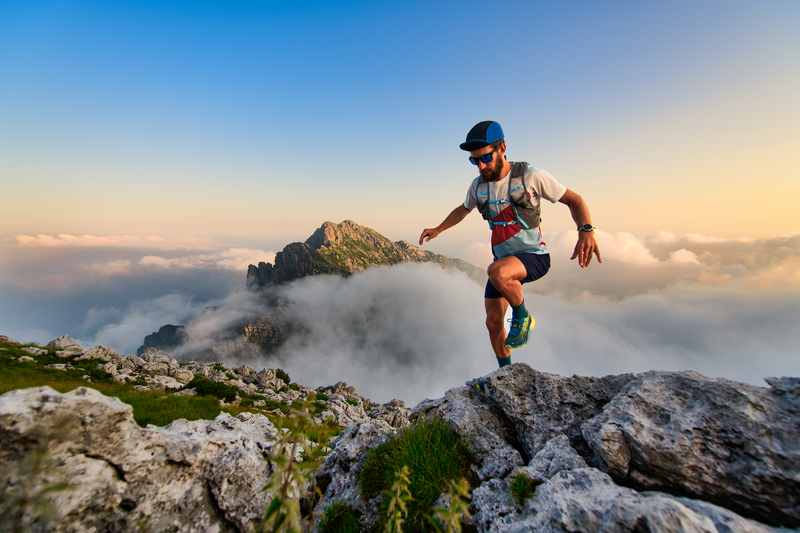
94% of researchers rate our articles as excellent or good
Learn more about the work of our research integrity team to safeguard the quality of each article we publish.
Find out more
MINI REVIEW article
Front. Chem. , 14 May 2021
Sec. Green and Sustainable Chemistry
Volume 9 - 2021 | https://doi.org/10.3389/fchem.2021.671750
This article is part of the Research Topic Frontiers in Chemistry - Rising Stars: Asia View all 13 articles
In the wake of plastic pollution increasing around the world, biodegradable plastics are one of the fastest-growing segments within the global plastics market. The biodegradation of these plastics depends on diverse factors including, but not limited to, the physicochemical structure of the materials, environmental conditions, and the microbial populations involved in the biodegradation. Although laboratory-based biodegradation tests simulate natural processes, they cannot precisely mimic the natural biodegradation of biodegradable plastics due to the disparity of several factors. In addition, the biodegradation levels claimed and/or reported by individuals and studies in different environments vary to a great extent. Biodegradable plastics are considered a sustainable alternative to non-biodegradable conventional plastics and are being promoted as an eco-friendlier choice for consumers. However, biodegradable plastics might not be as biodegradable as commonly believed, particularly in natural environments. This mini-review aims to bridge the following three gaps in biodegradable plastics by elucidating the common misconceptions and truths about biodegradation: i) the gaps among reported biodegradation level of biodegradable plastics; ii) the gaps between the biodegradation conditions in the controlled laboratory system and in the natural environment; and iii) the gaps between public perception and the actual environmental fate of biodegradable products. These gaps are critically reviewed with feasible solutions. This work will ease the assessment of biodegradable plastics and provide sound communication on corresponding claims–a prerequisite for successful market performance.
The massive accumulation of plastics in the natural environment is threatening the sustainability of our planet (Jambeck et al., 2015; UN Environment, 2018). As of 2014, over 250,000 tons of plastics were estimated to be floating in the sea (Eriksen et al., 2014). It is predicted that by 2030, 90 Mt/year of plastics will enter the aquatic ecosystem under the scenario where the current plastic production trend continues without improvements in the waste management system (Borrelle et al., 2020). Bioplastics refer to synthetic polymers that are biodegradable [e.g., poly(lactic acid) (PLA)] and/or are derived from bio-based materials [e.g., bio-based poly(ethylene) (bio-PE)]. Biodegradable plastics are one of the fastest-growing segments within the global plastics market. The global production capacity for biodegradable plastics was 1.2 Mt/year in 2020 and is expected to grow rapidly (European Bioplastics, 2020).
Numerous standards have paved the way for evaluating the biodegradability of plastic by simulating various environments, including natural conditions such as soil and aquatic environments, and systemically controlled conditions such as industrial composting and anaerobic digestion. Studies on the biodegradability of biodegradable plastics have been conducted according to the most prominent standards such as International Organization for Standardization (ISO), American Society for Testing and Material (ASTM), and European Norm (EN) (Eubeler et al., 2009). Although laboratory-based biodegradation tests simulate natural processes, they cannot precisely mimic the natural biodegradation of biodegradable plastics due to the disparity of several factors. In addition, the biodegradation levels claimed and/or reported by individuals and studies in different environments vary to a great extent.
Despite of legislative efforts for the standardization and specification of biodegradable plastics, the complicated descriptions and coverage of bioplastics and biodegradable plastics (e.g., bio-based, biodegradable, compostable, oxo-biodegradable plastic, etc.) are confusing to the public. The word “bioplastic” is commonly perceived as a synonym for “biodegradable plastic” (Dilkes-Hoffman et al., 2019). In addition, a common perception of biodegradable plastics is that the materials will biodegrade in natural environments. The reality is that many “so-called” biodegradable plastics are not biodegradable in the aqueous environments (Bagheri et al., 2017; Emadian et al., 2017).
Most countries label the products decomposed under controlled composting test within the designated period. Some examples of these test standards are ASTM D5338 in the United States, EN 13432 in European Union, KS M ISO14855 in Korea, and JIS K 6953 in Japan. The test-passed products are labeled as “compostable” in many countries, whereas “eco-labeled” and “biodegradable” labels are inappropriately applied in Korea and Japan (Supplementary Table S1). This can lead to overestimation of biodegradability of the labeled products.
This mini-review aims to bridge the following three gaps in biodegradable plastics by elucidating the common misconceptions and truths about biodegradation: i) the gaps among reported biodegradation level of biodegradable plastics; ii) the gaps between the biodegradation conditions in the controlled laboratory system and in the natural environment; and iii) the gaps between public perception and the actual environmental fate of biodegradable products. These gaps are critically reviewed with feasible solutions. This work will ease the assessment of biodegradable plastics and provide sound communication on corresponding claims–a prerequisite for successful market performance.
The first step to bridge the gaps is to understand the biodegradation of biodegradable plastics–How then do these materials decompose in the natural environments? Biodegradation is a biological process, whereby polymers are partially or completely converted into the end products by the action of microorganisms (Song et al., 2009; Luckachan and Pillai, 2011; Soroudi and Jakubowicz, 2013). Biodegradable plastics are decomposed via four stages: biodeterioration, depolymerization, assimilation, and mineralization (Harrison et al., 2018). First, the microbial biofilms are formed on the surface of the materials, and decomposers and/or abiotic factors fragment them into tiny fractions, while polymers lose their initial physicochemical properties. Second, from the biofilm, the extracellular enzymes are excreted. Numerous enzymes specifically depolymerize polymers into smaller units such as oligomers, dimers, and monomers, reducing the molecular weight. Third, these molecules are metabolically assimilated in the cytoplasm, producing new biomass and energy as well as primary and secondary metabolites. Eventually, these metabolites are mineralized into the end products such as carbon dioxide, methane, water, and mineral salts (Lucas et al., 2008).
The biodegradation of biodegradable plastics depends on diverse factors including, but not limited to, the physicochemical structure of the materials, environmental conditions, and the microbial populations involved in the biodegradation (Massardier-Nageotte et al., 2006). In the natural ecosystem, biotic and abiotic factors synergistically decompose biodegradable plastics. Biotic factors are plastic-decomposing microorganisms such as bacteria, fungi, archaea, and algae (Lee et al., 2005; Kyrikou and Briassoulis, 2007; Gonzalez-Fernandez et al., 2015). Some examples of abiotic factors include temperature, sunlight, mechanical impact (weathering), oxygen, humidity, and acidity (Song et al., 2009; Thakur et al., 2018). Abiotic hydrolysis is the main degradation step, as humidity and temperature enable cleavage of the ester linkage (Karamanlioglu et al., 2017). The rate of hydrolysis and biodeterioration increases when the temperature exceeds the glass temperature of the polymer (Henton et al., 2005). The presence of oxygen determines the type of decomposers and biological reaction. When oxygen is available, aerobic organisms utilize the polymers as carbon and energy sources (Sudesh and Iwata, 2008). In anaerobic conditions, anaerobic organisms decompose polymers and generate biogas, mainly in the form of methane (Tokiwa et al., 2009; Mekonnen et al., 2013; Bátori et al., 2018).
It should be underlined that degradation and biodegradation are different (Shah et al., 2008; Luckachan and Pillai, 2011). Degradation of non-biodegradable plastics terminates at the fragmentation stage, generating even more persistent microplastics (Wagner et al., 2014; Auta et al., 2017; Mason et al., 2018). In contrast, biodegradation involves further biological steps, ultimately mineralizing the polymers. We suggest that the definition of biodegradable plastics be established based on their capacity to be mineralized into gaseous end products when the surrounding condition meets biodegradability requirements such as temperature, moisture, and microbial populations.
We collected the results of studies that quantitatively measured biodegradation level via weight loss and/or produced gaseous end products (Figure 1). Despite some variations among studies, we analyzed the biodegradation data to obtain critical insights into biodegradable plastics.
FIGURE 1. Biodegradation study results of biodegradable plastics in the laboratory (blue) and in the natural environments (green), selected by the authors. The comprehensive biodegradation data are available in the supplementary material (Supplementary Table S2). Note that the analytical analysis (averaging) was conducted based on the supplementary data set.
The bio-based and biodegradable poly(hydroxyalkanoates) (PHA) and PLA are most widely studied. PHA-based bioplastics are biodegradable in all indicated environments (Volova et al., 2007; Woolnough et al., 2008; Yagi et al., 2014). PLA-based bioplastics, on the other hand, are well biodegradable under industrial composting and anaerobic digesting conditions, but are hardly biodegradable in soil and aquatic environments (Pranamuda et al., 1997; Itävaara et al., 2002; Tsuji and Suzuyoshi, 2002a; Tsuji and Suzuyoshi, 2002b; Shogren et al., 2003; Martin et al., 2014). PLA requires specific high-temperature conditions and degrades through abiotic hydrolysis (Elsawy et al., 2017; Gorrasi and Pantani, 2017). On the contrary, poly(lactide-co-glycolide) (PLGA), a PLA-based copolymer, was completely biodegraded in seawater and freshwater within 270 days (Bagheri et al., 2017).
The type of environment is a significant determinant of biodegradation. Each environment has different conditions such as temperature, humidity, and microbial populations (Kale et al., 2007; Tokiwa et al., 2009). Based on comprehensive data analysis (Supplementary Table S2), we show the manifest difference in biodegradation in various environments. The average biodegradation level can be arranged in descending order as follows: industrial composting (72.3% over 75 days), anaerobic digestion (64.6% over 88 days), marine (47.1% over 155 days), soil (39.7% over 159 days), and aerobic aqueous (31.7 over 113 days) environments. Industrial composting is a highly optimized system for the biodegradation by thermophilic microorganisms (Gómez and Michel, 2013; Arrieta et al., 2014). Due to the high temperature (typically 55–60°C, Mathur, 1998) and proper water content (50–60% is appropriate for most materials, Mathur, 1998), the highest biodegradation level and shortest period are achieved under industrial composting conditions.
The microbial populations and the fraction of decomposers in the microbial community significantly differ depending on the environment. Microorganisms generally thrive where the environmental conditions suffice. Industrial composting and soil environments contain more microbes per unit than aquatic environments (Watson et al., 1977; Flemming and Wuertz, 2019; Wang et al., 2020). Even if the same material is tested under the same type of environment, the biodegradation level can vary to a great extent. For example, in one study (Boyandin et al., 2013), the weight of poly(3-hydroxybutyrate) (PHB) films buried in natural soil was reduced by 98 and 47% near Hanoi and Nha Trang, Vietnam, respectively. The higher biodegradation in the Hanoi area was attributed to richer PHA degrading microbial populations in the soil.
Although biodegradation cannot be measured via weight loss, it is widely applied in degradation tests (Shah et al., 2008). Weight loss can incorporate the influence of biodegradation, abiotic hydrolysis, and production of water-soluble products (e.g., plasticizers). In one study (Abou-Zeid et al., 2001), the weight of poly(3-hydroxybutyrate-co-3-hydroxyvalerate) (PHBV) was reduced by 60%, but only 29% of the theoretical biogas was formed. Measuring the gaseous end product by a respirometry system determines the biodegradation level precisely, although some carbons are assimilated into the new biomass (Shah et al., 2008). The biomass should be accounted for in the carbon balance during biodegradation. However, no analytical methods are available until now (Degli Innocenti and Breton, 2020). Therefore, weight loss should be applied as a biodegradation indicator only when the condition meets biodegradability requirements.
In sum, due to numerous biotic and abiotic factors being complicatedly involved in the biodegradation process, discrepancies among reported biodegradation level are inevitably present, and are difficult to standardize.
Biodegradability studies have been mainly conducted under laboratory systems based on test standards by simulating various biodegradation environments. However, biodegradation is highly accelerated in designed laboratory systems where the condition meets all biodegradability requirements. It is important to understand that there is a discrepancy between biodegradation conditions in the laboratory and in the natural environments (Figure 2A).
FIGURE 2. (A) Disparity in biodegradation conditions in the laboratory and in the natural environments. (B) The discrepancy between public perception and the actual biodegradability of labeled products. An industrial composting test is commonly applied for the certification, but the conditions in industrial composting facilities hardly exist in natural environments.
Temperature is a significant abiotic factor that influences microbial activity (Shen and Burgess, 2012; Pischedda et al., 2019), thermal degradation, and aging (Deroiné et al., 2014). Biodegradable plastics experience fluctuating temperature in nature, whereas they remain under stable temperature in a laboratory system (Rudnik and Briassoulis, 2011). For instance, in temperate regions, seasonal temperature fluctuation accelerates or delays biodegradation. Biodegradation research conducted under both conditions showed such disparity: 30°C in laboratory incubation (ASTM D6691) compared to 12–22°C in aquarium incubation subjected to continuously flowing seawater (Thellen et al., 2008).
Access to sunlight accelerates the decomposition of polymers (photodegradation). Most plastics tend to absorb high-energy radiation in the ultraviolet (UV) portion of the spectrum, which can activate their electrons to higher reactivity and cause oxidation, cleavage, and other degradation (Shah et al., 2008; Sadi et al., 2010). UV radiation can disrupt polymeric chains and embrittle the polymers by being absorbed in oxygen-containing components (Lucas et al., 2008; Rujnić-Sokele and Pilipović, 2017). Several ISO standards suggest that the samples be located under a dark or diffused light incubator. However, artificial light (i.e., a light bulb) in a laboratory system generally does not generate UV radiation. This contrasts with the conditions in a natural environment with intermittent (e.g., floating and sinking in the ocean) or long-term exposure to sunlight.
Mechanical stress is a significant abiotic parameter that affects the degradation of plastics. Weathering degradation of plastics results in their surface embrittlement and microcracking (Andrady, 2011). The free surface energy increased after weathering of PLA-lignin bioplastics (Spiridon et al., 2015). In addition, continuously pumped seawater may increase the time for microorganisms to colonize surfaces (Deroiné et al., 2014; Wright et al., 2020). Laboratory systems mimic these natural mechanical stresses by agitating incubators. However, agitation during the laboratory experiment showed little influence on biodegradation level (Briese et al., 1994).
The shape of materials considerably affects biodegradation rate (Volova et al., 2010; Boyandin et al., 2013). In the laboratory test, the samples are generally prepared in powder, film, or pellet forms for the assessment of their biodegradability. This maximizes surface area and facilitates the biodeterioration stage by providing more surface area for microorganisms to colonize, and therefore accelerates biodegradation rate (Andrady, 1994; Chinaglia et al., 2018). However, in most cases, littered products are decomposed from their original shapes, and disintegration occurs via multiple factors like weathering, UV radiation, microbial activity.
The presence of co-substrates such as food or beverage residue influences microbial activity (Andrady, 1994), as much of the plastic waste found in the environment consists of food-related packaging (Marsh and Bugusu, 2007; Heidbreder et al., 2019). These substrates shorten the lag phase of biodegradation by accelerating biofilm formation. However, in laboratory experiments, pure polymers are commonly tested. In this context, blending organic materials with biodegradable plastics influences the biodegradation rate. Biodegradation was accelerated when biodegradable plastics were blended with various materials, such as corn (Sarasa et al., 2009), poultry feather fibers (Ahn et al., 2011), rice husk (Wu, 2014), potato peel waste (Wei et al., 2015), and empty fruit bunch fiber (Wei et al., 2015).
The microbial populations differ depending on the environment. The heterogeneity of environmental microorganisms (inoculum) leads to inconsistent biodegradability test results (Haider et al., 2019). The laboratory settings are designed to highly condense or isolate decomposers since they utilize polymers as the sole carbon and energy sources (Dussud et al., 2018), thereby accelerating biodegradation. In the natural environment, however, competition within the environmental microbiome takes place due to the presence of various carbon sources and the heterogeneity of the microbial populations (Andrady, 1994).
In sum, due to the disparity of key factors, biodegradation tests under laboratory systems do not sufficiently reflect natural conditions and this can lead to overestimation of the biodegradation level of biodegradable plastics in the event of littering. Therefore, biodegradation test standards should clearly indicate the limit of representativeness and/or new specific standards should provide more options to minimize the gaps to transfer natural biodegradation to the laboratory. For instance, the temperature range should be altered depending on the geographical region. Access to sunlight and water should also be considered to imitate synergistic reactions of biotic and abiotic factors.
The general knowledge of bioplastics is low, but perception, particularly of biodegradable plastics, is positive (Lynch et al., 2017; Dilkes-Hoffman et al., 2019). Choosing biodegradable products could be adopted as a possible solution to plastic pollution rather than reducing plastic consumption (Klein et al., 2019). Marketers are keen to tout the biodegradability of materials. However, a biodegradable label on products often leads to littering wastes with the belief that biodegradable plastics will decompose naturally (Klöckner, 2013; UNEP, 2015). The truth is that they might not be as biodegradable as commonly believed (Figure 2B).
The overestimation of the biodegradability of biodegradable plastics derives from three factors: i) reported biodegradability resulted from an optimized laboratory system; ii) the overlapping term between biodegradable and compostable plastics; and iii) a lack of discrete recycling codes and treatment systems.
First, biodegradability has been tested under laboratory systems and the results are valid. However, as examined earlier, biodegradation occurs only when surrounding condition suffices biodegradability requirements. In other words, a biodegradable plastic under laboratory test may not biodegrade in natural condition. Second, many countries clearly distinguish between compostable and biodegradable products via labeling system (Supplementary Table S1). However, as compostable plastics are often coined as biodegradable polymers (Haider et al., 2019), the public easily misunderstand the biodegradability of compostable products. In addition, few are aware that compostable and biodegradable plastics are different, and the conditions in industrial composting facilities hardly exist in natural environments. Finally, recycling code (resin identification code, in some countries) on biodegradable plastics is “7”, which indicates “other polymers”. As a result, they are hardly recycled, and in turn are incinerated, landfilled or littered (Pathak et al., 2014). Incinerating is not a desirable mode for fossil-based biodegradable plastics, as it consumes a high amount of energy and emits greenhouse gases (Razza et al., 2015; Folino et al., 2020a). Also, some biodegradable products may not have completed their life cycle when landfilled (Shin et al., 1997; Quecholac-Piña et al., 2020).
PLA provides a good illustration to explain these misconceptions. PLA is derived from bio-based resources and is applied to daily commodities such as disposable packaging and cups (Karamanlioglu et al., 2017). As PLA is promoted as an eco-friendly material, the public may assume that it is naturally decomposed in the ocean. However, PLA is well biodegradable only under industrial composting and anaerobic digesting conditions (Supplementary Table S2). Even if products are labeled compostable (as in most Western countries), many people might be confused between compostable and biodegradable plastics. Furthermore, the recycling code on PLA products is generally “7”, which may lead most littered PLA products to not being properly treated.
Therefore, we suggest that a separate recycling code for compostable plastics be established to ensure that these plastics end up their life cycle in an industrial composting facility, and littering should be the last resort. Furthermore, following the growing production trend of biodegradable plastics, separate collection systems and treatment facilities should be built, making so-called biodegradable products genuinely biodegradable. Education on biodegradable plastics should be provided so that the public can make informed decisions (UNEP, 2015). For instance, it should be understood that industrial composting test does not necessarily guarantee biodegradation in natural conditions, especially in aquatic environments. The labeling on compostable products should clearly indicate that they are only biodegradable in an industrial composting facility. Society should have access to reliable, authoritative, and clear guidance on what terms such as “compostable” or “biodegradable” actually mean. The national legalization on the i) definition, ii) classification, iii) labeling, iv) collection system, and v) treatment guideline will enhance public awareness of biodegradable plastics and eliminate misconceptions.
Biodegradable polymers are only beneficial when they can actually biodegrade (Gross and Kalra, 2002). Bridging the aforementioned three gaps will enhance sound communication on biodegradable plastics, eliminating confusion and misconceptions. Understanding the truths about biodegradable plastics will provide support for the progressive substitution of conventional plastics with biodegradable plastics. There are also other anticipated outcomes: i) Products with high biodegradability will be promoted in the market. ii) Policies on eco-friendlier and sound design will be established as well as financial incentives. iii) The development of biodegradation accelerating technology will be triggered. iv) Waste littering based on the false belief in the biodegradability of biodegradable plastics will be minimized. v) Bridging the gaps in biodegradable plastics will open up a sustainable future.
SC wrote the manuscript and designed figures. YK collected and analyzed biodegradation research and wrote part of the manuscript. YW investigated biodegradation certification and edited the figures. JM supervised the overall progress of the research.
This work was supported by the National Research Foundation of Korea (NRF) grant funded by the Korea government (MSIT) (No. 2020R1F1A1076022), and research grants from the Korea Advanced Institute of Science and Technology (KAIST) and KAIST’s Corona Response Science and Technology New Deal Project (MCM-2020-N11200211). Also, this research was a part of the project titled “Development of 1 tonf-class Ultrasonic Pretreatment-Pyrolysis Liquefaction Facility and Operating System for Marine Plastic Recycling,” funded by the Ministry of Oceans and Fisheries, Korea, and the “Innovative Talent Education Program for Smart City,” funded by Korea Ministry of Land, Infrastructure and Transport (MOLIT).
The authors declare that the research was conducted in the absence of any commercial or financial relationships that could be construed as a potential conflict of interest.
The Supplementary Material for this article can be found online at: https://www.frontiersin.org/articles/10.3389/fchem.2021.671750/full#supplementary-material
Abou-Zeid, D.-M., Müller, R.-J., and Deckwer, W.-D. (2001). Degradation of Natural and Synthetic Polyesters under Anaerobic Conditions. J. Biotechnol. 86 (2), 113–126. doi:10.1016/s0168-1656(00)00406-5
Ahn, H. K., Huda, M. S., Smith, M. C., Mulbry, W., Schmidt, W. F., and Reeves, J. B. (2011). Biodegradability of Injection Molded Bioplastic Pots Containing Polylactic Acid and Poultry Feather Fiber. Bioresour. Tech. 102 (7), 4930–4933. doi:10.1016/j.biortech.2011.01.042
Andrady, A. L. (1994). Assessment of Environmental Biodegradation of Synthetic Polymers. J. Macromolecular Sci. C: Polym. Rev. 34 (1), 25–76. doi:10.1080/15321799408009632
Andrady, A. L. (2011). Microplastics in the Marine Environment. Mar. Pollut. Bull. 62 (8), 1596–1605. doi:10.1016/j.marpolbul.2011.05.030
Arrieta, M. P., López, J., Rayón, E., and Jiménez, A. (2014). Disintegrability under Composting Conditions of Plasticized PLA-PHB Blends. Polym. Degrad. Stab. 108, 307–318. doi:10.1016/j.polymdegradstab.2014.01.034
Auta, H. S., Emenike, C. U., and Fauziah, S. H. (2017). Distribution and Importance of Microplastics in the Marine Environment: a Review of the Sources, Fate, Effects, and Potential Solutions. Environ. Int. 102, 165–176. doi:10.1016/j.envint.2017.02.013
Bagheri, A. R., Laforsch, C., Greiner, A., and Agarwal, S. (2017). Fate of So-Called Biodegradable Polymers in Seawater and Freshwater. Glob. Challenges 1 (4), 1700048. doi:10.1002/gch2.201700048
Bátori, V., Åkesson, D., Zamani, A., Taherzadeh, M. J., and Sárvári Horváth, I. (2018). Anaerobic Degradation of Bioplastics: A Review. Waste Manage. 80, 406–413. doi:10.1016/j.wasman.2018.09.040
Borrelle, S. B., Ringma, J., Law, K. L., Monnahan, C. C., Lebreton, L., McGivern, A., et al. (2020). Predicted Growth in Plastic Waste Exceeds Efforts to Mitigate Plastic Pollution. Science 369 (6510), 1515–1518. doi:10.1126/science.aba3656
Boyandin, A. N., Prudnikova, S. V., Karpov, V. A., Ivonin, V. N., Đỗ, N. L., Nguyễn, T. H., et al. (2013). Microbial Degradation of Polyhydroxyalkanoates in Tropical Soils. Int. Biodeterioration Biodegradation 83, 77–84. doi:10.1016/j.ibiod.2013.04.014
Briese, B. H., Jendrossek, D., and Schlegel, H. G. (1994). Degradation of Poly(3-Hydroxybutyrate-Co-3-Hydroxyvalerate) by Aerobic Sewage Sludge. FEMS Microbiol. Lett. 117 (1), 107–111. doi:10.1111/j.1574-6968.1994.tb06750.x
Chinaglia, S., Tosin, M., and Degli‐Innocenti, F. (2018). Biodegradation Rate of Biodegradable Plastics at Molecular Level. Polym Degrad and Stabil 147, 237–244.
Folino, A., Calabro', P. S., Fazzino, F., and Komilis, D. (2020a). Preliminary Evaluation of the Anaerobic Biodegradability of Three Biobased Materials Used for the Production of Disposable Plastics. J. Hazard. Mater. 390, 121653. doi:10.1016/j.jhazmat.2019.121653
Degli Innocenti, F., and Breton, T. (2020). Intrinsic Biodegradability of Plastics and Ecological Risk in the Case of Leakage. ACS Sustain. Chem. Eng. 8 (25), 9239–9249. doi:10.1021/acssuschemeng.0c01230
Deroiné, M., Le Duigou, A., Corre, Y.-M., Le Gac, P.-Y., Davies, P., César, G., et al. (2014). Seawater Accelerated Ageing of Poly(3-Hydroxybutyrate-Co-3-Hydroxyvalerate). Polym. Degrad. Stab. 105, 237–247. doi:10.1016/j.polymdegradstab.2014.04.026
Dilkes-Hoffman, L., Ashworth, P., Laycock, B., Pratt, S., and Lant, P. (2019). Public Attitudes towards Bioplastics - Knowledge, Perception and End-Of-Life Management. Resour. Conservation Recycling 151, 104479. doi:10.1016/j.resconrec.2019.104479
Dussud, C., Hudec, C., George, M., Fabre, P., Higgs, P., Bruzaud, S., et al. (2018). Colonization of Non-biodegradable and Biodegradable Plastics by Marine Microorganisms. Front. Microbiol. 9, 1571. doi:10.3389/fmicb.2018.01571
Elsawy, M. A., Kim, K.-H., Park, J.-W., and Deep, A. (2017). Hydrolytic Degradation of Polylactic Acid (PLA) and its Composites. Renew. Sustain. Energ. Rev. 79, 1346–1352. doi:10.1016/j.rser.2017.05.143
Emadian, S. M., Onay, T. T., and Demirel, B. (2017). Biodegradation of Bioplastics in Natural Environments. Waste Manage. 59, 526–536. doi:10.1016/j.wasman.2016.10.006
Eriksen, M., Lebreton, L. C., Carson, H. S., Thiel, M., Moore, C. J., Borerro, J. C., et al. (2014). Plastic Pollution in the World's Oceans: More Than 5 Trillion Plastic Pieces Weighing over 250,000 Tons Afloat at Sea. PLOS one 9 (12), e111913. doi:10.1371/journal.pone.0111913
Eubeler, J. P., Zok, S., Bernhard, M., and Knepper, T. P. (2009). Environmental Biodegradation of Synthetic Polymers I. Test Methodologies and Procedures. Trac Trends Anal. Chem. 28 (9), 1057–1072. doi:10.1016/j.trac.2009.06.007
European Bioplastics (2020). Bioplastics Market Development Update 2020. Berlin: European Bioplastics.
Flemming, H.-C., and Wuertz, S. (2019). Bacteria and Archaea on Earth and Their Abundance in Biofilms. Nat. Rev. Microbiol. 17 (4), 247–260. doi:10.1038/s41579-019-0158-9
Gómez, E. F., and Michel, F. C. (2013). Biodegradability of Conventional and Bio-Based Plastics and Natural Fiber Composites during Composting, Anaerobic Digestion and Long-Term Soil Incubation. Polym. Degrad. Stab. 98 (12), 2583–2591. doi:10.1016/j.polymdegradstab.2013.09.018
Gonzalez-Fernandez, C., Sialve, B., and Molinuevo-Salces, B. (2015). Anaerobic Digestion of Microalgal Biomass: Challenges, Opportunities and Research Needs. Bioresour. Tech. 198, 896–906. doi:10.1016/j.biortech.2015.09.095
Gorrasi, G., and Pantani, R. (2017). Hydrolysis and Biodegradation of Poly (Lactic Acid). Synthesis, Structure and Properties of Poly (Lactic Acid). (Boston, MA: Springer), 119–151. doi:10.1007/12_2016_12
Gross, R. A., and Kalra, B. (2002). Biodegradable Polymers for the Environment. Science 297 (5582), 803–807. doi:10.1126/science.297.5582.803
Haider, T. P., Völker, C., Kramm, J., Landfester, K., and Wurm, F. R. (2019). Plastics of the Future? the Impact of Biodegradable Polymers on the Environment and on Society. Angew. Chem. Int. Ed. 58 (1), 50–62. doi:10.1002/anie.201805766
Harrison, J. P., Boardman, C., O'Callaghan, K., Delort, A.-M., and Song, J. (2018). Biodegradability Standards for Carrier Bags and Plastic Films in Aquatic Environments: a Critical Review. R. Soc. Open Sci. 5 (5), 171792. Royal Society Open Science. doi:10.1098/rsos.171792
Heidbreder, L. M., Bablok, I., Drews, S., and Menzel, C. (2019). Tackling the Plastic Problem: A Review on Perceptions, Behaviors, and Interventions. Sci. Total Environ. 668, 1077–1093. doi:10.1016/j.scitotenv.2019.02.437
Henton, D. E., Gruber, P., Lunt, J., and Randall, J. (2005). Polylactic Acid Technology. Nat. Fibers, Biopolymers, Biocomposites 16, 527–577. doi:10.1002/1521-4095(200012)12:23<1841::AID-ADMA1841>3.0.CO;2-E
Itävaara, M., Karjomaa, S., and Selin, J.-F. (2002). Biodegradation of Polylactide in Aerobic and Anaerobic Thermophilic Conditions. Chemosphere 46 (6), 879–885. doi:10.1016/s0045-6535(01)00163-1
Jambeck, J. R., Geyer, R., Wilcox, C., Siegler, T. R., Perryman, M., Andrady, A., et al. (2015). Plastic Waste Inputs from Land into the Ocean. Science 347 (6223), 768–771. doi:10.1126/science.1260352
Kale, G., Kijchavengkul, T., Auras, R., Rubino, M., Selke, S. E., and Singh, S. P. (2007). Compostability of Bioplastic Packaging Materials: an Overview. Macromol. Biosci. 7 (3), 255–277. doi:10.1002/mabi.200600168
Karamanlioglu, M., Preziosi, R., and Robson, G. D. (2017). Abiotic and Biotic Environmental Degradation of the Bioplastic Polymer Poly(lactic Acid): A Review. Polym. Degrad. Stab. 137, 122–130. doi:10.1016/j.polymdegradstab.2017.01.009
Klein, F., Emberger-Klein, A., Menrad, K., Möhring, W., and Blesin, J.-M. (2019). Influencing Factors for the Purchase Intention of Consumers Choosing Bioplastic Products in Germany. Sustainable Prod. Consumption 19, 33–43. doi:10.1016/j.spc.2019.01.004
Klöckner, C. A. (2013). A Comprehensive Model of the Psychology of Environmental Behaviour-A Meta-Analysis. Glob. Environ. Change 23 (5), 1028–1038. doi:10.1016/j.gloenvcha.2013.05.014
Kyrikou, I., and Briassoulis, D. (2007). Biodegradation of Agricultural Plastic Films: a Critical Review. J. Polym. Environ. 15 (2), 125–150. doi:10.1007/s10924-007-0053-8
Lee, K.-M., Gimore, D. F., and Huss, M. J. (2005). Fungal Degradation of the Bioplastic PHB (Poly-3-Hydroxy- Butyric Acid). J. Polym. Environ. 13 (3), 213–219. doi:10.1007/s10924-005-4756-4
Lucas, N., Bienaime, C., Belloy, C., Queneudec, M., Silvestre, F., and Nava-Saucedo, J.-E. (2008). Polymer Biodegradation: Mechanisms and Estimation Techniques - A Review. Chemosphere 73 (4), 429–442. doi:10.1016/j.chemosphere.2008.06.064
Luckachan, G. E., and Pillai, C. K. S. (2011). Biodegradable Polymers- A Review on Recent Trends and Emerging Perspectives. J. Polym. Environ. 19 (3), 637–676. doi:10.1007/s10924-011-0317-1
Lynch, D. H. J., Klaassen, P., and Broerse, J. E. W. (2017). Unraveling Dutch Citizens' Perceptions on the Bio-Based Economy: The Case of Bioplastics, Bio-Jetfuels and Small-Scale Bio-Refineries. Ind. Crops Prod. 106, 130–137. doi:10.1016/j.indcrop.2016.10.035
Marsh, K., and Bugusu, B. (2007). Food Packaging?Roles, Materials, and Environmental Issues. J. Food Sci. 72 (3), R39–R55. doi:10.1111/j.1750-3841.2007.00301.x
Martin, R. T., Camargo, L. P., and Miller, S. A. (2014). Marine-degradable Polylactic Acid. Green. Chem. 16 (4), 1768–1773. doi:10.1039/c3gc42604a
Mason, S. A., Welch, V. G., and Neratko, J. (2018). Synthetic Polymer Contamination in Bottled Water. Front. Chem. 6, 407. doi:10.3389/fchem.2018.00407
Massardier-Nageotte, V., Pestre, C., Cruard-Pradet, T., and Bayard, R. (2006). Aerobic and Anaerobic Biodegradability of Polymer Films and Physico-Chemical Characterization. Polym. Degrad. Stab. 91 (3), 620–627. doi:10.1016/j.polymdegradstab.2005.02.029
Mathur, S. P. (1998). “Composting Processes,” in Bioconversion of Waste Materials to Industrial Products (Boston, MA: Springer), 154–193. doi:10.1007/978-1-4615-5821-7_4
Mekonnen, T., Mussone, P., Khalil, H., and Bressler, D. (2013). Progress in Bio-Based Plastics and Plasticizing Modifications. J. Mater. Chem. A. 1 (43), 13379–13398. doi:10.1039/c3ta12555f
Pathak, S., Sneha, C., and Mathew, B. B. (2014). Bioplastics: its Timeline Based Scenario & Challenges. J. Polym. Biopolymer Phys. Chem. 2 (4), 84–90.
Pischedda, A., Tosin, M., and Degli-Innocenti, F. (2019). Biodegradation of Plastics in Soil: The Effect of Temperature. Polym. Degrad. Stab. 170, 109017. doi:10.1016/j.polymdegradstab.2019.109017
Pranamuda, H., Tokiwa, Y., and Tanaka, H. (1997). Polylactide Degradation by an Amycolatopsis Sp. Appl. Environ. Microbiol. 63 (4), 1637–1640. doi:10.1128/aem.63.4.1637-1640.1997
Quecholac-Piña, X., Hernández-Berriel, M. d. C., Mañón-Salas, M. d. C., Espinosa-Valdemar, R. M., and Vázquez-Morillas, A. (2020). Degradation of Plastics under Anaerobic Conditions: a Short Review. Polymers 12 (1), 109. doi:10.3390/polym12010109
Razza, F., Degli Innocenti, F., Dobon, A., Aliaga, C., Sanchez, C., and Hortal, M. (2015). Environmental Profile of a Bio-Based and Biodegradable Foamed Packaging Prototype in Comparison with the Current Benchmark. J. Clean. Prod. 102, 493–500. doi:10.1016/j.jclepro.2015.04.033
Rudnik, E., and Briassoulis, D. (2011). Degradation Behaviour of Poly(lactic Acid) Films and Fibres in Soil under Mediterranean Field Conditions and Laboratory Simulations Testing. Ind. Crops Prod. 33 (3), 648–658. doi:10.1016/j.indcrop.2010.12.031
Rujnić-Sokele, M., and Pilipović, A. (2017). Challenges and Opportunities of Biodegradable Plastics: A Mini Review. Waste Manage. Res. 35 (2), 132–140.
Sadi, R. K., Fechine, G. J. M., and Demarquette, N. R. (2010). Photodegradation of Poly(3-Hydroxybutyrate). Polym. Degrad. Stab. 95 (12), 2318–2327. doi:10.1016/j.polymdegradstab.2010.09.003
Sarasa, J., Gracia, J. M., and Javierre, C. (2009). Study of the Biodisintegration of a Bioplastic Material Waste. Bioresour. Tech. 100 (15), 3764–3768. doi:10.1016/j.biortech.2008.11.049
Shah, A. A., Hasan, F., Hameed, A., and Ahmed, S. (2008). Biological Degradation of Plastics: a Comprehensive Review. Biotechnol. Adv. 26 (3), 246–265. doi:10.1016/j.biotechadv.2007.12.005
Shen, J., and Burgess, D. J. (2012). Accelerated In-Vitro Release Testing Methods for Extended-Release Parenteral Dosage Forms. J. Pharm. Pharmacol. 64 (7), 986–996. doi:10.1111/j.2042-7158.2012.01482.x
Shin, P. K., Kim, M. H., and Kim, J. M. (1997). Biodegradability of Degradable Plastics Exposed to Anaerobic Digested Sludge and Simulated Landfill Conditions. J. Environ. Polym. Degrad. 5 (1), 33–39.
Shogren, R. L., Doane, W. M., Garlotta, D., Lawton, J. W., and Willett, J. L. (2003). Biodegradation of Starch/polylactic Acid/poly(hydroxyester-Ether) Composite Bars in Soil. Polym. Degrad. Stab. 79 (3), 405–411. doi:10.1016/s0141-3910(02)00356-7
Song, J. H., Murphy, R. J., Narayan, R., and Davies, G. B. H. (2009). Biodegradable and Compostable Alternatives to Conventional Plastics. Phil. Trans. R. Soc. B 364 (1526), 2127–2139. doi:10.1098/rstb.2008.0289
Soroudi, A., and Jakubowicz, I. (2013). Recycling of Bioplastics, Their Blends and Biocomposites: A Review. Eur. Polym. J. 49 (10), 2839–2858. doi:10.1016/j.eurpolymj.2013.07.025
Spiridon, I., Leluk, K., Resmerita, A. M., and Darie, R. N. (2015). Evaluation of PLA-Lignin Bioplastics Properties before and after Accelerated Weathering. Composites B: Eng. 69, 342–349. doi:10.1016/j.compositesb.2014.10.006
Sudesh, K., and Iwata, T. (2008). Sustainability of Biobased and Biodegradable Plastics. Clean. Soil Air Water 36 (5‐6), 433–442. doi:10.1002/clen.200700183
Thakur, S., Chaudhary, J., Sharma, B., Verma, A., Tamulevicius, S., and Thakur, V. K. (2018). Sustainability of Bioplastics: Opportunities and Challenges. Curr. Opin. Green Sustain. Chem. 13, 68–75. doi:10.1016/j.cogsc.2018.04.013
Thellen, C., Coyne, M., Froio, D., Auerbach, M., Wirsen, C., and Ratto, J. A. (2008). A Processing, Characterization and Marine Biodegradation Study of Melt-Extruded Polyhydroxyalkanoate (PHA) Films. J. Polym. Environ. 16 (1), 1–11. doi:10.1007/s10924-008-0079-6
Tokiwa, Y., Calabia, B., Ugwu, C., and Aiba, S. (2009). Biodegradability of Plastics. Ijms 10 (9), 3722–3742. doi:10.3390/ijms10093722
Tsuji, H., and Suzuyoshi, K. (2002a). Environmental Degradation of Biodegradable Polyesters 1. Poly(ε-Caprolactone), poly[(R)-3-hydroxybutyrate], and poly(L-Lactide) Films in Controlled Static Seawater. Polym. Degrad. Stab. 75 (2), 347–355. doi:10.1016/s0141-3910(01)00240-3
Tsuji, H., and Suzuyoshi, K. (2002b). Environmental Degradation of Biodegradable Polyesters 2. Poly(ε-Caprolactone), poly[(R)-3-hydroxybutyrate], and poly(L-Lactide) Films in Natural Dynamic Seawater. Polym. Degrad. Stab. 75 (2), 357–365. doi:10.1016/s0141-3910(01)00239-7
UN Environment (2018). SINGLE-USE PLASTICS: A Roadmap for Sustainability. Nairobi: United Nations Environment Programme.
UNEP (2015). Biodegradable Plastics and Marine Litter. Misconceptions, Concerns and Impacts on Marine Envionments. Nairobi: United Nations Environment Programme.
Volova, T. G., Boyandin, A. N., Vasiliev, A. D., Karpov, V. A., Prudnikova, S. V., Mishukova, O. V., et al. (2010). Biodegradation of Polyhydroxyalkanoates (PHAs) in Tropical Coastal Waters and Identification of PHA-Degrading Bacteria. Polym. Degrad. Stab. 95 (12), 2350–2359. doi:10.1016/j.polymdegradstab.2010.08.023
Volova, T. G., Gladyshev, M. I., Trusova, M. Y., and Zhila, N. O. (2007). Degradation of Polyhydroxyalkanoates in Eutrophic Reservoir. Polym. Degrad. Stab. 92 (4), 580–586. doi:10.1016/j.polymdegradstab.2007.01.011
Wagner, M., Scherer, C., Alvarez-Muñoz, D., Brennholt, N., Bourrain, X., Buchinger, S., et al. (2014). Microplastics in Freshwater Ecosystems: what We Know and what We Need to Know. Environ. Sci. Europe 26 (1), 1–9. doi:10.1186/s12302-014-0012-7
Wang, G. X., Huang, D., Ji, J. H., Völker, C., and Wurm, F. R. (2020). Seawater‐Degradable Polymers—Fighting the Marine Plastic Pollution. Adv. Sci, 2001121. doi:10.1002/advs.202001121
Watson, S. W., Novitsky, T. J., Quinby, H. L., and Valois, F. W. (1977). Determination of Bacterial Number and Biomass in the Marine Environment. Appl. Environ. Microbiol. 33 (4), 940–946. doi:10.1128/aem.33.4.940-946.1977
Wei, L., Liang, S., and McDonald, A. G. (2015). Thermophysical Properties and Biodegradation Behavior of Green Composites Made from Polyhydroxybutyrate and Potato Peel Waste Fermentation Residue. Ind. Crops Prod. 69, 91–103. doi:10.1016/j.indcrop.2015.02.011
Woolnough, C. A., Charlton, T., Yee, L. H., Sarris, M., and Foster, L. J. R. (2008). Surface Changes in Polyhydroxyalkanoate Films during Biodegradation and Biofouling. Polym. Int. 57 (9), 1042–1051. doi:10.1002/pi.2444
Wright, R. J., Erni-Cassola, G., Zadjelovic, V., Latva, M., and Christie-Oleza, J. A. (2020). Marine Plastic Debris: A New Surface for Microbial Colonization. Environ. Sci. Technol. 54 (19), 11657–11672. doi:10.1021/acs.est.0c02305
Wu, C.-S. (2014). Preparation and Characterization of Polyhydroxyalkanoate Bioplastic-Based Green Renewable Composites from Rice Husk. J. Polym. Environ. 22 (3), 384–392. doi:10.1007/s10924-014-0662-y
Yagi, H., Ninomiya, F., Funabashi, M., and Kunioka, M. (2014). Mesophilic Anaerobic Biodegradation Test and Analysis of Eubacteria and Archaea Involved in Anaerobic Biodegradation of Four Specified Biodegradable Polyesters. Polym. Degrad. Stab. 110, 278–283. doi:10.1016/j.polymdegradstab.2014.08.031
Keywords: bioplastics, biodegradable plastics, biodegradable products, misconception, sustainability, biopolymer, biodegradation
Citation: Choe S, Kim Y, Won Y and Myung J (2021) Bridging Three Gaps in Biodegradable Plastics: Misconceptions and Truths About Biodegradation. Front. Chem. 9:671750. doi: 10.3389/fchem.2021.671750
Received: 24 February 2021; Accepted: 26 April 2021;
Published: 14 May 2021.
Edited by:
Taner Yonar, Uludağ University, TurkeyReviewed by:
Francesco Degli-Innocenti, Novamont S.p.A. - Chimica Vivente per la Qualità della Vita, ItalyCopyright © 2021 Choe, Kim, Won and Myung. This is an open-access article distributed under the terms of the Creative Commons Attribution License (CC BY). The use, distribution or reproduction in other forums is permitted, provided the original author(s) and the copyright owner(s) are credited and that the original publication in this journal is cited, in accordance with accepted academic practice. No use, distribution or reproduction is permitted which does not comply with these terms.
*Correspondence: Jaewook Myung, amphaW15dW5nQGthaXN0LmFjLmty
Disclaimer: All claims expressed in this article are solely those of the authors and do not necessarily represent those of their affiliated organizations, or those of the publisher, the editors and the reviewers. Any product that may be evaluated in this article or claim that may be made by its manufacturer is not guaranteed or endorsed by the publisher.
Research integrity at Frontiers
Learn more about the work of our research integrity team to safeguard the quality of each article we publish.