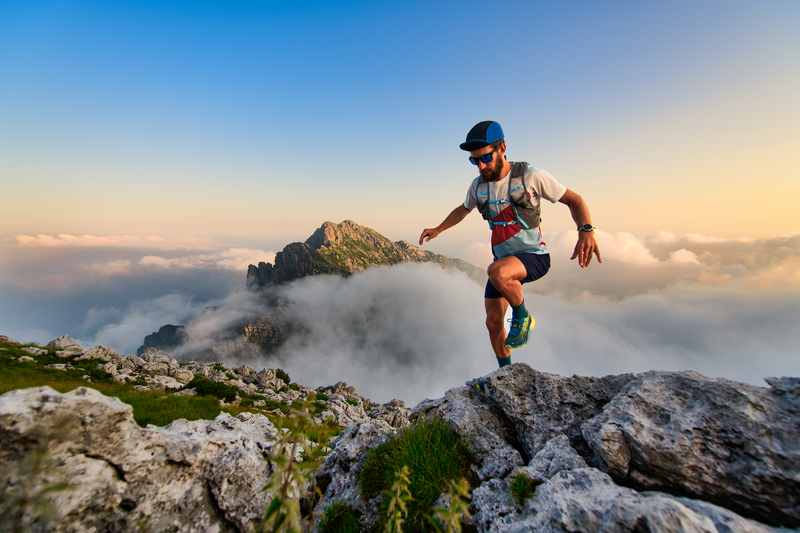
95% of researchers rate our articles as excellent or good
Learn more about the work of our research integrity team to safeguard the quality of each article we publish.
Find out more
ORIGINAL RESEARCH article
Front. Chem. , 01 February 2021
Sec. Electrochemistry
Volume 9 - 2021 | https://doi.org/10.3389/fchem.2021.646218
This article is part of the Research Topic Design, Synthesis, and Modification of Metal Oxides for Energy Storage View all 12 articles
A high energy efficiency, low charging voltage cathode is of great significance for the development of non-aqueous lithium-oxygen batteries. Non-stoichiometric manganese dioxide (MnO2-x) and chromium trioxide (Cr2O3) are known to have good catalytic activities for the discharging and charging processes, respectively. In this work, we prepared a cathode based on Cr2O3 decorated MnO2-x nanosheets via a simple anodic electrodeposition-electrostatic adsorption-calcination process. This combined fabrication process allowed the simultaneous introduction of abundant oxygen vacancies and trivalent manganese into the MnO2-x nanosheets, with a uniform load of a small amount of Cr2O3 on the surface of the MnO2-x nanosheets. Therefore, the Cr2O3/MnO2-x electrode exhibited a high catalytic effect for both discharging and charging, while providing high energy efficiency and low charge voltage. Experimental results show that the as-prepared Cr2O3/MnO2-x cathode could provide a specific capacity of 6,779 mA·h·g−1 with a terminal charge voltage of 3.84 V, and energy efficiency of 78%, at a current density of 200 mA·g−1. The Cr2O3/MnO2-x electrode also showed good rate capability and cycle stability. All the results suggest that the as-prepared Cr2O3/MnO2-x nanosheet electrode has great prospects in non-aqueous lithium-oxygen batteries.
Non-aqueous lithium-oxygen batteries have been considered as a promising power source for portable devices and electric vehicles due to their high energy density (1.14 × 104 Wh kg−1) (Wang et al., 2019; Kwak et al., 2020). However, several issues, such as high charge voltage, low energy efficiency, and short cycle life, need to be addressed before this technology is commercially viable (Eftekhari and Ramanujam, 2017; Wang et al., 2018). The abovementioned issues can be mainly attributed to the sluggish reaction dynamics of both, the cathode oxygen reduction reaction (ORR) and the oxygen evolution reaction (OER) (Li and Chen, 2017; Lim et al., 2017; Huang and Peng, 2019). Typically, during discharge, the lithium metal is oxidized to lithium ion at the negative electrode, and the oxygen is reduced to form an insoluble product, i.e., lithium peroxide (Li2O2), at the positive electrode. During charging, the discharge product, Li2O2, is converted into lithium ions and oxygen by electrochemical decomposition at the positive electrode, and the lithium ions are reduced and deposited at the negative electrode (Liu et al., 2019; Shu et al., 2019). The reaction at the negative electrode is known to be more reversible, with a faster reaction rate, while the reaction at the positive electrode suffers from poor reversibility and low reaction rate (Lim et al., 2017; Shu et al., 2019). Therefore, the ORR/OER reaction resistance dominates the total resistance. Many catalytic materials have been prepared to promote the electrochemical reaction and improve battery performance, such as carbon-based materials (Wu et al., 2020b; Li et al., 2020c; Falinski et al., 2020; Ren et al., 2020), noble metal/metal oxides (Li et al., 2020a; Nam et al., 2020; Zhu et al., 2020), and transition metal oxides (Wu et al., 2020a; Cai et al., 2020; Song et al., 2020).
Manganese dioxide (MnO2) based materials have received great attention as cathodes for lithium-oxygen batteries, because of their good stability and excellent catalytic activity for oxygen reduction reactions (Bi et al., 2019; Cheng et al., 2019; Yao et al., 2019; Dai et al., 2020). Among MnO2-based materials, non-stoichiometric manganese dioxide (MnO2-x), which contains oxygen vacancies and trivalent manganese, can significantly increase the conductivity and enhance the adsorption of oxygen species on the electrode surface (Zhai et al., 2014; Chen et al., 2015; Liu et al., 2018). Several studies have been conducted which demonstrate the high discharge capacity of MnO2-x (Song et al., 2013; Hu et al., 2014). However, its catalytic activity for oxygen evolution reaction, during the charging process, is still not satisfactory and needs to be further improved (Luo et al., 2018; Zhang et al., 2019).
Chromium trioxide (Cr2O3) has a unique catalytic effect on the charging process of lithium-oxygen batteries. Yao et al. first studied its charging performance and showed that chromium-based materials could promote the discharge product decomposition through a solid-activation process by the mixed valence states Cr3+/Cr6+ on the interface at the Li2O2/Cr2O3 interface (Yao et al., 2014). Since then, a number of works have been carried out, and the results proved the high OER catalytic ability of Cr2O3 in lithium-oxygen batteries (Gan et al., 2016; Zhang et al., 2016). Thus, it is highly beneficial to use Cr2O3 to improve the charge performance of the MnO2-x cathode.
In this work, we prepared a cathode based on Cr2O3 decorated MnO2-x nanosheets using a simple adsorption process and applied this novel cathode as a binder-free, non-carbon cathode for non-aqueous lithium-oxygen batteries. In this electrode, a small number of Cr2O3 particles are uniformly decorated on the MnO2-x surface, providing the following three advantages: 1) the MnO2-x nanosheets provide high surface area for ORR and deliver high discharge capacity; 2) Cr2O3 promotes the formation of discharge product to achieve low charging voltage; and 3) a low loading of evenly distributed Cr2O3 on the surface of MnO2-x nanosheets can minimize the inhibition effect on the oxygen reduction process and catalyze the Li2O2 formation. These striking features enable the Cr2O3/MnO2-x nanosheet electrode to achieve high discharge capacity, high energy efficiency, and low charge voltage.
The MnO2 nanosheet electrode was prepared with a simple electrodeposition method. Manganese acetate (C4H6MnO4·4H2O), chromium nitrate (Cr(NO3)3·9H2O), and sodium sulfate (Na2SO4) were purchased from Aladdin, China. Stainless steel (SS) felt substrates were first cleaned in an H2SO4 solution, rinsed in distilled (DI) water, and air-dried at 60°C. The SS felt substrate was then immersed in a solution containing 0.1 M Na2SO4 and C4H6MnO4 with an anodic current density of 0.25 mA·cm−2 to obtain an electrodeposited layer of MnO2 nanosheets. Then, the as-obtained deposition was rinsed in DI water, dried at 90°C for 12 h, and was used as the MnO2 electrode. The loading of MnO2 was controlled to achieve 0.5 mg·cm−2 by adjusting the electrodepositing time and weighing.
After electrodeposition, the as-prepared MnO2 electrode was immersed in a 0.1 M (NH4)2CrO4 solution for 6 h to achieve the full adsorption of Cr ions. After the above treatment, the electrode was taken out of the solution, and the remaining liquid on the electrode surface was wiped dry by filter paper. The electrode was dried at 90°C, and calcined at 350 °C for 3 h, under N2 atmosphere. The resulting electrode was named Cr2O3@MnO2-x. For comparison, the MnO2-SS electrode, without chromium adsorption, was also calcined under the same conditions and named as MnO2-x.
The as-prepared electrodes were cut into a 14 mm discs and used as the cathode in non-aqueous lithium-oxygen batteries. The battery performance was tested in a lithium-oxygen battery developed in-house, with a lithium metal anode, a separator (GF/C, Whatman), 200 μl 1.0 M Lithium bis(trifluoromethanesulphonyl)imide (LiTFSI)-tetraethylene glycol dimethyl ether (TEGDME) electrolyte, and a cathode. The battery was assembled in a glove box and then tested in an O2 atmosphere, at a pressure of 1.25 atm.
The electrochemical performance of the electrode was first tested through electrochemical impedance spectra (EIS) and cyclic voltammetry (CV). All the tests were carried out in an electrochemical workstation (CHI660, Shanghai Chenhua). The discharge-charge tests were conducted on a battery testing system (CT-4008, Neware) at current densities of 200, 400, and 800 mA·g−1. The cycle stability was tested in a homemade Li+-oxygen batteries at the current density of 400 mA·g−1, with a fixed specific capacity of 1,000 mAh·g−1. A LiFePO4 electrode was used as the reference electrode and counter electrode in the Li+-oxygen batteries and the electrode was prepared as follows: lithium iron phosphate (LiFePO4, MTI Corporation), acetylene Black (AB, Canrd) and Poly tetra fluoroethylene (PTFE, Canrd) (85:5:10 wt%) were thoroughly mixed and pressed on a stainless steel mesh (16 mm diameter) and then dried under vacuum at 120°C for 12 h. To ensure the voltage stability, a ∼10-fold excess of LiFePO4 was applied in the Li+-oxygen batteries. All tests were carried out at room temperature (25 ± 1°C).
The crystal structures of different samples were tested with an X-ray diffraction system (XRD, D/max2500/PC). The morphologies were obtained by scanning electron microscopy (SEM, S-4700) and scanning transmission electron microscopy (STEM, FEI TECNAI G2F20S-TWIN). The valence states of Mn and Cr were characterized by X-ray photoelectron spectroscopy (XPS) on an Axis Ultra spectrometer. The composition of the as-prepared manganese oxide was tested by the iodometry method. The discharge product was analyzed by XPS and Raman spectroscopy (RENISHAW in Via, wave length 532 nm). The morphology of the product was observed by SEM.
The SEM images of different electrodes were shown in Figure 1. From these images, we can clearly find that the MnO2 prepared by electrodeposition exhibits an irregular nanosheet structure, with a thickness of ∼50 nm. These nanosheets are expected to provide a high surface area for chromium adsorption and electrochemical reactions in lithium-oxygen batteries. After the simple calcination or adsorption-calcination process, the electrode morphology was characterized, as shown in Figures 1C–F, respectively. The results show that after the calcination or adsorption-calcination process, the surface morphology of the electrode remains unchanged. Therefore, the improvement in the charge-discharge performance of the electrode can be attributed to the change of the surface state.
XRD and XPS were measured to investigate the composition and valence state of the elements of the different electrodes, as shown in Figure 2. To avoid the influence of the SS substrate, before the test, the electrode materials on the electrode surface were peeled away by ultrasonication and collected. XRD showed that these patterns exhibited a group of diffraction peaks at 37.1°, 42.4°, 56.0°, and 66.7°, which matched well with the diffraction of the (100), (101), (102), and (110) planes of the ε-MnO2 (akhtenskite, Joint Committee on Powder Diffraction Standards no. 33-0820). This result indicates that the as-prepared MnO2 was mainly ε-MnO2 and that after the adsorption-calcination process, the crystal structure did not change significantly.
FIGURE 2. (A) XRD patterns for different electrode materials; (B) Deconvoluted Mn 2p3/2 spectra of different electrode materials; (C) Deconvoluted Cr (2p) spectra of Cr2O3/MnO2-x; (D) Deconvoluted O (1s) spectra of different electrode materials.
XPS was used to identify the valence states of Mn, Cr, and O their species in the different samples. Figure 2B shows the deconvoluted Mn 2p3/2 peak of the different electrodes, where the peaks at 642.4 and 641.8 eV correspond to Mn (IV) and Mn (III), respectively. The results show that after the calcination/adsorption-calcination, the peak intensity of Mn (III) increased while the peak intensity of Mn (IV) decreased, revealing that the oxygen vacancies and the associated Mn (III) were generated during the heat treatment. These oxygen vacancies are expected to increase the catalytic oxygen reduction activity of the MnO2-based materials, thereby achieving high discharge capacity.
The existence of Cr on MnO2-x nanosheets was studied by XPS, as shown in Figure 2C. The test results show that Cr mainly exists in the mixed form of Cr (III) and Cr (VI):The peaks located at 576.8 and 586.4 eV are corresponding to Cr (III) and the peaks located at 578 and 587.8 eV can be attributed to the Cr (VI) within the Cr2O3 (Yao et al., 2014; Gan et al., 2016). The mixed states of Cr in Cr2O3/MnO2-x are expected to have high catalytic activity in promoting charge process. The O 1s spectrum, in Figure 2D, shows two peaks at 529.8, and 531.5 eV, which are correlated to the normal lattice oxygen (Olatt), and adsorbed oxygen (Oabs), respectively. Since the surface adsorbed oxygen mainly occurs at the oxygen vacancy, the oxygen vacancy content can be known through the analysis of Oabs (Li et al., 2020b; Mo et al., 2020). Through the O 1s spectrum, it can be found that the proportion of Oabs increases after the calcination and adsorption-calcination process, indicating that the process can introduce more oxygen vacancies in the MnO2 structure. The increase in oxygen vacancies is consistent with the Mn 2p spectrum and is expected to be beneficial for ORR. Iodometry and Inductively Coupled Plasma Optical Emission Spectrometer (ICP-OES) were also used to determine the composition of the different electrode materials. The composition of MnO2, MnO2-x and Cr2O3/MnO2-x was found to be MnO1.99, MnO1.96, and 0.004Cr2O3/MnO1.96, respectively.
To investigate the distribution of Cr2O3 on the MnO2-x nanosheets after adsorption-calcination, STEM-EDS mapping was carried out, as shown in Figure 3. The TEM images (Figure 3A) show that the electrodeposited MnO2-x material is in the form of nanosheets, and the diameter of the Cr2O3/MnO2-x nanosheet is consistent with that obtained from the SEM results. EDS mapping (Figures 3B–D) shows that chromium is evenly distributed on the MnO2-x nanosheets. The surface Cr/Mn ratio of the Cr2O3/MnO2-x was calculated to be 0.153 from the elements abundance test from STEM-EDS mapping (Supplementary Figure S1). This uniform dispersion can be attributed to the uniform adsorption process on the MnO2 surface. Thus, chromium can be uniformly loaded on the surface of MnO2-x under low loading, which has two advantages: 1) the low Cr2O3 loading can minimize the inhibition effect to MnO2-x for the discharge process of lithium-oxygen batteries; and 2) uniform chromium distribution is conducive to achieve uniform contact with the discharge product, Li2O2, so as to achieve high catalytic effect in the charging process.
From the SEM, XRD, XPS, iodometry and ICP-OES and TEM results, it can be concluded that: 1) a MnO2 film can be grown on the surface of the substrate by electrodeposition; 2) the adsorption process can adsorb chromium species on the surface of the MnO2; and 3) the subsequent calcination process introduces a large amount of oxygen vacancies and Mn (III) into manganese dioxide, and at the same time, converts chromium species to Cr2O3. The above results indicate that the Cr2O3 decorated MnO2-x nanosheet electrode can be prepared by the combined electrodeposition-adsorption-calcination method. The electrode has a high oxygen vacancy content, low Cr2O3 loading, and uniform Cr2O3 distribution, and can deliver high discharge capacity and low charge voltage.
Before the charge and discharge test, the impedance characteristics of different electrodes in lithium-oxygen batteries were studied by AC impedance and fitted with a simple equivalent circuit mode, and the results are shown in Figure 4A. In this model, RΩ corresponds to the ohmic resistance of electrolyte and electrode materials, Rct represents charge transfer resistance for ORR/OER on the cathode-electrolyte interface, CPE is the constant phase element corresponds to the cathode-electrolyte interfaces and W is the finite length Warburg contribution. The fitting results show that the ohmic resistance of MnO2, MnO2-x and Cr2O3/MnO2-x electrode was calculated to be 15.1, 13.7 and 13.9 Ω, respectively. At the same time, the charge transport resistance of MnO2, MnO2-x and Cr2O3/MnO2-x electrode was calculated to be 43.4, 36.6 and 38.6 Ω, respectively. This result can be attributed to three reasons: 1) oxygen defects and Mn (III) generated during the calcination and adsorption-calcination process can increase the electronic conductivity of the MnO2-x nanosheets, thereby reducing the ohmic resistance of the electrode material; 2) oxygen vacancies can improve the adsorption of oxygen species on the surface of the MnO2-based material, thereby obtaining good catalytic activity for oxygen reduction and lower charge transfer resistance; 3) the small amount of Cr2O3 is uniformly distributed on the MnO2-x nanosheets, thereby reducing the inhibitory effect of Cr2O3 on oxygen reduction to a minimum. Therefore, the charge transfer impedances of the MnO2-x electrode and the Cr2O3/MnO2-x electrode are close in value.
FIGURE 4. (A) EIS plots and simulated results for different electrode (insert: the equivalent circuit model for EIS); (B) Discharge-charge performances of different electrodes at 200 mA·g−1; (C) High current discharge-charge performances of the Cr2O3/MnO2-x electrode; (D) Calculated energy efficiency of different electrodes at different current densities.
Figure 4B shows the galvanostatic discharge/charge performance of the three electrodes in non-aqueous lithium-oxygen batteries, under a current density of 200 mA·g−1. The MnO2 electrode delivers a discharge capacity of 4,801 mAh·g−1 with a terminal charge voltage of 4.14 V. Under the same conditions, the MnO2-x electrode and Cr2O3/MnO2-x electrode exhibit specific discharge capacities of 6,854 mAh·g−1 and 6,779 mAh·g−1, respectively. More importantly, the Cr2O3/MnO2-x electrode delivers a reduced terminal charging voltage of 3.84 V. The energy efficiencies of the MnO2, MnO2-x, and Cr2O3/MnO2-x electrodes were calculated to be 66%, 65%, and 78%, respectively. This result suggests that the Cr2O3/MnO2-x electrode exhibits a high specific capacity, the lowest charge voltage, and the highest energy efficiency.
MnO2-based materials can work as anode active materials in lithium-ion batteries through the lithiation and delithiation reactions, thus showing discharge-charge capacity. In order to exclude the capacity of lithiation and delithiation capacity, the specific capacities of different electrodes were tested in sealed button cells under the same current density. The results (Supplementary Figure S2) show that the lithiation and delithiation capacities of the MnO2, MnO2-x and Cr2O3/MnO2-x electrodes were about 225–250 mAh·g−1, which can be ignored compared with the capacity in lithium-oxygen batteries.
The rate capability of the as-prepared MnO2, MnO2-x and Cr2O3/MnO2-x electrodes are tested under different current densities, and the results are shown in Supplementary Figure S3, and Figures 4C,D, respectively. The Cr2O3/MnO2-x electrode delivers specific discharge capacities of 6,779, 5,033, and 2,627 mAh·g−1 with the energy efficiencies 78%, 74%, and 72%, under the current densities 200, 400, 800 mA g−1, respectively (Figures 4C,D). At the same time, the MnO2 and MnO2-x cathodes can deliver specific capacities of 4,801/6,854, 3,712/5,147, and 2,083/2,706 mAh· g−1 with energy efficiencies, 66/65%, 63/62%, and 62/61, respectively.
The discharge products of Cr2O3/MnO2-x were characterized by SEM, XPS, and Raman spectroscopy, as shown in Figure 5. The SEM image (Figure 5A) shows that the surface of the nanosheets is covered by film-like products after discharge. This film-like product can achieve sufficient contact with the catalyst surface, ensuring good catalytic activity during charging. After charging, the SEM image (Figure 5B) shows that the film-like product completely disappears and that the electrode surface recovers to the same as before discharge. Li 1s XPS spectra (Figure 5C) were obtained from the electrode after cycling to analyze the composition of the discharge product. In the discharged electrode, the Li 1s peak was mainly composed of Li2O2 and a small amount of li-based impurities which come from contamination during testing or parasitic reactions, indicating that Li2O2 had decomposed on the catalyst during the charging process. Raman spectroscopy (Figure 5D) was also used to study the existence of discharge products on the electrode surface after cycling. The results show that the product after discharge was mainly in the form of Li2O2 and that the Li2O2 peak disappeared after charging, which is consistent with the XPS results.
FIGURE 5. Characterizations of the discharge products on Cr2O3/MnO2-x: (A), (B) morphology of the Cr2O3/MnO2-x electrode after discharge and charge; (C) Li 1s spectra of the Cr2O3/MnO2-x electrode after discharge and charge; (D) Raman spectra of the Cr2O3/MnO2-x electrode after discharge and charge.
The stability of the Cr2O3/MnO2-x electrode was tested in the in-house Li+-oxygen battery, using LiFePO4 as the counter electrode to eliminate the influence of lithium electrode on the cycle performance. The capacity of the battery was limited to 1,000 mAh·g−1, and the battery voltage and the cycle performance are shown in Figure 6A. This experimental result shows that the battery can stably cycle for 100 cycles in the voltage range of 2.0–4.5 V without capacity degradation, but its discharge terminal voltages decrease from 2.693 to 2.530 V with the charge terminal voltages increase from 3.985 to 4.022 V. This voltage change can be attribute to the accumulation of minor by-products rather than by material degradation. To verify this hypothesis, the cycled battery was disassembled, cleaned, and reassembled with a fresh lithium anode, separator, and electrolyte for testing. The discharge/charge performance in Figure 6B shows that the reassembled battery could achieve the same charge and discharge performance as that of a fresh battery, indicating good stability of the Cr2O3/MnO2-x electrode.
FIGURE 6. (A) Cycle performance of the Cr2O3/MnO2-x cathode electrode under the current density of 400 mA·g−1 with the fixed capacity of 1,000 mAh·g−1; (B) Discharge-charge performance of the reassembled battery and the initial battery.
In this work, we have prepared an electrode based on Cr2O3 decorated MnO2-x nanosheets as a non-carbon and binder-free cathode for lithium-oxygen batteries. The as-prepared Cr2O3/MnO2-x electrode contains abundant oxygen vacancies and Mn (III) and uniformly distributed Cr2O3. With this novel electrode, a specific capacity of 6,779 mAh·g−1, terminal charge voltage of 3.84 V and energy efficiency of 78% were achieved in the non-aqueous lithium-oxygen battery. In addition, this electrode also showed good performance in rate capability tests. SEM, Raman spectroscopy, and XPS demonstrate that the film-like Li2O2 is deposited on the surface of the electrode as the main discharge product and is fully decomposed in the subsequent charging process. Furthermore, the cycling performances of freshly assembled and reassembled batteries show the good stability of the Cr2O3/MnO2-x electrode. Thus, this work shows that the Cr2O3/MnO2-x electrode is an important candidate for non-aqueous lithium-oxygen batteries.
The original contributions presented in the study are included in the article/Supplementary Material, further inquiries can be directed to the corresponding authors.
ZW and YR designed the study and prepared the manuscript. ZW and ZZ performed the experiments. HZ was involved in the discussion of the experimental results and revision of the manuscript. All authors read and approved the final manuscript. All authors agree to be accountable for the content of the work.
This work is supported by National Natural Science Foundation of China (Grant no. 51702040), Sichuan Province Science and Technology Support Program (no. 2020YJ0392).
The authors declare that the research was conducted in the absence of any commercial or financial relationships that could be construed as a potential conflict of interest.
The Supplementary Material for this article can be found online at: https://www.frontiersin.org/articles/10.3389/fchem.2021.646218/full#supplementary-material.
Bi, R., Liu, G. X., Zeng, C., Wang, X. P., Zhang, L., and Qiao, S. Z. (2019). 3D hollow alpha-MnO2 framework as an efficient electrocatalyst for lithium-oxygen batteries. Small 15 (10), 7. doi:10.1002/smll.201804958
Cai, K., Qu, T., Lang, X., Li, L., and Zhang, Q. (2020). Novel Ni and Al doped manganese oxide (NixAlyMnzO2) ternary catalyst materials synthesized by a homogeneous precipitation method for high performance air electrodes of lithium-oxygen batteries. Sustainable Energy Fuels 4 (10), 5009–5016. doi:10.1039/d0se00981d
Chen, C., Xu, K., Ji, X., Zhang, B., Miao, L., and Jiang, J. J. (2015). Enhanced electrochemical performance by facile oxygen vacancies from lower valence-state doping for ramsdellite-MnO2. J. Mater. Chem. 3 (23), 12461–12467. doi:10.1039/c5ta01930c
Cheng, H., Xie, J., Cao, G. S., Lu, Y. H., Zheng, D., Jin, Y., et al. (2019). Realizing discrete growth of thin Li2O2 sheets on black phosphorus quantum dots-decorated delta-MnO2 catalyst for long-life lithium-oxygen cells. Energy Storage Materials 23, 684–692. doi:10.1016/j.ensm.2019.02.028
Dai, L. N., Sun, Q., Chen, L. N., Guo, H. H., Nie, X. K., Cheng, J., et al. (2020). Ag doped urchin-like α-MnO2 toward efficient and bifunctional electrocatalysts for Li-O2 batteries. Nano Res. 13 (9), 2356–2364. doi:10.1007/s12274-020-2855-0
Eftekhari, A., and Ramanujam, B. (2017). In pursuit of catalytic cathodes for lithium-oxygen batteries. J. Mater. Chem. 5 (17), 7710–7731. doi:10.1039/c7ta01124e
Falinski, M. M., Albalghiti, E. M., Backhaus, A., and Zimmerman, J. B. (2020). Performance and sustainability tradeoffs of oxidized carbon nanotubes as a cathodic material in lithium-oxygen batteries. ChemSusChem. doi:10.1002/cssc.202002317
Gan, Y. Q., Lai, Y. Q., Zhang, Z., Chen, W., Du, K., and Li, J. (2016). Hierarchical Cr2O3@OPC composites with octahedral shape for rechargeable nonaqueous lithium-oxygen batteries. J. Alloys Compd. 665, 365–372. doi:10.1016/j.jallcom.2016.01.087
Hu, X. F., Han, X. P., Hu, Y. X., Cheng, F. Y., and Chen, J. (2014). Epsilon-MnO2 nanostructures directly grown on Ni foam: a cathode catalyst for rechargeable Li-O2 batteries. Nanoscale 6 (7), 3522–3525. doi:10.1039/c3nr06361e
Huang, J., and Peng, Z. Q. (2019). Understanding the reaction interface in lithium-oxygen batteries. Batteries Supercaps 2 (1), 37–48. doi:10.1002/batt.201800083
Kwak, W. J., Rosy, D., Xia, C., Kim, H., Johnson, L. R., Bruce, P. G., et al. (2020). Lithium-oxygen batteries and related systems: potential, status, and future. Chem. Rev. 120 (14), 6626–6683. doi:10.1021/acs.chemrev.9b00609
Li, F. J., and Chen, J. (2017). Mechanistic evolution of aprotic lithium-oxygen batteries. Adv. Energy Mater. 7 (24), 12. doi:10.1002/aenm.201602934
Li, K., Dong, H., Wang, Y., Yin, Y., and Yang, S. (2020a). Preparation of low-load Au-Pd alloy decorated carbon fibers binder-free cathode for Li-O2 battery. J. Colloid Interface Sci. 579, 448–454. doi:10.1016/j.jcis.2020.06.084
Li, L., Liu, Y., Zhang, S., Liang, M., Li, F., and Yuan, Y. (2020b). Enhanced mineralization of bisphenol A by eco-friendly BiFeO3-MnO2 composite: performance, mechanism and toxicity assessment. J. Hazard Mater. 399, 122883. doi:10.1016/j.jhazmat.2020.122883
Li, T. W., Li, H. X., Li, H. Z., Xie, Y. Y., and Zhang, Z. (2020c). Invar alloy@nitrogen doped carbon nanotubes as efficient bifunctional catalyst for lithium-oxygen batteries. J. Alloys Compd. 844, 5. doi:10.1016/j.jallcom.2020.156199
Lim, H. D., Lee, B., Bae, Y., Park, H., Ko, Y., Kim, H., et al. (2017). Reaction chemistry in rechargeable Li-O2 batteries. Chem. Soc. Rev. 46 (10), 2873–2888. doi:10.1039/c6cs00929h
Liu, B., Sun, Y. L., Liu, L., Xu, S., and Yan, X. B. (2018). Advances in manganese-based oxides cathodic electrocatalysts for Li-air batteries. Adv. Funct. Mater. 28 (15), 34. doi:10.1002/adfm.201704973
Liu, C., Sato, K., Han, X. B., and Ye, S. (2019). Reaction mechanisms of the oxygen reduction and evolution reactions in aprotic solvents for Li-O2 batteries. Curr. Opin. Electrochem. 14, 151–156. doi:10.1016/j.coelec.2019.02.003
Luo, C. S., Sun, H., Jiang, Z. L., Guo, H. L., Gao, M. Y., Wei, M. H., et al. (2018). Electrocatalysts of Mn and Ru oxides loaded on MWCNTS with 3D structure and synergistic effect for rechargeable Li-O2 battery. Electrochim. Acta 282, 56–63. doi:10.1016/j.electacta.2018.06.040
Mo, S. P., Zhang, Q., Li, J. Q., Sun, Y. H., Ren, Q. M., Zou, S. B., et al. (2020). Highly efficient mesoporous MnO2 catalysts for the total toluene oxidation: oxygen-Vacancy defect engineering and involved intermediates using in situ DRIFTS. Appl. Catal. B Environ. 264, 16. doi:10.1016/j.apcatb.2019.118464
Nam, J. S., Jung, J.-W., Youn, D.-Y., Cho, S.-H., Cheong, J. Y., Kim, M. S., et al. (2020). Free-Standing carbon nanofibers protected by a thin metallic iridium layer for extended life-cycle Li-oxygen batteries. ACS Appl. Mater. Interfaces 12 (50), 55756. doi:10.1021/acsami.0c13325
Ren, J., Yang, X., Yu, J., Wang, X., Lou, Y., and Chen, J. (2020). A MOF derived Co-NC@CNT composite with a 3D interconnected conductive carbon network as a highly efficient cathode catalyst for Li-O2 batteries. Sustainable Energy & Fuels 4 (12), 6105–6111. doi:10.1039/d0se01154a
Shu, C. Z., Wang, J. Z., Long, J. P., Liu, H. K., and Dou, S. X. (2019). Understanding the reaction chemistry during charging in aprotic lithium-oxygen batteries: existing problems and solutions. Adv. Mater. 31 (15), 43. doi:10.1002/adma.201804587
Song, K., Jung, J., Heo, Y.-U., Lee, Y. C., Cho, K., and Kang, Y.-M. (2013). Alpha-MnO2 nanowire catalysts with ultra-high capacity and extremely low overpotential in lithium-air batteries through tailored surface arrangement. Phys. Chem. Chem. Phys. 15 (46), 20075–20079. doi:10.1039/c3cp53754d
Song, K., Yang, B., Li, Z., Lv, Y., Yu, Y., Yuan, L., et al. (2020). Direct synthesis of ACo2O4 (A = Ni, Cu, Fe, Zn) nanowires on carbon cloth as an oxygen electrode catalyst for rechargeable lithium-oxygen batteries. Appl. Surf. Sci. 529, 147064. doi:10.1016/j.apsusc.2020.147064
Wang, D., Mu, X. W., He, P., and Zhou, H. S. (2019). Materials for advanced Li-O2 batteries: explorations, challenges and prospects. Mater. Today 26, 87–99. doi:10.1016/j.mattod.2019.01.016
Wang, K. X., Zhu, Q. C., and Chen, J. S. (2018). Strategies toward high-performance cathode materials for lithium-oxygen batteries. Small 14 (27), 23. doi:10.1002/smll.201800078
Wu, C., Hou, Y. Y., Jiang, J. C., Guo, H. P., Liu, H. K., Chen, J., et al. (2020a). Heterostructured Mo2C-MoO2 as highly efficient catalyst for rechargeable Li-O2 battery. J. Power Sources 470, 7. doi:10.1016/j.jpowsour.2020.228317
Wu, Y., Zhu, X., Ji, X., Liu, W., Wan, W., Wang, Y., et al. (2020b). Graphene quantum dots as a highly efficient electrocatalyst for lithium-oxygen batteries. J. Mater. Chem. 8 (42), 22356–22368. doi:10.1039/d0ta07587f
Yao, K. P. C., Lu, Y. C., Amanchukwu, C. V., Kwabi, D. G., Risch, M., Zhou, J. G., et al. (2014). The influence of transition metal oxides on the kinetics of Li2O2 oxidation in Li-O2 batteries: high activity of chromium oxides. Phys. Chem. Chem. Phys. 16 (6), 2297–2304. doi:10.1039/c3cp53330a
Yao, W. T., Yuan, Y. F., Tan, G. Q., Liu, C., Cheng, M., Yurkiv, V., et al. (2019). Tuning Li2O2 formation routes by facet engineering of MnO2 cathode catalysts. J. Am. Chem. Soc. 141 (32), 12832–12838. doi:10.1021/jacs.9b05992
Zhai, T., Xie, S. L., Yu, M. H., Fang, P. P., Liang, C. L., Lu, X. H., et al. (2014). Oxygen vacancies enhancing capacitive properties of MnO2 nanorods for wearable asymmetric supercapacitors. Nano Energy 8, 255–263. doi:10.1016/j.nanoen.2014.06.013
Zhang, X. H., Chen, C. G., Chen, X., Wang, L. D. Y., Huang, T., and Yu, A. S. (2019). Ruthenium oxide modified alpha-manganese dioxide nanotube as efficient bifunctional cathode catalysts for lithium oxygen batteries. Chemistryselect 4 (25), 7455–7462. doi:10.1002/slct.201901744
Zhang, X. Z., Han, D., He, Y. B., Zhai, D. Y., Liu, D. Q., Du, H. D., et al. (2016). Mesoporous Cr2O3 nanotubes as an efficient catalyst for Li-O2 batteries with low charge potential and enhanced cyclic performance. J. Mater. Chem. 4 (20), 7727–7735. doi:10.1039/c6ta00331a
Keywords: lithium-oxygen battery, MnO2-x, Cr2O3, Energy efficiency, charge voltage
Citation: Wei Z, Zhang Z, Ren Y and Zhao H (2021) A Novel Cr2O3/MnO2-x Electrode for Lithium-Oxygen Batteries with Low Charge Voltage and High Energy Efficiency. Front. Chem. 9:646218. doi: 10.3389/fchem.2021.646218
Received: 25 December 2020; Accepted: 07 January 2021;
Published: 01 February 2021.
Edited by:
Bin Huang, Guilin University of Technology, ChinaReviewed by:
Peng Tan, University of Science and Technology of China, ChinaCopyright © 2021 Wei, Zhang, Ren and Zhao. This is an open-access article distributed under the terms of the Creative Commons Attribution License (CC BY). The use, distribution or reproduction in other forums is permitted, provided the original author(s) and the copyright owner(s) are credited and that the original publication in this journal is cited, in accordance with accepted academic practice. No use, distribution or reproduction is permitted which does not comply with these terms.
*Correspondence: Zhaohuan Wei, emh3ZWlAdWVzdGMuZWR1LmNu; Yaqi Ren, cmVueWFxaWlAMTYzLmNvbQ==
Disclaimer: All claims expressed in this article are solely those of the authors and do not necessarily represent those of their affiliated organizations, or those of the publisher, the editors and the reviewers. Any product that may be evaluated in this article or claim that may be made by its manufacturer is not guaranteed or endorsed by the publisher.
Research integrity at Frontiers
Learn more about the work of our research integrity team to safeguard the quality of each article we publish.