- 1International Center for Materials Nanoarchitectonics (WPI-MANA), National Institute for Materials Science (NIMS), Tsukuba, Japan
- 2Department of Chemistry, College of Humanities and Sciences, Nihon University, Tokyo, Japan
Thin-film growth is a platform technique that allows the preparation of various undeveloped materials and enables the development of novel energy generation devices. Preferred phase formation, control of crystalline orientation and quality, defect concentration, and stoichiometry in thin films are important for obtaining thin films exhibiting desired physical and chemical properties. In particular, the control of crystalline phase formation by utilizing thin-film technology favors the preparation of undeveloped materials. In this study, thin-film growth of transition metal nitride and rare-earth metal boride was performed using remote plasma–assisted molecular beam epitaxy and hybrid physical–chemical vapor deposition techniques, and was successfully achieved by tuning the competition between thermodynamics and kinetics during vapor-phase thin-film growth. Growth conditions of high crystalline quality titanium nitride thin films and high phase purity ytterbium boride thin films were not thermodynamically favorable. Appropriate control of the contribution degree of thermodynamics and kinetics during vapor-phase thin-film growth is crucial for fabricating high phase purity and high crystalline quality thin films.
Introduction
Thin-film technology is actively used in various fundamental research, industries, and applications, and is instrumental in providing a possible solution for preparing high-quality samples of undeveloped materials such as groups of metal borides, nitrides, and carbides, and for preparing nanostructured materials (Nishinaga and Kuech, 2015). In particular, this is a fundamental technology for fabricating novel energy generation devices, including thermoelectric energy-harvesting devices. Thin-film growth under kinetically favorable growth conditions enables the formation of metastable phases, hetero-interfaces, and nanocomposite phases. In contrast, highly crystalline thin films with low defect concentrations tend to grow under thermodynamically favorable growth conditions (Dhanaraji et al., 2010; Nishinaga, 2015). Phase formation, crystalline orientation and quality, defect concentration, surface morphology, and stoichiometry in thin films are influenced by thin-film growth parameters and greatly influence their properties. Control of competing thermodynamics and kinetics during vapor-phase thin-film growth is thus necessary to obtain thin films that exhibit the required physical and chemical properties. This can be implemented by precisely tuning thin-film growth parameters. In fact, the importance of the control of competing thermodynamics and kinetics has been pointed out for thin-film growth. The in-plane and out-of-plane crystalline orientations of ZnO layers on sapphire (0001) substrates were varied by the growth temperature and the growth rate (Ohkubo et al., 1999; Ohkubo et al., 2000). The ordering degrees of Ni and Mn ions in the double perovskite La2NiMnO6 thin films were changed by the growth temperature and partial oxygen pressure. A highly ordered phase of Ni and Mn ions can be obtained at a growth temperature of approximately 700°C. Promoting the disordering of Ni and Mn ions in La2NiMnO6 thin films has been reported above 700°C (Kitamura et al., 2009).
Among the various vapor-phase thin-film growth techniques, molecular beam epitaxy (MBE) and chemical vapor deposition (CVD) are attractive because of their low-kinetic-energy growth techniques which have the potential to generate high-quality thin films with lower defect concentrations than those in films fabricated by sputtering or pulsed laser deposition techniques (Nishinaga and Kuech, 2015; Nishinaga, 2015). In addition, the control of competing thermodynamics and kinetics can be precisely realized during thin-film growth and allows the formation of the required crystalline phase in thin films. These thin-film growth techniques are used for thin-film preparation of metal nitrides, borides, and carbides for use in various energy device applications, including the preparation of thermoelectric thin films. Crystalline phase formation control using thin-film technology is effectively applied to the preparation of undeveloped materials such as nitrides, borides, and carbides. Recently, theoretical predictions by density functional theory calculations and experimental studies have suggested that layered transition metal nitrides (Ohkubo and Mori, 2014a; Ohkubo and Mori, 2014b; Ohkubo and Mori, 2015a; Ohkubo and Mori, 2015b; Orabi et al., 2015; Ohkubo and Mori, 2016), alkaline-earth metal borides (Tynell et al., 2016), and rare-earth metal borides (Guélou et al., 2018) are potential attractive thermoelectric materials.
Thin-film growth of metal nitrides, borides, and carbides composed of a transition metal and/or a rare-earth metal is associated with difficulties in terms of supplying the source material. The low vapor pressures of elemental source materials such as boron, carbon, transition metals, and rare-earth metals make it difficult to stably supply these source elements for thin-film formations, which crucially limits the growth rates and film thickness. The flux instability of these source elements is well known and generates non-stoichiometry in thin films. Few solutions exist to solve this problem. Metal–organic molecules (Ohkubo et al., 2021) and boron hydrides (Nakamura, 1984; Tynell et al., 2016; Guélou et al., 2018) are promising source materials because the vapor pressures of most of these sources are sufficiently high for thin-film preparations. The low reactivity of nitrogen gas is also a critical issue in nitride thin-film growth owing to the suppression of the formation of the nitride phase. This can be resolved by using reactive gas species of ammonia gas or nitrogen plasma sources. Control of thin-film growth can be achieved by tuning the parameters of thin-film growth temperatures and supply rates of these source materials, which are parameters related to thermodynamics and kinetics, respectively. In this study, detailed analyses of thin-film growth conditions for titanium nitride and ytterbium boride were conducted to reveal the formation of high phase purity and high crystalline quality thin films under the growth conditions of proper contribution of thermodynamics and kinetics during vapor-phase thin-film growth.
Experimental Methods, Results, and Discussion
Molecular Beam Epitaxy of Titanium Nitride
Among the transition metal nitrides, titanium nitride (TiN) is a remarkably important compound because of its extensive use in various different types of applications, including energy-harvesting applications (Oyama, 1996; Kafizas et al., 2013; Ohkubo et al., 2021). In this study, TiN thin films were grown on (100)-oriented single-crystal magnesium oxide (MgO) substrates (Tateho Chemical) using an MBE apparatus (Eiko), as shown schematically in Figure 1. MgO is commonly used as a lattice-matched substrate for TiN epitaxial growth because of the small lattice mismatch of 0.68% between MgO (cubic rock salt crystal structure, a = 4.213 Å) (Swanson and Tatge, 1953) and TiN (cubic rock salt crystal structure, a = 4.242 Å) (Wong-Ng et al., 1987). The base pressure of the MBE apparatus was lower than 1.0 × 10–7 Pa. The thin-film growth temperature (substrate temperature) was varied between 600 and 800°C. Prior to thin-film growth, preheating of the MgO(100) substrate was conducted at 800°C for 60 min under ultrahigh-vacuum conditions to obtain a clean and flat surface. As shown in Figure 2A, after preheating at 800°C for 60 min, reflection high-energy electron diffraction (RHEED) images showed a change from a broad streaky pattern to a sharp streaky pattern. Ti was supplied using an electron-beam evaporator from a Ti (99.9%, Nilaco) pellet. The supply rates of Ti were 0.2–2.3 Å/s, which were measured using a quartz crystal microbalance thickness monitor (Inficon). Reactive nitrogen species were supplied by a radio frequency (RF) remote plasma source (300 W) with a constant nitrogen gas pressure between 3.0 × 10–3 and 7.0 × 10–3 Pa (0.5–1.8 sccm N2 gas flow) for nitridation reactions and the formation of TiN phase on MgO (100) substrates. Film growth was monitored in situ using RHEED. Film thickness was evaluated using a surface profiler (Veeco, Dektak 6M). The growth rates of the TiN layers were determined from the measured film thickness, which changed with the substrate temperature, supply rate of Ti, nitrogen plasma power, and the N2 gas flow rate.
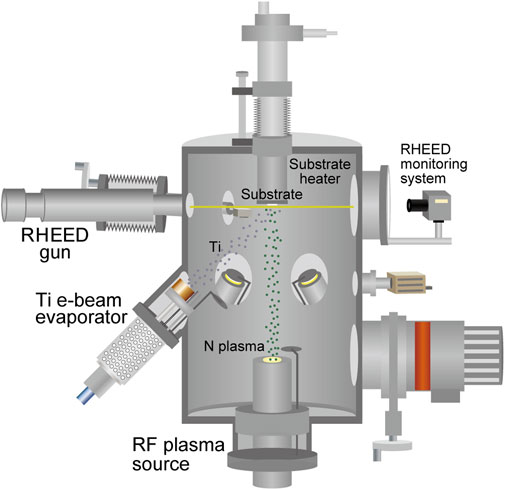
FIGURE 1. Schematic of the molecular beam epitaxy (MBE) apparatus for the thin-film growth of titanium nitrides.
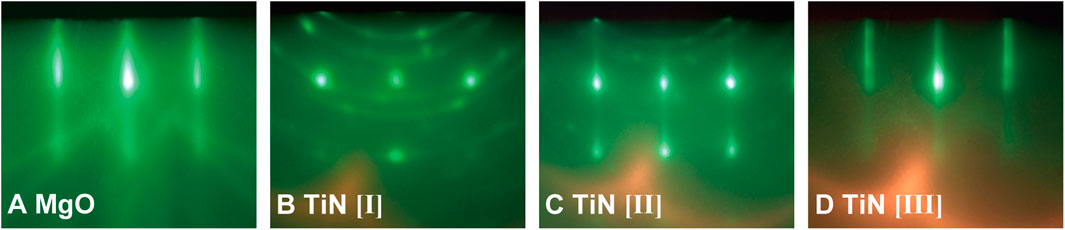
FIGURE 2. In situ RHEED images of (100)-oriented MgO substrates and TiN thin films along [010] azimuth. (A) MgO(100) substrate prior to film growth without nitrogen plasma. (B) TiN thin film grown at a substrate temperature of 800°C and a growth rate of 0.27 Å/s. (C) TiN thin film grown at 600°C and 0.92 Å/s. (D) TiN thin film grown at 800°C and 0.94 Å/s. All TiN layers were grown under reactive nitrogen species supply from RF plasma source operated by RF power of 300 W, 1.4 sccm N2 gas flow (partial nitrogen pressure of 6.0 × 10–3 Pa).
The RHEED patterns during TiN thin-film growth under various growth conditions are depicted in Figure 2; they varied greatly with the growth conditions. The crystalline orientation of TiN is sensitive to growth conditions. Under the condition of a growth rate lower than 0.3 Å/s, RHEED patterns exhibited Debye–Scherrer rings and weak spots, indicating that randomly oriented polycrystalline phases were dominant, even when the growth temperature was high at 800°C (Figure 2B). In contrast, dominant transmission diffraction patterns with weak rings were observed under the conditions of a growth temperature lower than 700°C and a growth rate higher than 0.3 Å/s, as shown in Figure 2C. This pattern indicates a rough surface of the grown film. Both the high growth rate (greater than 0.4 Å/s) and high growth temperature (above 700°C) were necessary to observe streaky RHEED patterns of TiN layers, which did not contain spotty and ring patterns (Figure 2D). Phase-pure epitaxial TiN growth without polycrystalline phases was achieved under the conditions of appropriately high growth rates and high growth temperature.
We mapped the growth mode of hetero-epitaxial TiN thin films on (100)-oriented MgO single-crystal substrates as a function of both the growth rate and the substrate temperature (Figure 3). The growth mode was evaluated by in situ RHEED, as described in Figure 2. The growth rates in Figures 2, 3 were changed by changing the supply rates of Ti and the growth temperatures under constant supply conditions of reactive nitrogen species (RF power of 300 W, 1.4 sccm N2 gas flow). Three distinct growth regimes of two different polycrystalline growth modes (regions [I] and [II] in Figure 3) and a phase-pure epitaxial growth mode (region [III]) were identified. Optimal growth conditions to realize phase-pure TiN epitaxial thin films with high crystalline quality and low electrical resistance were achieved at the growth conditions in region [III], where the applied growth temperature and the growth rate were 700–800°C and 0.5–1.0 Å/s, respectively. Regardless of the growth temperature, polycrystalline TiN thin films with high electrical resistance were obtained at growth rates below 0.3 Å/s (region [I]). Under the condition of low growth temperature (600–700°C) and a growth rate range of 0.2–1.0 Å/s, polycrystalline thin films with low electrical resistance were prepared (region [II]). In regions [I] and [II], streaky RHEED patterns were observed during the initial stage of TiN thin-film growth. The streaky RHEED patterns could not be maintained and were gradually changed to ring and spot patterns, as depicted in Figures 2B,C. Finally, mixed phases composed of a polycrystalline layer and an epitaxial layer were formed in regions [I] and [II]. Unsuccessful TiN homo-epitaxial growth can be considered under these growth conditions.
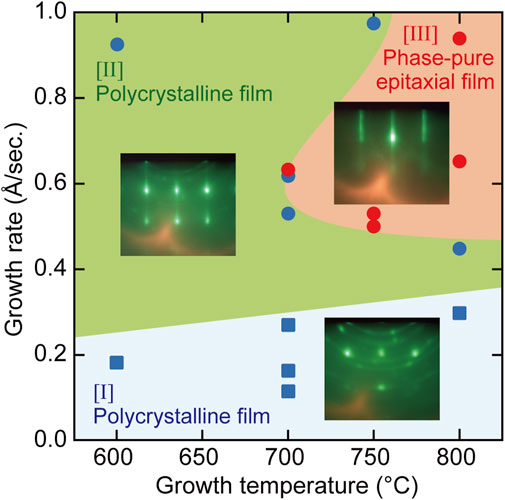
FIGURE 3. A map of TiN epitaxial growth modes depending on growth temperature and growth rate. Lines that represent borders between each region are guides for the eyes.
In general, thermodynamically favorable growth conditions are preferred to obtain a thin film with a highly aligned crystalline orientation and high crystalline quality. Low growth rate and high growth temperature are thermodynamically favorable growth conditions. However, appropriately high growth rates, greater than 0.5 Å/s, are necessary to achieve the phase-pure epitaxial TiN growth despite sufficiently high growth temperatures above 700°C (region [III]). This indicates that an appropriate epitaxial TiN growth occurs under the conditions of competing thermodynamics and kinetics. In the molecular beam epitaxy of TiN on MgO (100) substrates, the growth conditions with large thermodynamic contributions of the low growth rate and high growth temperature are located on the right-hand side of region [I] in Figure 3, where polycrystalline TiN thin films were obtained instead of phase-pure epitaxial TiN layers. In region [I], the Ti supply rate was considerably low, resulting in a large imbalance between the Ti and N supply rates. The influence of the cation/anion supply ratio on GaN MBE growth has been discussed in detail (Tarsa et al., 1997; Zywietz et al., 1998). Under nitrogen-rich growth conditions, abundantly absorbed nitrogen species on the surface tend to suppress Ga surface diffusion. Similarly, Ti surface diffusion may be suppressed by abundantly absorbed nitrogen species on the surface because of lower Ti supply rates, which could hamper the epitaxial growth of TiN even under thermodynamically favorable conditions in region [I]. The formation of phase-pure epitaxial TiN layers was achieved under the growth conditions of proper contribution of thermodynamics and kinetics. Kinetically favorable growth conditions of low growth temperature and a high growth rate are located in region [II] in Figure 3, in which polycrystalline TiN thin films were obtained. This is consistent with the crystal growth theory (Dhanaraji, G. et al., 2010; Nishinaga, 2015).
Ytterbium Boride Thin-Film Growth Via Hybrid Physical–Chemical Vapor Deposition
The thermoelectric properties of borides have attracted considerable attention in recent years (Aselage and Emin, 2003; Kim and Kimura, 2011; Gürsoy et al., 2015; Slack and Morgan, 2014; Mori, 2019; Mori, 2020). Recently, relatively good thermoelectric properties of YbBx thin films have been reported (Guélou et al., 2018). In addition, YbB6 has been disputed as a topological insulator that does not include the Kondo effect (Neupane et al., 2015; Kang et al., 2016; Gabani et al., 2020). Hybrid physical–chemical vapor deposition (HPCVD) was developed for the thin-film preparation of metal borides, as illustrated in Figure 4 (Tynell et al., 2016). To prepare ytterbium boride thin films, a boron hydride, decaborane (B10H14), was used as the boron source. A stable supply of boron elements from a solid boron source is rather difficult to be obtained because boron has a high melting point (2076°C) and low vapor pressure (Figure 5), and the boron melt is so reactive that it is difficult to hold it in any kind of crucible in an evaporator. The instability of supply source elements generates non-stoichiometry in thin films. On the contrary, as exhibited in Figure 5, boron hydrides are promising compounds as boron source materials for thin-film preparations owing to their high vapor pressure. A sufficiently high vapor pressure makes it possible to stably supply a boron source. However, lower boron hydrides, such as diborane (B2H6) and pentaborane (B5H9), are difficult to handle with because of their high reactivity, flammability, and toxicity. Among the boron hydrides, decaborane, which is a solid at room temperature with an adequate vapor pressure, is an appropriate compound for safe use as a source for thin-film preparations. Decaborane molecules (98%, Alfa Aesar) were supplied from a bottle and a gas line heated at approximately 390 K. Decaborane supply pressures were 1–3 × 10−3 Pa. Thermal sublimation of ytterbium is reasonably controllable because of its adequate vapor pressure (Daane and Habermann, 1964; Desideri et al., 1973); this was performed using a conventional effusion cell from an ytterbium ingot (99.9%, Sigma-Aldrich). Ytterbium boride thin films were prepared on (111)- and (100)-oriented MgO single-crystal substrates (Tateho Chemical) at a growth temperature (substrate temperature) range between 800 and 1,050°C, as listed in Table 1, for 30 min. Preheating of the MgO substrate was carried out at 1,000°C for 60 min prior to the thin-film growth. The substrate was heated using an electrical heating system, and the achievable temperature of the substrate was above 1,000°C. The ytterbium boride thin films were evaluated by X-ray diffraction (XRD, Rigaku SmartLab 3) and ex situ RHEED. The film thicknesses were characterized using a surface profiler (Veeco, Dektak 6M).
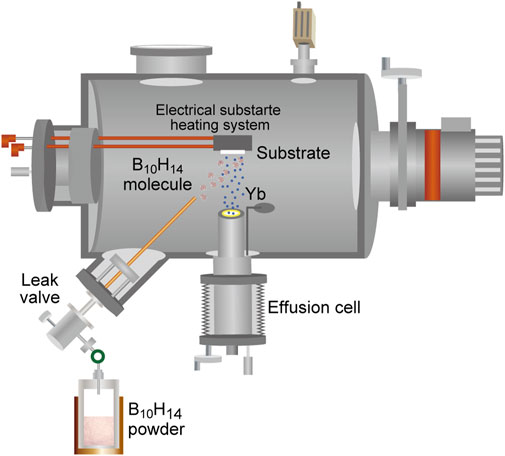
FIGURE 4. Schematic of hybrid physical–chemical vapor deposition (HPCVD) apparatus for ytterbium boride thin-film growth.
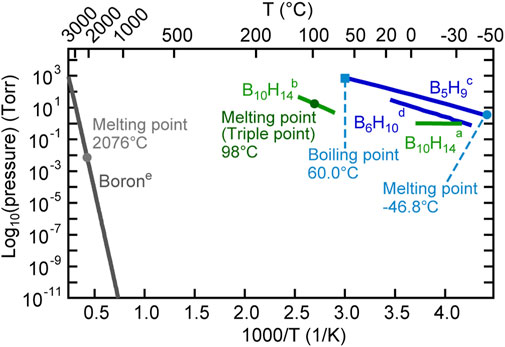
FIGURE 5. Vapor pressures of elemental boron and boron hydrides. a, b The vapor pressure data of B10H14 are taken from the literature reported by Furukawa and Park (1955) and Miller (1963). c The vapor pressure data of B5H9 are taken from the literature reported by Shapiro and Ditter (1957). d The vapor pressure data of B6H10 are taken from the literature reported by Gibbins and Shapiro (1959). e The vapor pressure data of boron are taken from the literature reported by Honig (1957).
Figure 6 shows the XRD and ex situ RHEED patterns of the grown films. Several compounds are known to exist between Yb and B: YbBx, x = 2, 4, 6, 12, and 66 (Massalski, 1986). In the grown films, YbB2 (sample a), YbB4 (samples b and c), and YbB6 (sample d) were identified from the characteristic XRD peak from 2θ = 20°–25°. As shown in the RHEED patterns, the grown films were polycrystalline with preferred orientations. However, the preferred orientation was not necessarily perpendicular to the film surface. Therefore, the peak intensities of the XRD patterns are not proportional to the film thickness. The YbB6 film grown on the MgO (100) substrate (sample d) spontaneously peeled off after cooling. The adhesion between ytterbium boride and MgO (100) is probably very weak. This is the reason why the RHEED pattern of sample d could not be obtained. When the growth temperature was increased, the boron content increased, and the Yb content decreased. The Yb vapor pressure in this temperature range is significantly high, 103–104 Pa (Daane and Habermann, 1964; Desideri et al., 1973); thus, Yb cannot stay on the growing surface for a long time as a metal and can be easily reevaporated. Indeed, the growth rate decreased with increasing growth temperature, as shown in Table 1, probably due to the enhancement of Yb reevaporation. Consequently, boron-rich ytterbium boride became the dominant phase with increasing growth temperature. The YbB2 phase was grown at 800°C (sample a). Above 900°C, YbB4 was detected by XRD measurements as the dominant phase in the thin films (samples b and c). The Yb chemical potential during the film growth determines the kinetics of the YbBx film growth. In fact, the YbB6 phase was grown instead of YbB4 under the conditions of low Yb supply rates (sample d). However, we have not yet obtained the YbB12 or YbB66 phases. It is likely that more thermodynamically favorable conditions are required to form complicated crystals of extremely boron-rich phases (Veremchuk et al., 2005; Sologub et al., 2017). The obtained growth rates of ytterbium boride thin films are obviously high: an order of magnitude higher than the typical thin-film growth rates of other inorganic materials. Even at high growth temperatures of 800–1,000°C, more kinetic growth conditions of a high growth rate are preferable to form the crystalline phases of ytterbium borides, probably because they lead to a reduction in the reevaporation effects of Yb at the thin film or substrate surfaces. Epitaxial stabilization effects can be expected under growth conditions with appropriate kinetic contribution (Gorbenko et al., 2002). The YbB2 crystal has a rather simple AlB2-type crystal structure (Veremchuk et al., 2005). In contrast, the crystal structures of YbB4 and YbB6 are more complicated and contain boron networks (Etourneau et al., 1979; Blomberg et al., 1995). Appropriate kinetic contributions might be necessary to crystallize YbB4 and YbB6 with complex crystal structures. A high growth rate has also been reported for the thin-film growth of SrB6 (Tynell et al., 2016). Appropriate control of competing thermodynamics and kinetics is necessary to obtain the crystalline phases of ytterbium borides, and this was realized by the growth conditions of a high growth rate and high growth temperature. The high growth rate of ytterbium borides was enabled by the use of boron hydride, decaborane, as a boron source, which has a sufficiently high vapor pressure. The supply of boron from a solid boron source crucially limits the achievement of a sufficient supply rate owing to its low vapor pressure. Recently, metal–organic molecular beam epitaxy of TiN has been reported (Ohkubo et al., 2021). The metal–organic source tetrakis dimethylamido titanium {TDMAT, Ti [N(CH3)2]4} was successfully used as a titanium source. The vapor pressure of TDMAT is also high enough for a stable supply of titanium. The use of metal–organic and boron hydride sources with sufficiently high vapor pressures can achieve stable source supplies and make it possible to control the competing thermodynamics and kinetics in vapor-phase thin-film growth.
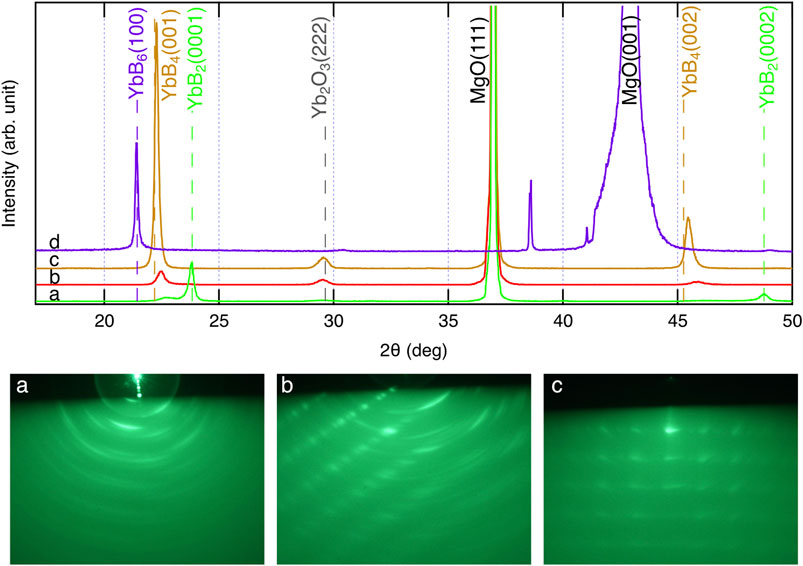
FIGURE 6. XRD (upper panel) and RHEED (lower panels) patterns of ytterbium boride thin films. The alphabets (a–d) in XRD and RHEED patterns correspond to the sample IDs listed in Table 1.
Conclusion
Details of titanium nitride and ytterbium boride thin-film growth via MBE and HPCVD methods are described. High crystalline quality epitaxial thin films of titanium nitride and high phase-pure ytterbium boride thin films were grown under the conditions of high growth temperatures and high growth rates. These growth conditions were not thermodynamically favorable conditions, even though the MBE and HPCVD methods are low kinetic energy growth techniques. The formation of high phase purity and high crystalline quality thin films were achieved under the growth conditions of proper contribution of thermodynamics and kinetics during vapor-phase thin-film growth. In many cases, phase-pure and high crystalline quality thin films are preferred to realize required physical and chemical properties. In particular, thermoelectric transport coefficients such as electrical conductivity (σ), Seebeck coefficient (S), and the thermoelectric power factor (S2σ) are greatly influenced by the crystalline phase purity, crystalline orientation, and quality. By tuning the growth parameters precisely, appropriate control of the contribution degree of thermodynamics and kinetics is crucial for fabricating thin films that exhibit the required properties.
Data Availability Statement
The original contributions presented in the study are included in the article/Supplementary Material; further inquiries can be directed to the corresponding author.
Author Contributions
IO and TA conceived the research; IO prepared titanium nitride epitaxial thin films by MBE and characterized titanium nitride thin films using a RHEED and a surface profiler. TA developed the HPCVD apparatus assisted by KN. TA prepared ytterbium boride thin films by HPCVD and evaluated ytterbium boride thin films by XRD. The film thicknesses of ytterbium boride thin films were characterized by IO. IO and TA wrote the manuscript; TM and KN helped to revise the manuscript. All the authors discussed and commented on the manuscript.
Funding
The authors acknowledge the support from the JST-Mirai Program (JPMJMI19A1) and JSPS KAKENHI (JP16H06441).
Conflict of Interest
The authors declare that the research was conducted in the absence of any commercial or financial relationships that could be construed as a potential conflict of interest.
Publisher’s Note
All claims expressed in this article are solely those of the authors and do not necessarily represent those of their affiliated organizations, or those of the publisher, the editors and the reviewers. Any product that may be evaluated in this article, or claim that may be made by its manufacturer, is not guaranteed or endorsed by the publisher.
References
Aselage, T. L., and Emin, D. (2003). “Boron Carbides: Unconventional High-Temperature Thermoelectrics,” in Chemistry, Physics and Materials Science of Thermoelectric Materials: Beyond Bismuth telluride. Editor M. G. Kanatzidis (Kluwer), 55–70. doi:10.1007/978-1-4419-9278-9
Blomberg, M. K., Merisalo, M. J., Korsukova, M. M., and Gurin, V. N. (1995). Single-crystal X-ray Diffraction Study of NdB6, EuB6 and YbB6. J. Alloys Compd. 217, 123–127. doi:10.1016/0925-8388(94)01313-7
Daane, A. H., and Habermann, C. E. (1964). Vapor Pressures of the Rare-Earth Metals. J. Chem. Phys. 41, 2818–2827. doi:10.1063/1.1726358
Desideri, A., Piacente, V., and Nobili, S. (1973). Vapor Pressure of α-samarium and α-ytterbium. J. Chem. Eng. Data 18, 140–141. doi:10.1021/je60057a004
Dhanaraji, G., Byrappa, K., Prasad, V., and Dudley, M. (2010). Springer Handbook of crystal Growth. Berlin, Heidelberg: Springer. doi:10.1007/978-3-540-74761-1
Etourneau, J., Mercurio, J. P., Berrada, A., Hagenmuller, P., Georges, R., Bourezg, R., et al. (1979). The Magnetic and Electrical Properties of Some Rare Earth Tetraborides. J. Less Common Met. 67, 531–539. doi:10.1016/0022-5088(79)90038-9
Furukawa, G. T., and Park, R. P. (1955). Heat Capacity, Heats of Fusion and Vaporization, and Vapor Pressure of Decaborane (B10H14). J. Res. Natl. Bur. Stan., 55, 255–260. doi:10.6028/jres.055.029
Gabani, S., Flachbart, K., Siemensmeyer, K., and Mori, T. (2020). Magnetism and Superconductivity of Rare Earth Borides. J. Alloys Compd. 821, 153201. doi:10.1016/j.jallcom.2019.153201
Gibbins, S. G., and Shapiro, I. (1959). Vapor Pressure, Mass, and Infrared Spectra of Hexaborane. J. Chem. Phys. 30, 1483–1485. doi:10.1063/1.1730222
Gorbenko, O. Y., Samoilenkov, S. V., Graboy, I. E., and Kaul, A. R. 2002). Epitaxial Stabilization of Oxides in Thin Films. Chem. Mater. 14, 4026–4043. doi:10.1021/cm021111v
Guélou, G., Martirossyan, M., Ogata, K., Ohkubo, I., Kakefuda, Y., Kawamoto, N., et al. (2018). Rapid Deposition and Thermoelectric Properties of Ytterbium Boride Thin Films Using Hybrid Physical Chemical Vapor Deposition. Materialia 1, 244–248. doi:10.1016/j.mtla.2018.06.003
Gürsoy, M., Takeda, M., and Albert, B. (2015). High-pressure Densified Solid Solutions of Alkaline Earth Hexaborides (Ca/Sr, Ca/Ba, Sr/Ba) and Their High-Temperature Thermoelectric Properties. J. Solid State. Chem. 221, 191–195. doi:10.1016/j.jssc.2014.10.001
Kafizas, A., Carmalt, C. J., and Parkin, I. P. (2013). CVD and Precursor Chemistry of Transition Metal Nitrides. Coord. Chem. Rev. 257, 2073–2119. doi:10.1016/j.ccr.2012.12.004
Kang, C.-J., Denlinger, J. D., Allen, J. W., Min, C.-H., Reinert, F., Kang, B. Y., et al. (2016). Electronic Structure of YbB6: Is it a Topological Insulator or Not? Phys. Rev. Lett. 116, 116401. doi:10.1103/PhysRevLett.116.116401
Kim, H., and Kimura, K. (2011). Vanadium Concentration Dependence of Thermoelectric Properties of β-rhombohedral boron Prepared by Spark Plasma Sintering. Mater. Trans. 52, 41–48. doi:10.2320/matertrans.m2010272
Kitamura, M., Ohkubo, I., Kubota, M., Matsumoto, Y., Koinuma, H., and Oshima, M. (2009). Ferromagnetic Properties of Epitaxial La2NiMnO6 Thin Films Grown by Pulsed Laser Deposition. Appl. Phys. Lett. 94, 132506. doi:10.1063/1.3111436
Massalski, T. B. (1986). “B-Yb (boron-ytterbium),” in Binary alloy Phase Diagrams. ASM Int. Mater. Park 1, 395–396.
Miller, G. A. (1963). The Vapor Pressure of Solid Decaborane. J. Phys. Chem. 67, 1363–1364. doi:10.1021/j100800a507
Mori, T. (2020). “Rare Earth Higher Borides,” in Handbook on the Physics and Chemistry of Rare-Earths. Editors. J. -C. Bunzli, and V. Pecharsky (Elsevier) Vol. 58, pp. 39–154. doi:10.1016/bs.hpcre.2020.10.002
Mori, T. (2019). Thermoelectric and Magnetic Properties of Rare Earth Borides: Boron Cluster and Layered Compounds. J. Solid State. Chem. 275, 70–82. doi:10.1016/j.jssc.2019.03.046
Nakamura, K. (1984). Preparation and Properties of Amorphous boron Films Deposited by Pyrolysis of Decaborane in the Molecular Flow Region. J. Electrochem. Soc. 131, 2691–2697. doi:10.1149/1.2115385
Neupane, M., Xu, S.-Y., Alidoust, N., Bian, G., Kim, D. J., Liu, C., et al. (2015). Non-Kondo-like Electronic Structure in the Correlated Rare-Earth Hexaboride YbB6. Phys. Rev. Lett. 114, 16403. doi:10.1103/PhysRevLett.114.016403
Nishinaga, T. (2015). Handbook of crystal Growth, Fundamentals. Second Edition, Vol. I. Amsterdam: Elsevier. doi:10.1016/C2011-0-04376-4
Nishinaga, T., and Kuech, T. F. (2015). Handbook of crystal Growth, Thin Films and Epitaxy. Second Edition, Vol. III. Amsterdam: Elsevier. doi:10.1016/C2013-0-09792-7
Ohkubo, I., Hou, Z., Lee, J. N., Aizawa, T., Lippmaa, M., Chikyow, T., et al. (2021). Realization of Closed-Loop Optimization of Epitaxial Titanium Nitride Thin-Film Growth via Machine Learning. Mater. Today Phys. 16, 100296. doi:10.1016/j.mtphys.2020.100296
Ohkubo, I., Matsumoto, Y., Ohtomo, A., Ohnishi, T., Tsukazaki, A., Lippmaa, M., et al. (2000). Investigation of ZnO/sapphire Interface and Formation of ZnO Nanocrystalline by Laser MBE. Appl. Surf. Sci. 159-160, 514–519. doi:10.1016/s0169-4332(00)00138-0
Ohkubo, I., and Mori, T. (2014a). Two-dimensional Layered Complex Nitrides as a New Class of Thermoelectric Materials. Chem. Mater. 26, 2532–2536. doi:10.1021/cm403840e
Ohkubo, I., and Mori, T. (2014b). Three-Dimensionality of Electronic Structures and Thermoelectric Transport in SrZrN2 and SrHfN2 Layered Complex Metal Nitrides. Inorg. Chem. 53, 8979–8984. doi:10.1021/ic500902q
Ohkubo, I., and Mori, T. (2015a). Origin of Projected Excellent Thermoelectric Transport Properties in d0-Electron AMN2 (A = Sr or Ba; M = Ti, Zr, Hf) Layered Complex Metal Nitrides. Eur. J. Inorg. Chem. 2015, 3715–3722. doi:10.1002/ejic.201500350
Ohkubo, I., and Mori, T. (2015b). Anisotropic Anomalies of Thermoelectric Transport Properties and Electronic Structures in Layered Complex Nitrides AMN2 (A = Na, Cu; M = Ta, Nb). Chem. Mater. 27, 7265–7275. doi:10.1021/acs.chemmater.5b02015
Ohkubo, I., and Mori, T. (2016). Anisotropic Thermoelectric Properties in Layered Complex Nitrides with α-NaFeO2-type Structure. APL Mater. 4, 104808. doi:10.1063/1.4955399
Ohkubo, I., Ohtomo, A., Ohnishi, T., Matsumoto, Y., Koinuma, H., and Kawasaki, M. (1999). In-plane and Polar Orientations of ZnO Thin Films Grown on Atomically Flat Sapphire. Surf. Sci. 443, L1043–L1048. doi:10.1016/S0039-6028(99)01024-9
Orabi, R. A. R. A., Orisakwe, E., Wee, D., Fontaine, B., Gautier, R., Halet, J. F., et al. (2015). Prediction of High Thermoelectric Potential in AMN2 Layered Nitrides: Electronic Structure, Phonons, and Anharmonic Effects. J. Mater. Chem. A. 3, 9945–9954. doi:10.1039/c5ta00546a
Oyama, S. T. (1996). The Chemistry of Transition Metal Carbides and Nitrides. Dordrecht: Springer. doi:10.1007/978-94-009-1565-7
Slack, G. A., and Morgan, K. E. (2014). Some Crystallography, Chemistry, Physics, and Thermodynamics of B12O2, B12P2, B12As2, and Related Alpha-boron Type Crystals. J. Phys. Chem. Sol. 75, 1054–1074. doi:10.1016/j.jpcs.2013.12.021
Sologub, O., Salamakha, L. P., Stöger, B., Rogl, P. F., Mori, T., Eguchi, G., et al. (2017). On the boron Rich Phases in the Yb-B System. J. Solid State Chem. 255, 172–177. doi:10.1016/j.jssc.2017.08.007
Swanson, H. E., and Tatge, E. (1953). Standard X-ray Diffraction Powder Patterns. Natl. Bur. Stand. Circular 539, 53–54.
Tarsa, E. J., Heying, B., Wu, X. H., Fini, P., DenBaars, S. P., and Speck, J. S. (1997). Homoepitaxial Growth of GaN under Ga-stable and N-Stable Conditions by Plasma-Assisted Molecular Beam Epitaxy. J. Appl. Phys. 82, 5472–5479. doi:10.1063/1.365575
Tynell, T., Aizawa, T., Ohkubo, I., Nakamura, K., and Mori, T. (2016). Deposition of Thermoelectric Strontium Hexaboride Thin Films by a Low Pressure CVD Method. J. Cryst. Growth 449, 10–14. doi:10.1016/j.jcrysgro.2016.05.030
Veremchuk, I. V., Chaban, N. F., Babizhetskyy, V. S., Pilyushchak, O. T., and Kuz’ma, Y. B. (2005). 1070-K Section of the Yb-Fe-B Phase Diagram. Inorg. Mater. 41, 700–705. doi:10.1007/s10789-005-0194-x
Wong-Ng, W., McMurdie, H. F., Paretzkin, B., Zhang, Y., Davis, K. L., Hubbard, C. R., et al. (1987). Standard X-ray Diffraction Powder Patterns of Sixteen Ceramic Phases. Powder Diffr. 2, 191–202. doi:10.1017/S0885715600012690
Keywords: thin-film growth, molecular beam epitaxy, chemical vapor deposition, nitrides, borides
Citation: Ohkubo I, Aizawa T, Nakamura K and Mori T (2021) Control of Competing Thermodynamics and Kinetics in Vapor Phase Thin-Film Growth of Nitrides and Borides. Front. Chem. 9:642388. doi: 10.3389/fchem.2021.642388
Received: 16 December 2020; Accepted: 22 June 2021;
Published: 27 July 2021.
Edited by:
Qing Peng, King Fahd University of Petroleum and Minerals, Saudi ArabiaCopyright © 2021 Ohkubo, Aizawa, Nakamura and Mori. This is an open-access article distributed under the terms of the Creative Commons Attribution License (CC BY). The use, distribution or reproduction in other forums is permitted, provided the original author(s) and the copyright owner(s) are credited and that the original publication in this journal is cited, in accordance with accepted academic practice. No use, distribution or reproduction is permitted which does not comply with these terms.
*Correspondence: Isao Ohkubo, T0hLVUJPLklzYW9Abmltcy5nby5qcA==