- 1Dipartimento di Chimica e Tecnologie Chimiche, Università Della Calabria, Arcavacata di Rende, Italy
- 2Dipartimento di Scienze Chimiche, Biologiche, Farmaceutiche Ed Ambientali, Università di Messina, Messina, Italy
A speciation study on the interaction between Ca2+ and ligands of biological interest in aqueous solution is reported. The ligands under study are l-cysteine (Cys), d-penicillamine (PSH), reduced glutathione (GSH), and oxidized glutathione (GSSG). From the elaboration of the potentiometric experimental data the most likely speciation patterns obtained are characterized by only protonated species with a 1:1 metal to ligand ratio. In detail, two species, CaLH2 and CaLH, for systems containing Cys, PSH, and GSH, and five species, CaLH5, CaLH4, CaLH3, CaLH2, and CaLH, for system containing GSSG, were observed. The potentiometric titrations were performed at different temperatures (15 ≤ t/°C ≤ 37, at I = 0.15 mol L−1). The enthalpy and entropy change values were calculated for all systems, and the dependence of the formation constants of the complex species on the temperature was evaluated. 1H NMR spectroscopy, MALDI mass spectrometry, and tandem mass spectrometry (MS/MS) investigations on Ca2+-ligand solutions were also employed, confirming the interactions and underlining characteristic complexing behaviors of Cys, PSH, GSH, and GSSG toward Ca2+. The results of the analysis of 1H NMR experimental data are in full agreement with potentiometric ones in terms of speciation models and stability constants of the species. MALDI mass spectrometry and tandem mass spectrometry (MS/MS) analyses confirm the formation of Ca2+-L complex species and elucidate the mechanism of interaction. On the basis of speciation models, simulations of species formation under conditions of some biological fluids were reported. The sequestering ability of Cys, PSH, GSH, and GSSG toward Ca2+ was evaluated under different conditions of pH and temperature and under physiological condition.
Introduction
Calcium is the fifth most important element in the human body. It is indispensable for life, for the regulation of metabolism and maintenance of structure (Peterlik and Stoeppler, 2004). It behaves like an intracellular “second messenger” in numerous processes, namely, neurotransmitter release, cellular proliferation and differentiation, and control of exocrine and endocrine secretions (Bringhurst and Potts., 1979; Broaudus, 1993). In human body, about 99% of total calcium (1.0–1.3 kg in adults) (Hluchan and Pomerantz, 2002) is found in the bones. The remaining part, 1%, is present in intra- and extracellular fluids. Free calcium concentration in the cell ranges between 10−6 and 10−8 mol kg−1. It is about 10−3 mol kg−1 in the sarcoplasm (Frausto da Silva and Williams, 2001a). The mean Ca2+ concentration in the plasma is 2.5 mmol L−1, of which about 50% is present as free ion; the remaining part is bound for 40% to plasma proteins and for 10% to citrate and phosphate. The rigid control of free calcium in the plasma is very crucial, as even small concentration changes can cause significant variations in the skeletal site, as well as intracellular free calcium, with harmful consequences for bone health (Peterlik and Stoeppler, 2004; Whedon, 1980). Calcium homeostasis is based on a dynamic equilibrium of its fluxes between three different body compartments, namely, extracellular fluid, intracellular one, and skeletal tissue. As regards the physiological role of calcium, it includes the control of many kinase reactions in metabolism, of dioxygen release in photosynthesis, and of dehydrogenases in oxidative phosphorylation (Frausto da Silva and Williams, 2001b). Ca2+ interacts preferably with oxygen donor groups. In the body fluids it can bind polymers, such as proteins, via carboxylate and phosphate sidechains. In the proteins, the main donor groups toward Ca2+ are represented by carboxylate and carbonyl centers (Frausto da Silva et al., 2001a).
Cys is one of the most important binding agents for metal cations in biological fluids (Laurie et al., 1979). Its concentration in normal human plasma is in the micromolar range (Brigham et al., 1960). The drug penicillamine, which has a very similar structure to Cys, was commonly employed in the treatment of Wilson’s disease (Walshe, 1956; Jones, 1991). GSH is a tripeptide consisting of the amino acids l-glutamic acid (Glu), Cys, and glycine (Gly). It exists in two forms: a reduced (GSH) and an oxidized one, i.e., dimer glutathione disulfide (GSSG) (Labib et al., 2016). GSH is ubiquitous antioxidant present in cells as well as in bacteria (Sies, 1999; Pompella et al., 2003; Kretzschmar et al., 2020; Meister and Anderson, 1983). In mammalian cells, concentrations greater than 12 mmol L−1 are reported (Dringen, 2000). Both GSH and its oxidized form, GSSG, are fundamental for the maintenance of the intracellular redox state (Shahid et al., 2020). They are considered biomarkers of oxidative stress in biological fluids as well as for the diagnosis of certain clinical disorders (Labib et al., 2016; Olmos Moya et al., 2017). The mechanism of antioxidant cellular defense in vivo is governed by GSH, oxidized continuously to disulfide glutathione (GSSG) (Davis and Hanumegowda, 2008). In healthy cells, the GSH form constitutes over 90% of glutathione (Labib et al., 2016). In addition to protecting cells from oxidative damage, GSH is involved in the complexation and transport reactions of metal ions (Olmos Moya et al., 2017). In blood, the normal values of GSH and GSSG are 3.8–5.5 and 0.2–0.5 μmol L−1, respectively (Labib et al., 2016).
Given all these aspects, reliable assessment of the speciation of biologically relevant ligands with Ca2+ is crucial to understand and to model the behavior of these systems. Ligands under study are reported in Figure 1. In this study, the experimental measurements were performed by different techniques: potentiometry, 1H NMR spectroscopy, MALDI mass spectrometry, and MS/MS. The potentiometric titrations were carried out at different temperatures, 15 ≤ t/°C ≤ 37 and I = 0.15 mol L−1 in NaCl. Some simulations of species formation under conditions of biological fluids were reported. The sequestering ability of all ligands understudy toward Ca2+ was evaluated under different conditions of pH and temperature.
Material and Methods
Materials
The solutions containing calcium metal cation were obtained by weighing and dissolving the corresponding salt, calcium (II) chloride dihydrate (purity >99%, Fluka/Honeywell, Charlotte, North Carolina, US). Afterward calcium solutions were standardized by titration with EDTA (Ethylenediaminetetraacetic acid disodium salt, BioUltra, ≥99%, Sigma-Aldrich/Merck, Darmstadt, Germany) standard solution. Ligand solutions were prepared by weighing and dissolving, without further purification, the following products: l-cysteine (purity ≥99.5%, Fluka/Honeywell, Charlotte, North Carolina, US), d-penicillamine (purity ≥97%, Alfa-Aesar/Thermo Fisher, Kandel, Germany), reduced glutathione (purity ≥98%, Alfa-Aesar, Thermo Fisher, Kandel, Germany), and oxidized glutathione (purity 98%, Sigma-Aldrich/Merck, Darmstadt, Germany). The purity of the ligands was checked by alkalimetric titration. It was found to be greater than 99%. Solutions of hydrochloric acid and sodium hydroxide were obtained by dilution of Fluka (Fluka/Honeywell, Charlotte, North Carolina, US) ampoules and afterward they were standardized with sodium carbonate (≥99.5%, Sigma-Aldrich/Merck, Darmstadt, Germany) and potassium biphthalate (≥99.5%, Sigma-Aldrich/Merck, Darmstadt, Germany), respectively. Both salts were previously dried in an oven at 110 °C. Solutions of sodium hydroxide were reprepared very frequently and were kept in bottles with soda lime traps. Solutions of sodium chloride were obtained by weighing the corresponding salt (puriss., Sigma-Aldrich/Merck, Darmstadt, Germany), previously dried in an oven at 110 °C. Distilled water (conductivity <0.1 μS cm−1) and grade A glassware were employed for the preparation of all the solutions.
Potentiometric Apparatus and Procedure
Two distinct systems were employed for the potentiometric titrations. In detail, the systems consist in an identical configuration consisting in an automatic dispenser Metrohm Dosino 800, a Metrohm model 809 Titrando potentiometer, and a Metrohm LL-Unitrode WOC combined glass electrode. Each potentiometric system was connected to a PC and the experimental titration data were acquired by the Metrohm TIAMO 2.2 software. It can control several parameters, such as e.m.f. stability, titrant delivery, and data acquisition. Estimated accuracy of this apparatus is ±0.15 mV and ±0.002 ml for e.m.f. and for readings of titrant volume, respectively.
Each titration consists in additions of volumes of NaOH standard to 25 ml of the solution containing Ca2+, ligand, and a supporting electrolyte (NaCl). Experimental details on potentiometric titrations are reported in Table 1. Glass jacket thermostated cells were employed for the measurements performed under different conditions of temperature (15 ≤ t/°C ≤ 37), by bubbling pure N2 in order to avoid CO2 and O2 inside the solutions and under magnetic stirring. For each measurement, an independent titration of HCl with standard NaOH was performed to calculate the standard electrode potential E0 and the pKw value, under the same experimental ionic strength and temperature conditions.
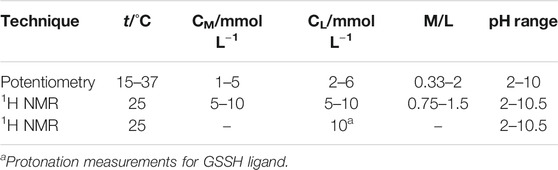
TABLE 1. Experimental conditions for potentiometric and 1H NMR titrations at I = 0.15 mol L−1 in NaCl.
NMR Apparatus and Procedure
The spectrometer employed for the collection of 1H NMR spectra is a Varian 500 F T-NMR. 1,4-Dioxane was used as internal reference (δCHdioxane = 3.70 ppm); the chemical shifts are referred to tetramethylsilane (TMS). All the measurements were carried out in a 9:1 H2O/D2O solution at t = 25 °C. Presaturation technique was employed to suppress the water signal. Experimental details on 1H NMR titrations are reported in Table 1.
Mass Spectrometric Apparatus and Procedure
A water solution of 2 equivalents of each ligand (Cys, PSH, GSH, GSSG) was added dropwise to 1 mmol of CaCl2 dissolved in water with magnetic stirring for 2 h at room temperature. MALDI MS and MS/MS analyses were performed using a 5800 MALDI-TOF-TOF Analyzer (AB SCIEX) in reflection positive ion mode with a mass accuracy of 5 ppm. At least 5000 laser shots were typically accumulated with a laser pulse rate of 400 Hz and 1000 Hz in the MS and MS/MS mode, respectively. MS/MS experiments were performed using ambient air as collision gas with a medium pressure of 10−6 Torr and a collision energy of 1 kV, with a mass accuracy of 20 ppm. After acquisition, spectra were processed using Data Explorer version 4.0. MALDI MS and MS/MS experimental conditions were optimized using sinapinic acid (SA, 5 mg/ml in H2O/CH3CN 40:60, v/v; with 0.1% TFA) as matrix for all ligands. The sample loading was performed by dried droplet method for all ligands, spotting 1 μL of sample/matrix premixed solution (1:5, v/v ratio).
Calculations
Experimental data of potentiometric titrations were processed using BSTAC and STACO programs. They allow for obtaining the best speciation model for each system under study, the formation constant values of the species, and the parameters of a titration (standard potential E0, analytical concentration of the reagents, and junction potential). The parameters for the dependence of complex formation constants on temperature were obtained by LIANA program. More details on software employed in the refinement of the experimental data are reported in De Stefano et al. (1997). For 1H-NMR titrations, HypNMR software was employed to obtain protonation and formation constant values, as well as the individual chemical shift of each species, using the observed signals and assuming fast mutual exchange in the NMR time scale (Frassineti et al., 1995). HySS program was used to obtain the speciation diagrams and the formation percentages of the complex species (Alderighi et al., 1999).
Results and Discussion
In the calculations, protonation constants of ligands understudy (Cardiano et al., 2008; Crea et al., 2008; Cardiano et al., 2013) and hydrolytic constant of Ca2+ were taken into account. They are reported in Supplementary Tables S1 and S2.
Potentiometric measurements were carried out under different conditions of temperature and metal-ligand ratios, to choose the most appropriate speciation model and to be able to refine the formation constants of the species in solution. The formation constants of Ca2+(M)-ligand(L) species are expressed as overall formation constants (β) and stepwise formation constants (K). The reactions are the following (charges are omitted for simplicity):
Within the speciation studies, the most reliable model for a metal-ligand system is chosen by taking into account several factors, such as the simplicity of the model itself, the statistical parameters (standard and mean deviation on the fit), the variance ratio between the chosen model and others, and the formation percentages of the formed species (Filella, 2005).
Speciation Profiles and Aqueous Behavior
Formation constant values of Ca2+-Cys, PSH, GSH, GSSG species obtained via potentiometric measurements at different temperatures and I = 0.15 mol L−1 were reported in Table 2. The speciation pattern for all the systems includes only 1:1 M:L species. Cys, PSH, and GSH show a very similar behavior with the same speciation model including only two significant species, namely, MLH2 and MLH. For all three systems, the stability of complex species in terms of stepwise formation constants is between a minimum of 1.57 (MLH species for Ca2+-GSH system, t = 25 °C) and a maximum of 3.66 (MLH2 species for Ca2+-PSH system, t = 37 °C). In Figure 2A the speciation diagram of Ca2+-Cys species is depicted at I = 0.15 mol L−1 and t = 15, 37 °C. Under physiological conditions (t = 37 °C, I = 0.15 mol L−1), MLH2 species is formed in the range 2 ≤ pH ≤ 9 and reaches a metal fraction of 0.4 in the range 3 ≤ pH ≤ 7. The main complex species in the range 8 ≤ pH ≤ 10 is MLH with a maximum metal fraction corresponding to 0.3 at pH = 9.5.
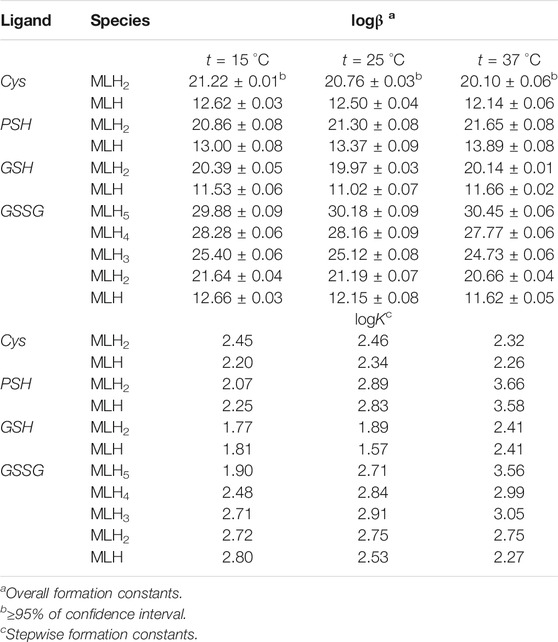
TABLE 2. Formation constants of Ca2+-Cys, PSH, GSH, GSSG species at different temperatures at I = 0.15 mol L−1 in NaCl.
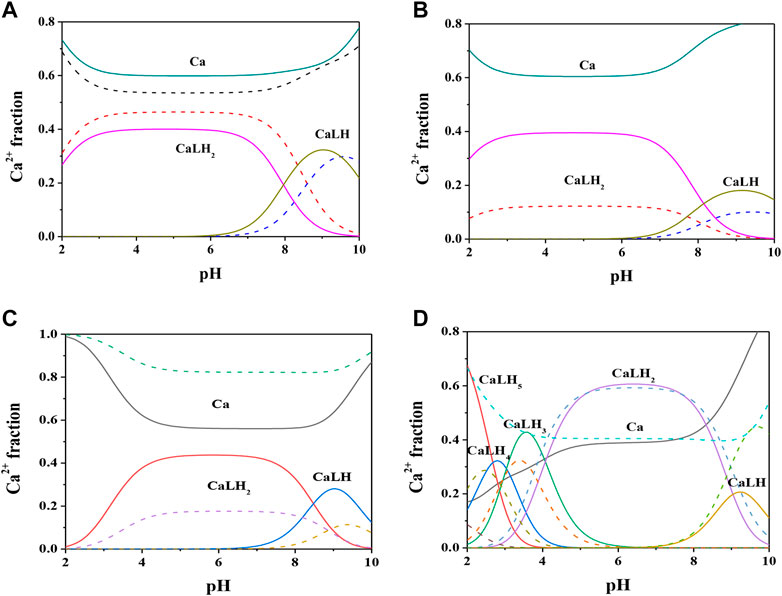
FIGURE 2. Speciation diagrams of Ca2+-ligand (L) systems at t = 15 °C (dotted lines) and t = 37 °C (solid lines), CM = 2 mmol L−1, CL = 4 mmol L−1, I = 0.15 mol L−1 in NaCl (A) L = Cys(B) L = PSH(C) L = GSH(D) L = GSSG.
Formation constants of Ca2+-PSH species are quite higher with respect to Ca2+-Cys ones. For example, stepwise formation constant values at t = 37 °C resulted between 3.58 and 3.66. The differences between stepwise formation constants of species formed by PSH and Cys with Ca2+, under physiological conditions, are ΔlogK = 1.3 for both MLH2 and MLH. The speciation diagram, represented in Figure 2B, refers to Ca2+-PSH system, under physiological conditions. MLH2 species is formed in the wide interval 2 ≤ pH ≤ 8, reaching a maximum metal fraction of 0.4; MLH species is present in the range 8 ≤ pH ≤ 10 with a lower metal fraction (0.2).
For Ca2+-GSH system, stepwise formation constant values at t = 37 °C are equal to 2.41 for both species. The differences between formation constants of Ca2+-PSH and -GSH species, under physiological conditions are ΔlogK = 1.2 for both. The speciation diagram, depicted in Figure 2C, refers to Ca2+-GSH system, under physiological conditions. It shows that MLH2 is the main complex species in the wide interval 2 ≤ pH ≤ 9, with a maximum metal fraction of 0.4, and MLH predominates in the range 8.5 ≤ pH ≤ 10, with a metal fraction of 0.3.
A separate discussion must be made for the system containing GSSG. As expected from the presence of the numerous protonable groups on molecule, the speciation model is very rich in complex species, namely, MLH5, MLH4, MLH3, MLH2, and MLH. Their stability is comparable to the values found for the other ligands already discussed. As an example, at t = 37 °C and I = 0.15 mol L−1, logK values, referring to stepwise formation constants, range between 2.27 and 3.56 for the five species. Speciation profile for Ca2+-GSSG system is represented in Figure 2D. Under physiological conditions, the less significant species is MLH one, while the most significant species is MLH2, which predominates in the pH range between 4.5 and 9 reaching metal fraction of 0.6. The most protonated species, MLH5, MLH4, and MLH3, predominant at pH < 4.5, reach maximum metal fractions equal to 0.65, 0.3, and 0.45, respectively. MLH species is significant only at pH > 9, with a metal fraction of 0.2.
1H NMR Spectroscopy
The interaction of ligands of biological interest with metal cations in aqueous solution had been already studied by our research group with several spectroscopic techniques, such as 1H NMR (Cardiano et al., 2008; Cardiano et al., 2011; Cardiano et al., 2013; Cardiano et al., 2016), UV-Vis (Falcone et al., 2011; De Stefano et al., 2014), Mössbauer (Cardiano et al., 2006), and Raman (Cassone et al., 2019). In the literature, there are some recent papers that report 1H NMR investigations on GSSH and PSH with metal cations other than Ca2+ (Sisombath et al., 2014; Kretzschmar et al., 2020). More in detail, Sisombath et al. report a complexation study on Pb2+ with PSH by 1H NMR analysis, in D2O solutions at pH = 9.6, at various M:L molar ratios. The data here reported are comparable with that reference and specifically it is possible to underline the same trend relative to the significant chemical shift of CH-2 (Δδ = 0.6 ppm) and of only one of the two -CH3.
In this paper 1H NMR spectra of Ca2+-Cys species, reported in Figure 3 at different pH values and t = 25 °C, show a chemical shift of the signals related to the proton in 2 and to the two protons in 3, indicated as H-2, Ha-3, and Hb-3, respectively for Cys. At pH < 8 there is a triplet for H-2 and a double doublet (dd) for Ha-3 and Hb-3. At pH > 8, the complexity of the signals increases wherein a multiplet for H-2, a dd for Ha-3, and a dd for Hb-3 are shown. The chemical shift of the H-2 proton to the increase of pH is approximately 0.8 ppm upfield due to the increase in the negative charge for the deprotonation of the carboxyl group and subsequently of the thiol group. A similar trend is evident for protons in 3; in this case the chemical shift is about 0.3 ppm. Much more interesting is the splitting of the signals into two different dd, which can be interpreted with greater rigidity of the ligand for the presence of a dianion or for the interaction with the metal cation as well. This AMX system is therefore due to the different magnetic properties of the two protons in 3 and consequent more complex coupling between the three protons H-2, Ha-3, and Hb-3. The interaction with Ca2+ is evident from the comparison with the corresponding chemical shift values of Cys alone, under the same experimental conditions.
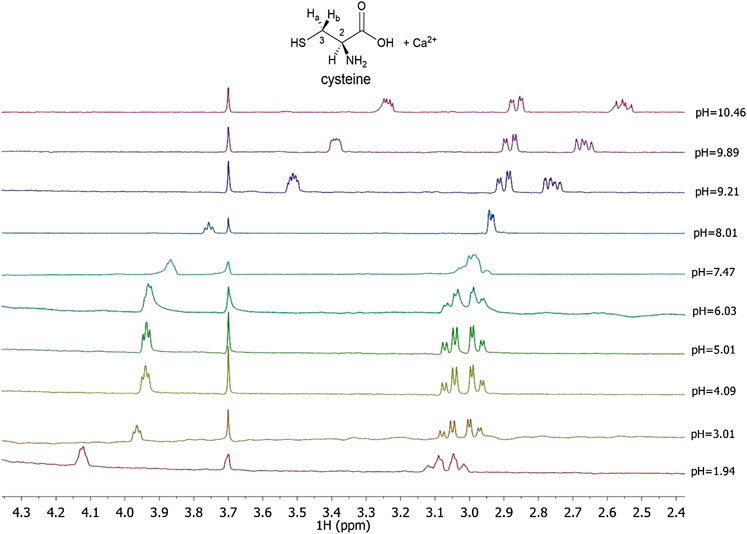
FIGURE 3. 1H NMR spectra on solutions containing Ca2+ (M) and Cys(L) at CM = 7.5 mmol L−1, CL = 10 mmol L−1, t = 25 °C, I = 0.15 mol L−1 in NaCl, 1.94 ≤ pH ≤ 10.46.
1H NMR spectra of Ca2+-PSH, Ca2+-GSH solutions were reported in Supplementary Figures S2-S3. Both ligands evidenced a similar behavior to Cys, as their spectra NMR showed a significant shift in signals at the change of pH. The interaction of each ligand with Ca2+ is highlighted by the comparison with the corresponding chemical shift values of the ligand in the absence of the metal cation under the same experimental conditions. The comparison of the formation constant values obtained via potentiometric and 1H NMR measurements (see Table 3) shows satisfactory correspondence.
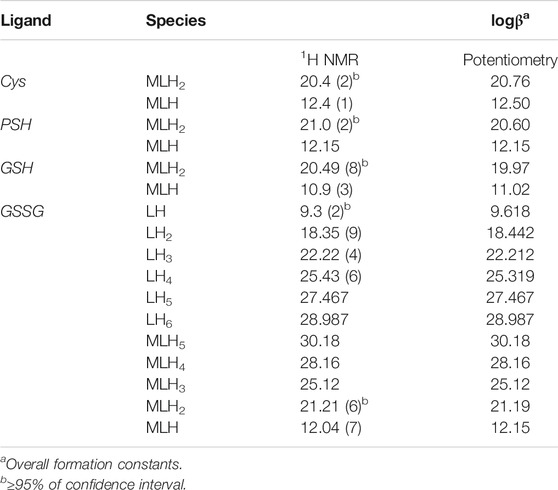
TABLE 3. Comparison between the experimental formation constants of Ca2+− ligand species and protonation constants of GSSG obtained via1H NMR and potentiometry at t = 25 °C and I = 0.15 mol L−1.
Here the NMR analysis of the free GSSG ligand at different pH values is reported. 1H NMR spectra of GSSG in 10% D2O/H2O solution show only six signals due to the symmetry to the S-S bond. Table 3 shows the comparison between the protonation constant values obtained by potentiometric and 1H NMR measurements. It was possible to obtain the values relating to the first four protonation constants (LH, LH2, LH3, and LH4), while those relating to the LH5 and LH6 species were kept constant using the values obtained by potentiometry. The agreement among the results obtained by the two different techniques was excellent. 1H NMR spectra registered on Ca2+-GSSG solutions at t = 25 °C and I = 0.15 mol L−1, represented in Figure 4, show substantially the same signal pattern observed in the spectra relating to the solutions containing GSSG ligand (Supplementary Figure S4). In detail, at pH = 2.2 are present amide protons 4 and 13 at δ = 8.5 ppm (2 singlets), proton in 3 at 3.95 ppm (quartet), proton in 11 at δ = 3.85 ppm (multiplet), proton in 14 at δ = 3.22 ppm (multiplet), proton in 2 at δ = 2.93 ppm (dd), proton in 9 at δ = 2.50 ppm (multiplet), and proton in 10 at δ = 2.13 ppm (multiplet).
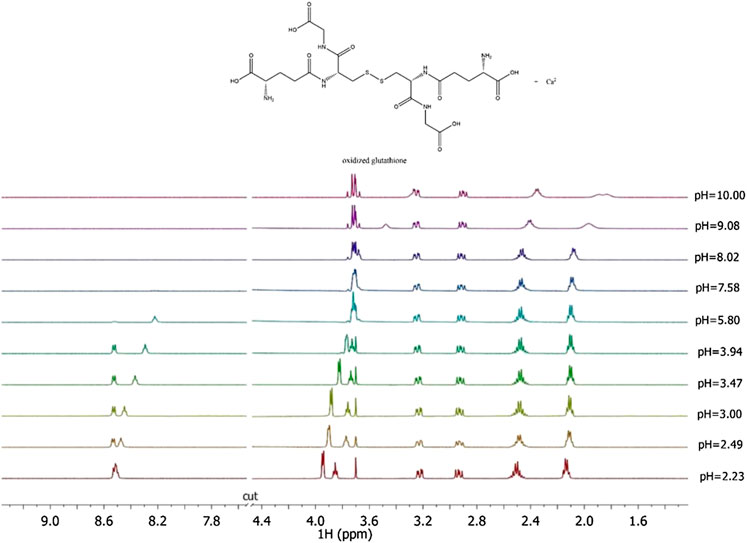
FIGURE 4. 1H NMR spectra on solutions containing Ca2+ (M) and GSSG(L) at CM = 8 mmol L−1, CL = 6 mmol L−1, t = 25 °C, I = 0.15 mol L−1 in NaCl, 2.23 ≤ pH ≤ 10.00.
The chemical shift values of the individual species were calculated on the basis of formation percentages of each species in solution. These chemical shifts, reported in Supplementary Table S3, were used to determine the values of the formation constants of the complex species. In Table 3 these formation constant values obtained by 1H NMR titrations were reported, together with potentiometric ones. It is possible to notice a good agreement among the values determined by the two different techniques. For Ca2+-GSSG species only the values referring to MLH2 and MLH species were refined, keeping constant ones obtained by potentiometry related to MLH5, MLH4, and MLH3 species. The speciation model considered for all the systems is also confirmed by the complete overlap of the experimental and calculated chemical shift values shown in Figure 5. It should be noted that, at pH > 8, 1H NMR spectra on the solutions containing ligands in the presence of Ca2+ show significant differences with respect to the corresponding free ligands. At pH > 8, differences of Δδ between 0.05 and 0.10 ppm were calculated on average for all ligands, except for GSSG. From this experimental evidence, it can be assumed that Cys, PSH, GSH, and GSSG could behave as divalent ligands, binding Ca2+ and giving rise to cyclic complexes.
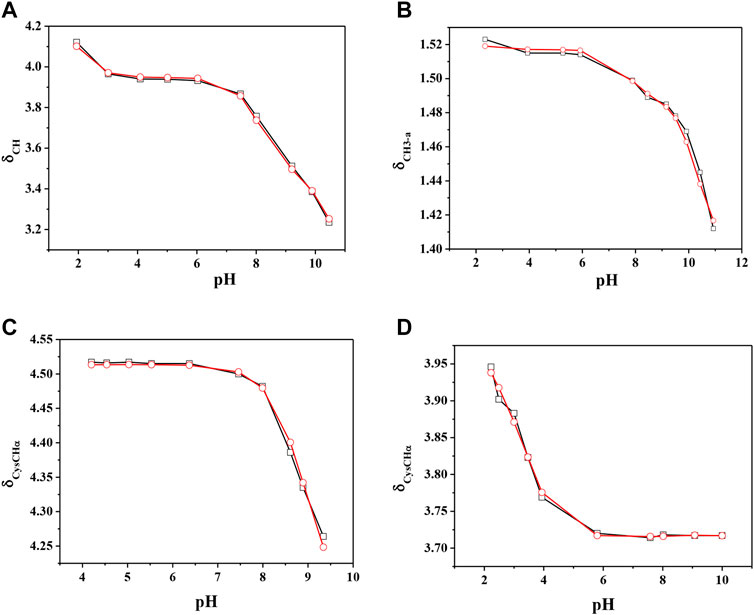
FIGURE 5. Experimental (□) and calculated (O) chemical shift values (in ppm) vs. pH of (A) CH on Ca2+(M)-Cys(L) solutions, (B) CH3-a on Ca2+(M)-PSH(L) solutions, (C) CysCHα on Ca2+(M)-GSH(L) solutions, (C) CysCHα on Ca2+(M)-GSSG(L) solutions.
MALDI MS and MS/MS
Mass spectrometry combined with soft ionization methods as electrospray ionization (ESI) and matrix assisted laser desorption ionization (MALDI) is currently becoming a strategic approach to clarify structures and coordination sites in compounds where metals are chelated by biological ligands (Cardiano et al., 2009; Furia et al., 2014; Aiello et al., 2017; Aiello et al., 2018a; Chillè et al., 2020). MALDI-TOF/TOF-MS platforms can be used for the highly sensitive analysis of low molecular weight compounds (Aiello et al., 2020a) in complex matrices (Aiello et al., 2018b; Aiello et al., 2020b; Imbrogno et al., 2019; Salvatore et al., 2020). In order to investigate whether calcium binding by Cys, PSH, GSH, and GSSG induces formation of complexes, a water solution of 2 equivalents of each ligand was added dropwise to 1 equivalent of CaCl2 and complex association was analyzed by MALDI MS using sinapinic acid as matrix. Signals corresponding to complex ML with 1:1 stoichiometry are the most intense signals in the spectrum for all investigated systems. The molecular masses derived from these measurements are in good agreement with the calculated mass (within 5 ppm, Table 4). The simplest systems, represented by Ca2+-Cys and Ca2+-PSH, will briefly be discussed. Both ligands hold multiple donor sites that are capable of intramolecular stabilization of the metal-ligand species. The carboxylic acids, bearing donor groups in their α or β positions, generally act as bidentate ligands giving rise to cyclic structures (Aiello et al., 2018a; Falcone et al., 2013). Accordingly, the formation of [MLH]+ species suggests that Cys and PSH act as bidentate ligands giving rise to six-membered cycles (Figure 6). The simplicity of the MS/MS spectra suggests that only few fragmentation pathways are allowed for the decomposition of complexes. MALDI MS/MS spectrum of the system Ca2+-Cys (Figure 6A) reveals that the main fragmentation pathways of the precursor [MLH]+ (m/z 159.97, [CaC3H6NSO2]+) consist in the loss of low molecular species such as NH2 (m/z 144.96 [CaC3H5SO2]+) and CH2NH (m/z 130.95 ([CaC2H3SO2]+). However, some characteristic fragment ions can be found and correlate with the proposed structure. In particular, the formation of the ions of m/z 130.95 ([CaC2H3SO2]+), m/z 118.95 ([CaC2H7SO]+), and m/z 90.95 ([CaH3SO]+) arises from across ring fragmentation of a six-membered structure. Analogously, PSH leads to a cyclic structure ([MLH]+ of m/z 188.01 ([CaC5H10NO2S]+). Several distinguishing ion products were detected in the MS/MS spectra; all the peak assignments are described in Table 4 and Figure 6B. In agreement with the NMR data, it can be reasonably stated that Cys and PSH act as divalent ligands and that they bind the Ca2+ ion through O and S giving rise to six-membered cyclic complexes, as already observed for other ligands containing carboxylic and thiol groups (Cardiano et al., 2009).
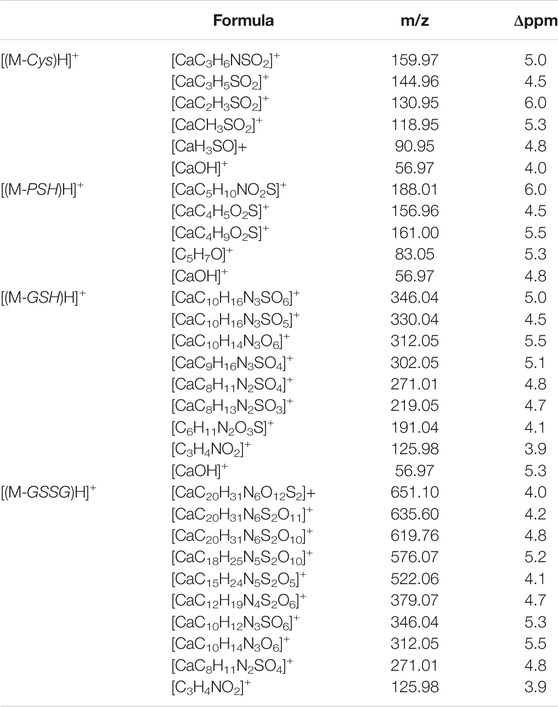
TABLE 4. Mass spectrometry data of Ca2+-L species, reported as m/z values, formula assignments, and MS/MS values for fragment ions.
GSH is a tripeptide bearing two free -COOH groups, a -NH2 group, and a -SH group; it provides a hydrophilic interface and a handle for further reactivity with other functional molecules as well as metal ions. The metal coordination ability of GSH is well documented, highlighting its multichelating nature. The speciation of both reduced and oxidized forms of GSH in MS/MS condition was considered. Information about molecular mass of the Ca2+-GSH as well as Ca2+-GSSG complex is easily obtained using 1:1 Ca2+-GSH molar ratios. The peak at m/z 346.04 corresponds to the ion [MLH]+ in which GSH is deprotonated (i.e., GSH2-) and therefore presumably bound to Ca2+via -COOH and -NH amino groups. The calcium complex of GSH (m/z 346.04 [CaC10H16N3SO6]+) decomposes to give, besides major H2O and CO2 and H2S losses, small abundances of w3b*, a3*, b2*, c2*, b1*, and d2a* calcium containing and z1 non-calcium product ions (Figure 7A). Product ions, which contain the C terminus, are formed by losses of residues comprised of only one amino acid, suggesting that the primary binding site for the Ca2+ is the N terminus of the peptide. The formation of the ion of m/z 125.98 ([C3H4CaNO2]+) and its counterpart m/z 219.05 [C8H13N2O3S]+ indicates that Glu is calcium-binding amino acid. The Ca2+-GSSG (m/z 651.10 [C20H31CaN6O12S2]+) complex decomposes giving a remarkably simple spectrum; it breaks down releasing Glu (m/z 522.06 [CaC15H24N5S2O5]+), Gly (m/z 576.07 [CaC18H26N5S2O10]+), and OH (m/z 635.60 [CaC20H31N6S2O11]+) as neutrals. The further formation of the most informative calcium containing products of m/z 619.76, m/z 346.04, and m/z 379.07 (Figure 7B) is also observed. The breakage of CH2-S and S-S bonds leads to the formation of the ions of m/z 379 and 346, respectively. Thereafter, both calcium containing species decompose giving rise to low intensity ion series. Appearance of small mass calcium containing ions, in MS/MS spectrum of Ca-GSSG peptide complex, is additional evidence that calcium binding is via N terminus of the peptide. Therefore, GSSG involves calcium in an “open” type complex, in which the metal ion is not coordinated from both glutamic acids, assuming a behavior like a simple amino acid. Finally, the simplicity of MS/MS spectra indicates that the binding of Ca2+ ions to GSH and GSSG is to the deprotonated glutamyl carboxylic residue and to the NH amino function. Ca2+-peptide complexes undergo fragmentations that are determined by the location of the Ca binding site.
Speciation in Biological Fluids
In order to evaluate the relevance of the systems under study under real conditions, two biological fluids were considered. The first application consists in the evaluation of formation percentages of Ca2+ complex species, by considering plasma concentration, temperature, and ionic strength conditions (t = 37 °C, I = 0.15 mol L−1, CCa = 2.5 mmol L−1, CCys = 0.01 mmol L−1; CGSH = 5.5 μmol L−1, CGSSG = 0.5 μmol L−1, CCl = 0.1037 mol L−1, CSO4 = 0.49 mmol L−1, CCO3 = 24.9 mmol L−1, CPO4 = 1.6 mmol L−1) (Lentner, 1983). In these conditions, at pH = 7.4 the main species is CaPO4, with a percentage of 60.9%. The most important species among ones under study are CaCysH2 and CaCysH, although their sum just reaches 10.3%, as shown in Figure 8A.
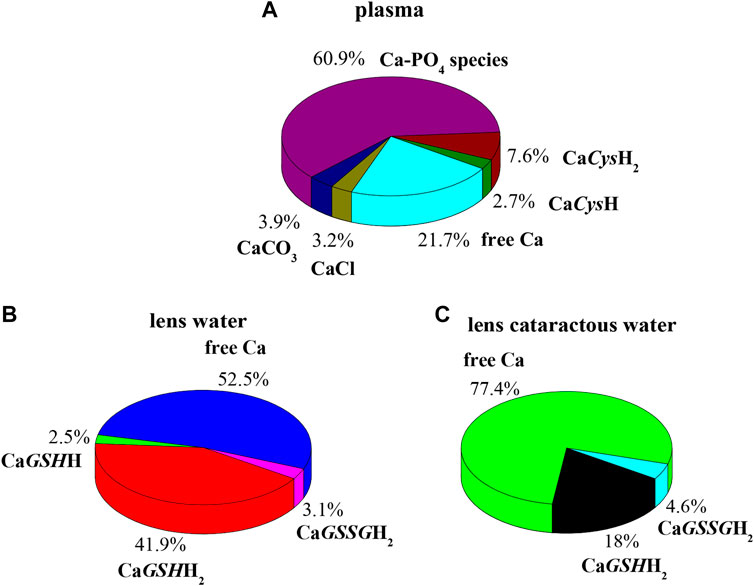
FIGURE 8. Ca-Cys, GSH, GSSG species in biological fluids at t = 37 °C, I = 0.15 mol L−1. (A) Plasma conditions (pH = 7.4); (B) lens water conditions (pH = 7.2); (C) lens cataractous water conditions (pH = 7.2).
The second application is based on lens aqueous solution. In the human eye the aqueous humor is located between the lens and the cornea. It is a gelatinous fluid where antioxidants, such as GSH and Cys, were investigated widely, since they serve as markers for eye diseases and infections. In the lens, the antioxidant GSH and ascorbic acid have unusually high concentration (Pescosolido et al., 2016). The functions performed by GSH with ascorbic acid in the lens are manifold. Among them, very important is the protection of protein thiol groups against oxidation agents and the detoxification of hydrophobic species in reactions catalyzed by glutathione S-transferase enzymes. In cataractous lens as well as in the aging lens, calcium concentration increases, and destruction of ascorbic acid and reduction of GSH content also occur (Chandorkar et al., 1980; Pescosolido et al., 2016). Accordingly, two different simulations were performed considering the composition of electrolyte and biological ligands in normal and in cataractous lens water. The obtained results are very different. Figure 8B represents the pie plot of Ca2+ complex species at pH = 7.2, by considering normal lens water concentrations (CCa = 0.01 mmol L−1, CCys = 0.0143 mmol L−1; CGSH = 3.28 mmol L−1, CGSSG = 0.095 mmol L−1, CCl = 0.79 mmol L−1, CAscorbic Acid = 1 mmol L−1) (Chandorkar et al., 1980; Königsberger et al., 2015). In this case, among species formed by Ca2+-ligands under study, those containing GSH form with higher percentages with a sum of 44.4%. The results significantly change by considering concentrations in cataractous lens water. Several studies reported that the level of reduced GSH in the lens decreases with the development of cataract (Kisic et al., 2012; Pescosolido et al., 2016). In this way over the years, GSH content reduces up to 73%, and GSSG content levels increase up to 18% (Pescosolido et al., 2016). Accordingly, the pie plot at pH = 7.2, under cataractous lens water conditions, was depicted in Figure 8C (CCa = 0.12 mmol L−1, CCys = 0.0143 mmol L−1; CGSH = 0.9 mmol L−1, CGSSG = 0.11 mmol L−1, CCl = 0.43 mmol L−1) (Chandorkar et al., 1980; Kisic et al., 2012; Königsberger et al., 2015; Pescosolido et al., 2016). In this case, percentage of CaGSHH2 species drastically decreases while remaining significant (from 41.9 to 18%); CaGSSGH2 increases slightly while resulting in an irrelevant species. These simulations confirm the need of knowledge of reliable formation constants at different conditions to predict the relevance of the species in real systems.
Dependence of Formation Constants on the Temperature
Formation constant values of the complex species reported in Table 2, obtained by potentiometric measurements at t = 15, 25, 37 °C, were analyzed for the determination of the formation enthalpy changes of the species, via the van't Hoff equation, already employed for several other systems (Cardiano et al., 2019; Cordaro et al., 2019; Foti and Giuffre, 2020; Giuffrè et al., 2019; Giuffrè et al., 2020):
where logβT is the formation constant at a specific ionic strength and temperature (expressed in Kelvin), logβθ is the formation constant at T = 298.15 K, and ΔH0 is the formation enthalpy change at T = 298.15 K in kJ mol−1, R = 8.314,472 J K−1 mol−1.
The values of formation enthalpy changes of all the species of Ca2+-Cys, -PSH, -GSH, and -GSSG systems are collected in Table 5, together with entropy and free energy values. They are also shown as bar plot in Figure 9, to better highlight the contribution to the formation free energy of the enthalpy and entropy thermodynamic parameters. Since the interactions between Ca2+ and the ligands understudy are mainly of electrostatic nature, it is expected that the entropic term gives the highest contribution to the free energy change, due to the orientation disorder given by the solvation water molecules. This was found for most species (except for MLH one formed by the interaction with Cys ligand, MLH2, and MLH ones containing GSSG ligand).
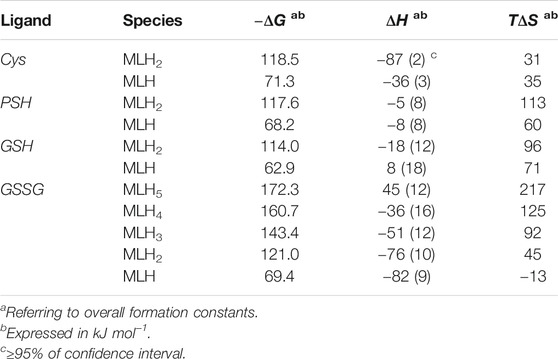
TABLE 5. Thermodynamic formation parameters of Ca2+-Cys, -PSH, -GSH, -GSSG species at t = 25 °C, I = 0.15 mol L−1 in NaCl.
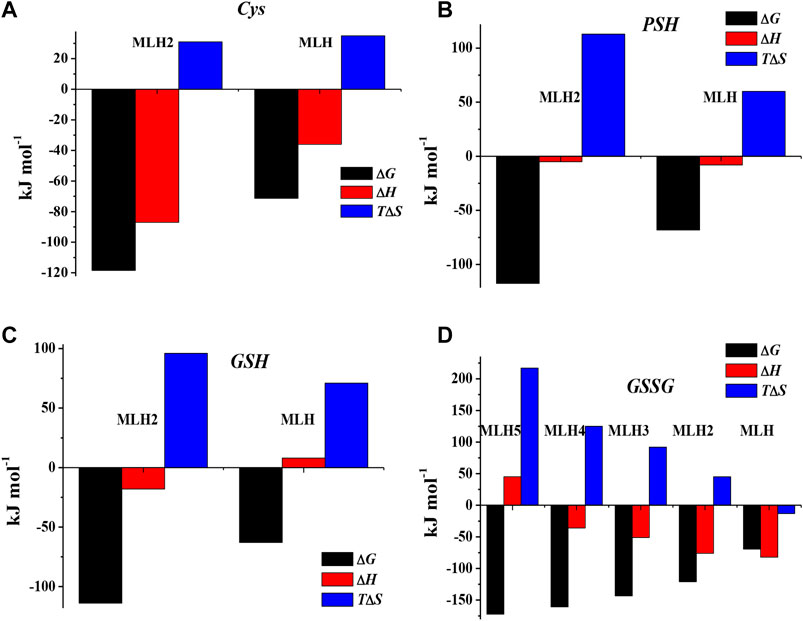
FIGURE 9. Bar plot of ΔG, ΔH, and TΔS referring to Ca2+-Cys(A), Ca2+-PSH(B), Ca2+-GSH(C), and Ca2+-GSSG(D) species at t = 25 °C, I = 0.15 mol L−1 in NaCl, according to overall formation reaction.
Sequestering Ability
The sequestering capacity represents the tendency, in solution, of a ligand to complex metal cation forming metal-ligand species, which allow for reducing the concentration of the free metal cation in solution. The stability of the complex species formed in solution influences the concentration of the free metal ion. The higher the stability of the formed species, the lower the concentration of the free cation. Considering the whole pH range, different metal-ligand species are formed in solution; each of them contributes to the sequestration of the metal cation. In order to describe the sequestering capacity of a given ligand with respect to a metal cation, it is not enough to know the formation constant values and the formation percentages of the different metal-ligand species. It is necessary to consider that different metal-ligand systems, having formation constants different from each other, can show the same formation percentages at a given pH and vice versa. Furthermore, all the equilibria in which the ligand and the metal ion under study take part must be considered, namely, ligand protonation, metal ion hydrolysis reactions, and weak interactions with the background salt. For these reasons, an empirical parameter, pL0.5, was proposed, which represents the cologarithm of the ligand concentration necessary to sequester 50% of the metal cation present in traces. The traces are precisely the concentration conditions with which many metal cations are present in natural fluids. To evaluate for quantitative purposes the sequestering capacity of a ligand with respect to a metal cation, the following Boltzmann-type equation with asymptotes 0 for pL→ 0 and 1 for pL→∞ was used (Gianguzza et al., 2012; Falcone et al., 2013; De Stefano et al., 2016):
where χ is the sum of the molar fractions of the metal-ligand species and pL is the cologarithm of the total ligand concentration. This parameter depends on system conditions, such as temperature, pH, and ionic strength.
In order to evaluate the sequestering capacity of Cys, PSH, GSH, and GSSG ligands toward Ca2+, pL0.5 values at different pH and temperatures were calculated. The results obtained are reported in Supplementary Table S5. Figure 10 illustrates the sequestering capacity of Cys, PSH, GSH, and GSSG ligands toward Ca2+ under physiological conditions (pH = 7.4, t = 37 °C, I = 0.15 mol L−1). As can be seen, the sequestering capacities of the ligands toward Ca2+ under physiological conditions follow the order:
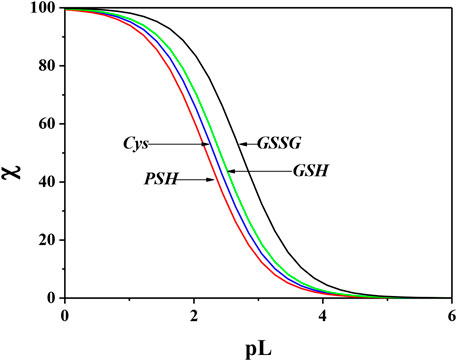
FIGURE 10. Comparison of sequestering ability of Cys, PSH, GSH, and GSSG toward Ca2+ under physiological conditions (pH = 7.4, t = 37 °C, I = 0.15 mol L−1).
By comparing these data with those relating to the stepwise formation constants of Ca2+-ligand species, obtained by potentiometric measurements under physiological conditions, it is possible to find a different order of stability for the MLH2 species:
and a further different order for the MLH species:
This underlines the importance of calculating the sequestering ability that, taking into account all the interactions, can be different with respect to the order of stability assessed for a single species and reveal the “real” trend of the ligands.
Literature Comparisons
In literature databases there are few thermodynamic data on interactions of ligands under study with Ca2+ (Martell et al., 2004; May and Murray, 2001; Pettit and Powell, 2001). As regards Ca2+-Cys system, a paper reports at t = 25 °C and I = 0.1 mol L−1 logβ = 1.92 for ML species and several ternary species with other ligands (Ramamoorthy and Manning, 1975). This only value cannot be compared with the results with this paper, since the speciation model is totally different. In the case of Ca2+-GSH system, a speciation model at t = 37 °C and I = 0.15 mol L−1 with four species, namely, MLH2, MLH, ML, and MLOH, with logβ = 20.68, 12.89, 3.84, -6.46, respectively, is reported (Touche and Williams, 1976). These values can be compared with ours, as regards the common species, i.e., MLH2 and MLH, in the same experimental conditions (logβ = 20.14, 11.66, respectively). The significant differences probably can be attributed to the different speciation model considered. In a paper of Singh, where formation constant values of GSH with several metal cations, namely, Ca2+, Mg2+, Cu2+, Pb2+, Ni2+, Zn2+, Co2+, Cd2+, and Mn2+, are reported, only one formation constant value referred to ML species was obtained for each system, including one containing Ca2+ (Singh et al., 2001). For this reason, this formation constant value cannot be compared with results here reported.
In a more recent paper, a fairly similar speciation model with three species was found, namely, MLH2, MLH, and ML, where logβ = 19.27, 11.08, 1.60, respectively (t = 25 °C, I = 0.15 mol L−1) (Cigala et al., 2012). In this paper, the values obtained under the same conditions for MLH2 and ML species are logβ = 20.39, 11.53, respectively. The agreement in this case, mainly for MLH species, is quite satisfactory.
Conclusion
The main purpose of this study was obtaining consistent speciation models and reliable thermodynamic data referring to Ca2+-bioligands systems, based on the results gained via different analytical techniques. Speciation models and stability formation constants obtained by potentiometry were confirmed by 1H NMR spectroscopy. Indeed, the comparative analysis of the chemical shift values of the studied bioligands allows for reasonably affirming that all of them act as chelating agents of Ca2+. MALDI MS confirmed the formation of complexes and MS/MS experiments and, moreover, indicated different complexing behaviors of the ligands toward Ca2+. The results suggest that Cys and PSH act as bidentate ligands giving rise to six-membered cycles via O and S; GSH and GSSG bind to Ca2+ ion via O and N. By potentiometry, formation constant values under different temperatures were evaluated. In this way were also obtained TΔS and ΔH values, necessary to calculate formation constants at different temperatures. The sequestering ability of Cys, PSH, GSH, and GSSG toward Ca2+ was evaluated under different pH and temperature conditions, with particular attention to those simulating biological fluids, evidencing an interesting trend.
Finally, obtained stability data were crucial to gain simulations under biological fluid conditions, as blood and lens water, and pointed out the importance of reliable thermodynamic data for simulations useful for applications to real systems, characterized by variable composition and pH.
Data Availability Statement
The original contributions presented in the study are included in the article/Supplementary Material; further inquiries can be directed to the corresponding author.
Author Contributions
OG planned the experiments, supervised and organized the analysis, performed speciation calculations and simulations, and wrote the manuscript. CF contributed to conception, design of the study, analysis of the results, and manuscript revision. FC performed the potentiometric measurements, prepared the solutions for 1H NMR experiments, and contributed to spectra acquisition. MC performed the 1H NMR experiments and the qualitative analysis of the spectra and contributed to 1H NMR section. DA contributed to experimental design of the study. DA and AN performed MALDI MS and MS/MS experiments and wrote mass spectrometry discussion. All authors contributed to manuscript revision, read, and approved the submitted version.
Funding
Publication fees will be covered by the University of Messina FFABR 2020 funds, University of Calabria funds and by Frontiers discount (Discount Code: DSC-11002218503PRD).
Conflict of Interest
The authors declare that the research was conducted in the absence of any commercial or financial relationships that could be construed as a potential conflict of interest.
Supplementary Material
The Supplementary Material for this article can be found online at: https://www.frontiersin.org/articles/10.3389/fchem.2021.640219/full#supplementary-material.
Acknowledgments
The authors OG and CF thank University of Messina for Research & Mobility 2017 funds (ARCADIA project) and for FFABR 2020 funds. The authors DA and AN thank University of Calabria for funds. All the authors thank Frontiers Fee Support Team for the discount granted.
References
Aiello, D., Giambona, A., Leto, F., Passarello, C., Damiani, G., Maggio, A., et al. (2018b). Human coelomic fluid investigation: a MS-based analytical approach to prenatal screening. Sci. Rep. 8, 10973. doi:10.1038/s41598-018-29384-9
Aiello, D., Siciliano, C., Mazzotti, F., Di Donna, L., Athanassopoulos, C. M., and Napoli, A. (2020a). A rapid MALDI MS/MS based method for assessing saffron (Crocus sativus L.) adulteration. Food Chem 307, 125527. doi:10.1016/j.foodchem.2019.125527
Aiello, D., Cardiano, P., Cigala, R. M., Gans, P., Giacobello, F., Giuffrè, O., et al. (2017). Sequestering ability of oligophosphate ligands toward Al3+ in aqueous solution. J. Chem. Eng. Data 62, 3981–3990. doi:10.1021/acs.jced.7b00685
Aiello, D., Furia, E., Siciliano, C., Bongiorno, D., and Napoli, A. (2018a). Study of the coordination of ortho-tyrosine and trans-4-hydroxyproline with aluminum(III) and iron(III). J. Mol. Liq 269, 387–397. doi:10.1016/j.molliq.2018.08.074
Aiello, D., Siciliano, C., Mazzotti, F., Di Donna, L., Risoluti, R., and Napoli, A. (2020b). Protein extraction, enrichment and MALDI MS and MS/MS analysis from bitter orange leaves (citrus aurantium). Molecules 25, 1485. doi:10.3390/molecules25071485
Alderighi, L., Gans, P., Ienco, A., Peters, D., Sabatini, A., and Vacca, A. (1999). Hyperquad simulation and speciation (HySS): a utility program for the investigation of equilibria involving soluble and partially soluble species. Coord. Chem. Rev 184, 311–318.
Brigham, M. P., Stein, W. H., and Moore, S. (1960). The concentrations of cysteine and cystine in human blood plasma. J. Clin. Invest 39, 1633–1638. doi:10.1172/JCI104186
Bringhurst, F. R., and Potts, J. T. (1979). “Calcium and phosphate,” in Endocrinology. Editor L. J. e. a. De Groot (New York: Grune & Stratton).
Broaudus, A. E. (1993). “Physiological functions of calcium, magnesium and phosphorous and mineral ion balance,” in Primer on the metabolic bone diseases and disorders of mineral metabolism. Editor M. J. Favus (New York: Raven Press).
Cardiano, P., De Stefano, C., Giuffrè, O., and Sammartano, S. (2008). Thermodynamic and spectroscopic study for the interaction of dimethyltin(IV) with L-cysteine in aqueous solution. Biophys. Chem 133, 19–27. doi:10.1016/j.bpc.2007.11.005
Cardiano, P., Falcone, G., Foti, C., Giuffrè, O., and Napoli, A. (2013). Binding ability of glutathione towards alkyltin(IV) compounds in aqueous solution. J. Inorg. Biochem 129, 84–93. doi:10.1016/j.jinorgbio.2013.09.004
Cardiano, P., Cordaro, M., Chillè, D., Foti, C., and Giuffrè, O. (2019). Interactions of inosine 5′-monophosphate with Ca2+ and Mg2+: a thermodynamic and spectroscopic study in aqueous solution. J. Chem. Eng. Data 64, 2859–2866. doi:10.1021/acs.jced.9b00231
Cardiano, P., Cucinotta, D., Foti, C., Giuffrè, O., and Sammartano, S. (2011). Potentiometric, calorimetric and 1H-NMR investigation on Hg2+-mercaptocarboxylate interaction in aqueous solution. J. Chem. Eng. Data 56, 1995–2004. doi:10.1021/je101007n
Cardiano, P., Giacobello, F., Giuffrè, O., and Sammartano, S. (2016). Thermodynamics of Al3+-thiocarboxylate interaction in aqueous solution. J. Mol. Liq 222, 614–621. doi:10.1016/j.molliq.2016.07.077
Cardiano, P., Giuffrè, O., Napoli, A., and Sammartano, S. (2009). Potentiometric, 1H-NMR, ESI-MS investigation on dimethyltin(IV) cation-mercaptocarboxylate interaction in aqueous solution. New J. Chem 33, 2286–2295. doi:10.1039/b908114c
Cardiano, P., Giuffrè, O., Pellerito, L., Pettignano, A., Sammartano, S., and Scopelliti, M. (2006). Thermodynamic and spectroscopic study of the binding of dimethyltin(IV) by citrate at 25°C. Appl. Organomet. Chem 20, 425–435. doi:10.1002/aoc.1076
Cassone, G., Chillè, D., Giacobello, F., Giuffrè, O., Mollica Nardo, V., Ponterio, R. C., et al. (2019). Interaction between As(III) and simple thioacids in water: an experimental and ab initio molecular dynamics investigation. J. Phys. Chem. B 123, 6090–6098. doi:10.1021/acs.jpcb.9b04901
Chandorkar, A. G., Bulakh, P. M., and Albal, M. V. (1980). Electrolyte composition in normal and cataractous lenses. Indian J. Ophthalmol 28, 135–138.
Chillè, D., Aiello, D., Grasso, G. I., Giuffrè, O., Napoli, A., Sgarlata, C., et al. (2020). Complexation of As(III) by phosphonate ligands in aqueous fluids: thermodynamic behavior, chemical binding forms and sequestering abilities. J. Environ. Sci. (China) 94, 100–110. doi:10.1016/j.jes.2020.03.056
Cigala, R. M., Crea, F., De Stefano, C., Lando, G., Milea, D., and Sammartano, S. (2012). Modeling the acid-base properties of glutathione in different ionic media, with particular reference to natural waters and biological fluids. Amino Acids 43, 629–648. doi:10.1007/s00726-011-1110-0
Cordaro, M., Foti, C., Giacobello, F., Giuffrè, O., and Sammartano, S. (2019). Phosphonic derivatives of nitrilotriacetic acid as sequestering agents for Ca2+ in aqueous solution: a speciation study for application in natural waters. ACS Earth Space Chem 3, 1942–1954. doi:10.1021/acsearthspacechem.9b00183
Crea, P., De Stefano, C., Kambarami, M., Millero, F. J., and Sharma, V. K. (2008). Effect of ionic strength and temperature on the protonation of oxidized glutathione. J. Solut. Chem 37, 1245–1259. doi:10.1007/s10953-008-9310-2
Davis, C. D., and Hanumegowda, U. M. (2008). Drug metabolism handbook. Hoboken, NJ: John Wiley & Sons.
De Stefano, C., Foti, C., Giuffrè, O., and Milea, D. (2016). Complexation of Hg2+, CH3Hg+, Sn2+, and (CH3)2Sn2+ with phosphonic NTA derivatives. New J. Chem 40, 1443–1453. doi:10.1039/C5NJ02531A
De Stefano, C., Foti, C., Giuffrè, O., and Sammartano, S. (2014). Acid-base and UV behaviour of 3-(3,4-dihydroxyphenyl)-propenoic acid (caffeic acid) and complexing ability towards different divalent metal cations in aqueous solution. J. Mol. Liquids 195, 9–16. doi:10.1016/j.molliq.2014.01.027
De Stefano, C., Sammartano, S., Mineo, P., and Rigano, C. (1997). “Computer tools for the speciation of natural fluids,” in Marine chemistry - an environmental analytical chemistry approach. Editors A. Gianguzza, E. Pelizzetti, and S. Sammartano (Amsterdam: Kluwer Academic Publishers), 71–83.
Dringen, R. (2000). Metabolism and functions of glutathione in brain. Prog. Neurobiol 62, 649–671. doi:10.1016/s0301-0082(99)00060-x
Falcone, G., Foti, C., Gianguzza, A., Giuffrè, O., Napoli, A., Pettignano, A., et al. (2013). Sequestering ability of some chelating agents towards methylmercury(II). Anal. Bioanal. Chem 405, 881–893. doi:10.1007/s00216-012-6336-5
Falcone, G., Giuffrè, O., and Sammartano, S. (2011). Acid-base and UV properties of some aminophenol ligands and their complexing ability towards Zn2+ in aqueous solution. J. Mol. Liquids 159, 146–151. doi:10.1016/j.molliq.2011.01.003
Filella, M., and May, P.M. (2005). Reflections on the calculation and publication of potentiometrically-determined formation constants. Talanta 65, 1221–1225.
Foti, C., and Giuffrè, O. (2020). Interaction of ampicillin and amoxicillin with Mn2+: a speciation study in aqueous solution. Molecules 25, 3110. doi:10.3390/molecules25143110
Frassineti, C., Ghelli, S., Gans, P., Sabatini, A., Moruzzi, M. S., and Vacca, A. (1995). Nuclear magnetic resonance as a tool for determining protonation constants of natural polyprotic bases in solution. Anal. Biochem 231, 374–382. doi:10.1006/abio.1995.9984
Frausto da Silva, J. J. R., and Williams, R. J. P. (2001a). “Calcium: controls and triggers,” in The biological chemistry of the elements: the inorganic chemistry of life. Oxford University Press, 278–314.
Frausto da Silva, J. J. R., and Williams, R. J. P. (2001b). “The principles of the uptake and chemical speciation of the elements in biology,” in The biological chemistry of the elements: the inorganic chemistry of life. Oxford University Press.
Furia, E., Aiello, D., Di Donna, L., Mazzotti, F., Tagarelli, A., Thangavel, H., et al. (2014). Mass spectrometry and potentiometry studies of Pb(II)-, Cd(II)- and Zn(II)-cystine complexes. Dalton Trans 43, 1055–1062. doi:10.1039/c3dt52255e
Gianguzza, A., Giuffrè, O., Piazzese, D., and Sammartano, S. (2012). Aqueous solution chemistry of alkyltin(IV) compounds for speciation studies in biological fluids and natural waters. Coord. Chem. Rev 256, 222–239. doi:10.1016/j.ccr.2011.06.027
Giuffrè, O., Aiello, D., Chillè, D., Napoli, A., and Foti, C. (2020). Binding ability of arsenate towards Cu2+ and Zn2+: thermodynamic behavior and simulation under natural water conditions. Environ. Sci. Process. Impacts 22, 1731–1742. doi:10.1039/d0em00136h
Giuffrè, O., Angowska, S., Foti, C., and Sammartano, S. (2019). Thermodynamic study on the interaction of ampicillin and amoxicillin with Ca2+ in aqueous solution at different ionic strengths and temperatures. J. Chem. Eng. Data 64, 800–809. doi:10.1021/acs.jced.8b01081
Hluchan, S. E., and Pomerantz, K. (2002). “Calcium and calcium alloys,” in Ullmann's Encyclopedia of industrial chemistry. Weinheim, Germany: Wiley-VCH Verlag GmbH&Co KGaA, 41–44.
Imbrogno, S., Aiello, D., Filice, M., Leo, S., Mazza, R., Cerra, M. C., et al. (2019). MS-based proteomic analysis of cardiac response to hypoxia in the goldfish (Carassius auratus). Sci. Rep 9, 18953. doi:10.1038/s41598-019-55497-w
Jones, M. M. (1991). New developments in therapeutic chelating agents as antidotes for metal poisoning. Crit. Rev. Toxicol 21, 209–233. doi:10.3109/10408449109089880
Kisic, B., Miric, D., Zoric, L., Ilic, A., and Dragojevic, I. (2012). Antioxidant capacity of lenses with age-related cataract. Oxid. Med. Cell. Longev, 2012, 467130. doi:10.1155/2012/467130
Königsberger, L.-C., Königsberger, E., Hefter, G., and May, P. M. (2015). Formation constants of copper(I) complexes with cysteine, penicillamine and glutathione: implications for copper speciation in the human eye. Dalton Trans 44, 20413–20425. doi:10.1039/C5DT02129D
Kretzschmar, J., Strobel, A., Haubitz, T., Drobot, B., Steudtner, R., Barkleit, A., et al. (2020). Uranium(VI) complexes of glutathione disulfide forming in aqueous solution. Inorg. Chem 59, 4244–4254. doi:10.1021/acs.inorgchem.9b02921
Labib, M., Sargent, E. H., and Kelley, S. O. (2016). Electrochemical methods for the analysis of clinically relevant biomolecules. Chem. Rev 116, 9001–9090. doi:10.1021/acs.chemrev.6b00220
Laurie, S. H., Prime, D. H., and Sarkar, B. (1979). Analytical potentiometric and spectroscopic study of the equilibria in the aqueous nickel(II)-triethylenetetramine and nickel(II)–D-penicillamine systems. Can. J. Chem 57, 1411–1417. doi:10.1139/v79-230
Martell, A. E., Smith, R. M., and Motekaitis, R. J. (2004). Critically selected stability constants of metal complexes. Garthersburg, MD: National Institute of Standard Technology.
May, P. M., and Murray, K. (2001). Database of chemical reactions designed to achieve thermodynamic consistency automatically. J. Chem. Eng. Data 46, 1035–1040. doi:10.1021/je000246j
Meister, A., and Anderson, M. E. (1983). Glutathione. Annu. Rev. Biochem 52, 711–760. doi:10.1146/annurev.bi.52.070183.003431
Olmos Moya, P. M., Martínez Alfaro, M., Kazemi, R., Alpuche-Avilés, M. A., Griveau, S., Bedioui, F., et al. (2017). Simultaneous electrochemical speciation of oxidized and reduced glutathione. Redox profiling of oxidative stress in biological fluids with a modified carbon electrode. Anal. Chem 89, 10726–10733. doi:10.1021/acs.analchem.7b01690
Pescosolido, N., Barbato, A., Giannotti, R., Komaiha, C., and Lenarduzzi, F. (2016). Age-related changes in the kinetics of human lenses: prevention of the cataract. Int. J. Ophthalmol 9, 1506–1517. doi:10.18240/ijo.2016.10.23
Peterlik, M., and Stoeppler, M. (2004). “Calcium,” in Elements and their compounds in the environment. Editors E. Merian, M. Anke, M. Ihnat, and M. Stoeppler (Weinheim: Wiley VCH), 599–618.
Pettit, L. D., and Powell, K. J. (2001). IUPAC stability constants database. IUPAC: Academic Software.
Pompella, A., Visvikis, A., Paolicchi, A., De Tata, V., and Casini, A. F. (2003). The changing faces of glutathione, a cellular protagonist. Biochem. Pharmacol 66, 1499–1503. doi:10.1016/s0006-2952(03)00504-5
Ramamoorthy, S., and Manning, P. G. (1975). Equilibrium studies of solutions containing Al3+, Ca2+ or Cd2+ and cysteine, orthophosphate and a carboxylic acid. J. Inorg. Nucl. Chem 37, 363–367. doi:10.1016/0022-1902(75)80206-5
Salvatore, L., Gallo, N., Aiello, D., Lunetti, P., Barca, A., Blasi, L., et al. (2020). An insight on type I collagen from horse tendon for the manufacture of implantable devices. Int. J. Biol. Macromol 154, 291–306. doi:10.1016/j.ijbiomac.2020.03.082
Shahid, N. M., Khalid, S., Bibi, I., Bundschuh, J., Khan Niazi, N., and Dumat, C. (2020). A critical review of mercury speciation, bioavailability, toxicity and detoxification in soil-plant environment: ecotoxicology and health risk assessment. Sci. Total Environ 711, 134749. doi:10.1016/j.scitotenv.2019.134749
Sies, H. (1999). Glutathione and its role in cellular functions. Free Radic. Biol. Med 27, 916–921. doi:10.1016/s0891-5849(99)00177-x
Singh, P. K., Garg, B. S., Kumar, D. N., and Singh, B. K. (2001). Complexation equilibria and evaluation of thermodynamic parameters of bivalent metal complexes of glutathione. Indian J. Chem 40A, 1339–1343.
Sisombath, N. S., Jalilehvand, F., Schell, A. C., and Wu, Q. (2014). Lead(II) binding to the chelating agent D-Penicillamine in aqueous solution. Inorg. Chem 53, 12459–12468. doi:10.1021/ic5018714
Touche, M. L. D., and Williams, D. R. (1976). Thermodynamic considerations in Co-ordination. Part XXI formation of ternary complexes containing two dissimilar metal ions and the implication for metal-metal stimulation phenomena in vivo. J. Chem. Soc. Dalton Trans, 1355–1359. doi:10.1039/DT9750001757
Walshe, J. M. (1956). Penicillamine, a new oral therapy for Wilson's disease. Am. J. Med 21, 487–495. doi:10.1016/0002-9343(56)90066-3
Keywords: Ca2+, biological ligands, speciation in biological fluids, sequestration, potentiometry, 1H NMR spectroscopy, mass spectrometry, thermodynamic parameters
Citation: Aiello D, Carnamucio F, Cordaro M, Foti C, Napoli A and Giuffrè O (2021) Ca2+ Complexation With Relevant Bioligands in Aqueous Solution: A Speciation Study With Implications for Biological Fluids. Front. Chem. 9:640219. doi: 10.3389/fchem.2021.640219
Received: 10 December 2020; Accepted: 11 January 2021;
Published: 24 February 2021.
Edited by:
Alberto Salomone, University of Turin, ItalyReviewed by:
Artik Elisa Angkawijaya, National Taiwan University of Science and Technology, TaiwanLuca Rivoira, School of Nature Sciences, University of Turin, Italy
Copyright © 2021 Aiello, Carnamucio, Cordaro, Foti, Napoli and Giuffrè. This is an open-access article distributed under the terms of the Creative Commons Attribution License (CC BY). The use, distribution or reproduction in other forums is permitted, provided the original author(s) and the copyright owner(s) are credited and that the original publication in this journal is cited, in accordance with accepted academic practice. No use, distribution or reproduction is permitted which does not comply with these terms.
*Correspondence: Ottavia Giuffrè, b2dpdWZmcmVAdW5pbWUuaXQ=