- 1Institute of Marine Biomedicine, Shenzhen Polytechnic, Shenzhen, China
- 2State Key Laboratory of Analytical Chemistry for Life Science, School of Chemistry and Chemical Engineering, Nanjing University, Nanjing, China
- 3College of Engineering and Applied Sciences, Nanjing University, Nanjing, China
Photocatalytic conversion of CO2 into solar fuels has gained increasing attention due to its great potential for alleviating the energy and environmental crisis at the same time. The low-cost TiO2 with suitable band structure and high resistibility to light corrosion has proven to be very promising for photoreduction of CO2 using water as the source of electrons and protons. However, the narrow spectral response range (ultraviolet region only) as well as the rapid recombination of photo-induced electron-hole pairs within pristine TiO2 results in the low utilization of solar energy and limited photocatalytic efficiency. Besides, its low selectivity toward photoreduction products of CO2 should also be improved. Combination of TiO2 with other photoelectric active materials, such as metal oxide/sulfide semiconductors, metal nanoparticles and carbon-based nanostructures, for the construction of well-defined heterostructures can enhance the quantum efficiency significantly by promoting visible light adsorption, facilitating charge transfer and suppressing the recombination of charge carriers, resulting in the enhanced photocatalytic performance of the composite photocatalytic system. In addition, the adsorption and activation of CO2 on these heterojunctions are also promoted, therefore enhancing the turnover frequency (TOF) of CO2 molecules, so as to the improved selectivity of photoreduction products. This review focus on the recent advances of photocatalytic CO2 reduction via TiO2-based heterojunctions with water oxidation. The rational design, fabrication, photocatalytic performance and CO2 photoreduction mechanisms of typical TiO2-based heterojunctions, including semiconductor-semiconductor (S-S), semiconductor-metal (S-M), semiconductor-carbon group (S-C) and multicomponent heterojunction are reviewed and discussed. Moreover, the TiO2-based phase heterojunction and facet heterojunction are also summarized and analyzed. In the end, the current challenges and future prospects of the TiO2-based heterostructures for photoreduction of CO2 with high efficiency, even for practical application are discussed.
Introduction
Energy and environmental crizes are two major bottlenecks restricting the sustainable development of human society. For a long time, the excessive consumption of fossil fuels has caused severe energy shortages, and the CO2 released during the combustion process has become the main factor leading to global warming (Stott et al., 2000; Meinshausen et al., 2009; Solomon et al., 2009; Höök and Tang, 2013). It is urgent to develop and utilize renewable clean energy while reducing the concentration of CO2 in the atmosphere (Brockway et al., 2019; Shindell and Smith, 2019). Notably, as a simple form of carbon storage, the rich carbon resources contained in CO2 have huge development potential. Using CO2 as a carbon feedstock to prepare carbon-based fuels can help alleviate the energy crisis and global warming at the same time, and has become a current research hotspot in the fields of both energy and environment (Olah et al., 2011; Kondratenko et al., 2013; Aresta et al., 2014; Ganesh, 2014; Li et al., 2019). However, the liner molecule with high thermodynamic stability and kinetic inertness makes it a great challenge for the activation and conversion of CO2 (Ola and Maroto-Valer, 2015; Wei L. et al., 2018; Li et al., 2019; Nguyen et al., 2020). A lot of energy needs to be injected to break the C=O bond (dissociation energy about 750 kJ mol−1) in CO2 (Kim et al., 2017). Moreover, the extremely low water solubility of CO2 (about 30 mM under 25°C at 1 atm) results in the low conversion efficiency of CO2 in the aqueous reaction system (Xie et al., 2014). Therefore, a highly efficient reaction mode is also in great demand.
Fortunately, the natural photosynthesis motivated by solar energy to covert CO2 into carbonhydrates as well as the release of O2 by water oxidation provides a very promising solution to reduce the CO2 level in atmosphere, which inspires the development of artificial photosynthesis systems (Barber, 2009; Dogutan and Nocera, 2019; Zhang and Sun, 2019). As shown in Figure 1A, the water oxidation process takes place in the photosystem II (PSII) of green plants to provide electrons and protons for the CO2 fixation and conversion in the photosystem I (PSI). Since a series of pioneering works devoted by Fujishima and Honda on semiconductor photocatalysis in the 1970s (Fujishima and Honda, 1972; Inoue et al., 1979), substantial efforts have been made for the combination of the two individual processes within a single artificial architecture to mimic the natural photosynthesis (shown in Figure 1B) during the past decades (Ma et al., 2014; Tu et al., 2014; White et al., 2015; Liu X. et al., 2016; Li et al., 2019; Xu and Carter, 2019).
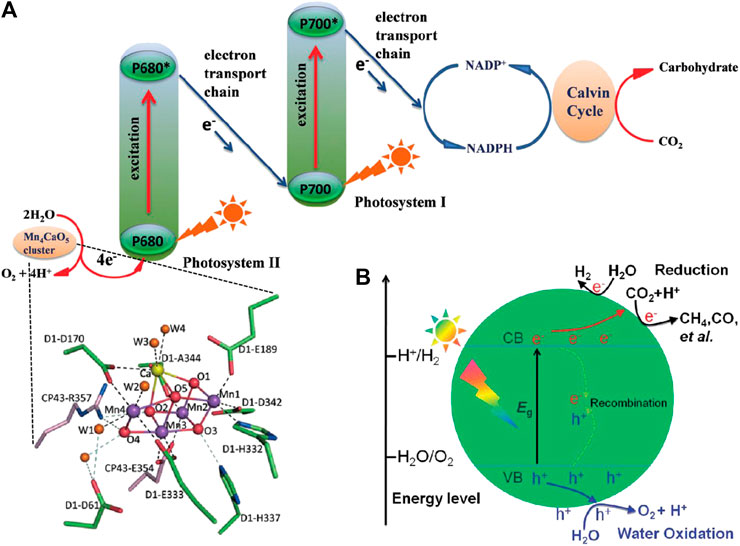
FIGURE 1. Schematic diagrams of (A) natural photosynthesis and (B) semiconductor photocatalytic reduction of CO2. Reproduced from Liu et al. (2016) with permission from Wiley-VCH and Wei et al. (2018) with permission from the Royal Society of Chemistry.
In a typical semiconductor photocatalytic process, the semiconductor photocatalyst is excited by the incident photons with energy greater than or equal to its bandgap energy (Eg), where electrons (e-) are excited to the conduction band (CB) to participate in the reduction reactions, remaining holes (h+) in the valence band (VB) for the oxidation reactions. Considering that CO2 molecule is very stable and the photocatalytic CO2 reduction is a series of uphill reactions (ΔG0>0, see in Table 1) (Wei L. et al., 2018), a large energy input is necessary to covert CO2 into solar fuels, corresponding to the photons in the ultraviolet or shortwave visible regions. In addition, the band structure of ideal semiconductors needs to meet the redox potentials of CO2 reduction and water oxidation reactions at the same time, as listed in Table 2 (Habisreutinger et al., 2013; White et al., 2015). Generally, the CB edge position (ECB) should be more negative than the potential for reducing CO2, whereas the VB edge position (EVB) should be more positive than the potential for oxidizing H2O to O2. So far, the photocatalytic activity of numerous photocatalysts, etc. TiO2 (Habisreutinger et al., 2013; Ma et al., 2014; Ola and Maroto-Valer, 2015), ZnO (Lee K. M. et al., 2016; Nie et al., 2018; Kegel et al., 2018), WO3 (Chen et al., 2012; Shi et al., 2019; Wang H. et al., 2019), SnO2 (He et al., 2015; You et al., 2020), Cu2O (An et al., 2014; Shi et al., 2019), CdS (Kuai et al., 2015; Kuehnel et al., 2017; Wei Z. H. et al., 2018; Bie et al., 2019; Low et al., 2019), Bi2WO6 (Cao et al., 2018; Liu et al., 2021), BiVO4 (Mao et al., 2012; Wei Z. H. et al., 2018), BiOBr (Ye et al., 2016; Wu et al., 2018), g-C3N4 (He et al., 2015; Nie et al., 2018; Thi Thanh Truc et al., 2019; Wang et al., 2020a) and graphene (An et al., 2014; Xiong et al., 2016; Shehzad et al., 2018a; Zhao H. et al., 2018; Bie et al., 2019), have been investigated intensively, in which few of them can realize the synergism of photocatalytic CO2 reduction and water oxidation. In particular, the low-cost TiO2 with suitable band structure and high resistibility to light corrosion is a very promising candidate, which has become the benchmark in this field (Habisreutinger et al., 2013; Ma et al., 2014; Ola and Maroto-Valer, 2015). However, the wide band gap of TiO2 (3.2 eV for anatase) responses to UV light only, which accounts for only 3–5% of the incoming solar spectrum, thus restricting the conversion efficiency of solar energy. Besides, the fast recombination of photo-induced e-/h+ pairs within TiO2 results in the low charge separation efficiency, therefore reducing its photocatalytic performance further. Moreover, the low selectivity toward photoreduction products of CO2 based on aqueous TiO2 suspension photocatalytic system should also be improved.
In the past few decades, various of strategies have been developed to enhance the photocatalytic performance of TiO2. Among them, the nanostructured TiO2 with single crystalline phase exhibited the decreased recombination rate of charge carriers, comparing to the polycrystalline samples that possess large amount of grain boundaries and defects acting as recombination centers (Tu et al., 2014; Xu et al., 2015). Moreover, crystal facet engineering has been adopted to tune the surface energy and active sites of TiO2, contributing to the adsorption and activation of CO2 (Liu L. et al., 2016; Xiong et al., 2018; Tu et al., 2020). Obviously, the preference adsorption of CO2 molecules at the surface oxygen vacancy sites of TiO2 can reduce the reactive barrier of CO2 photoreduction reactions, in which one oxygen atom of CO2 is trapped by a bridging oxygen vacancy defect to induce affinity interactions (Lee et al., 2011; Liu L. et al., 2016; Tan et al., 2017). Moreover, the localized electrons of oxygen vacancies can form adventitious energy levels, extending the photoresponsive range of semiconductor photocatalyst. Besides, surface oxygen vacancies with typical defect states can trap electrons or holes to inhibit their recombination (Wang et al., 2018). To sum up, the significance of surface oxygen vacancies on defected TiO2 has been ascertained in the enhancement of CO2 adsorption, activation, dissolution, and stabilization of reaction intermediates (Nguyen et al., 2020). In addition, metal/nonmetal ion doping is used to introduce additional energy level between the band gap of TiO2, resulting in the reduced band width and enhanced visible light adsorption (Tu et al., 2014; Ola and Maroto-Valer, 2015; Shehzad et al., 2018b; Patil et al., 2019). Dye sensitized TiO2 displays enhanced photoreduction efficiency of CO2 due to the injection of photosensitized electrons from the energy level of dye molecule to the CB of TiO2 with more negative potential, while the superior visible light responsibility of dye molecules can also improve the utilization of incident light (Ma et al., 2014; Ola and Maroto-Valer, 2015; Lee J. S. et al., 2016; Woo et al., 2019). Although these strategies have proven to be effective, the charge separation efficiency, light energy utilization and product selectivity still need to be further improved to fulfill the demand of more efficient photoreduction of CO2, even for the practical application in the future.
As is known, construction of heterojunction between TiO2 and cocatalyst with matching electronic band structures can significantly promote the separation of photogenerated e- and h+, enlarge the spectra response range, while the physicochemical properties of some special cocatalyst can promote the photocatalytic CO2 reduction or water oxidation to a certain extent, thereby resulting in high photoreduction efficiency of CO2 over the heterostructured phocatalytic system with enhanced reduction products selectivity (Ma et al., 2014; Wang et al., 2014; Wei L. et al., 2018; Nguyen et al., 2020).
In addition to photocatalysts, photoreactors as well as reaction modes also play vital roles in affecting the photoreduction efficiency of CO2. Generally, the two key parameters which determine the types of photoreactors utilized in CO2 photoreduction are the phases involved (i.e., gas-solid, liquid-solid) and the mode of operation (i.e., batch, semi-batch or continuous). In the solid-liquid cases, photocatalysts are usually dispersed in alkaline mediums (aqueous solution) which can realize higher CO2 solubility, resulting in the formation of CO32− and HCO3−. However, these species are difficult to be reduced in comparison with CO2 (Corma and Garcia, 2013). In order to overcome the above drawbacks, the solid-vapor mode has been widely applied where the generation rate of the products for CO2 photoreduction can be improved significantly (Xie et al., 2014; Xie et al., 2016; Xiong et al., 2017b). In addition, the exposure of photocatalysts in a CO2 atmosphere can reduce the generation of H2, thus enhancing the selectivity for CO2 reduction. Obviously, the solid-vapor mode is more suitable for CO2 photoreduction in the presence of H2O.
In this review, we will mainly focus on the recent advances of photocatatytic CO2 reduction processes with water oxidation using TiO2-based heterojunction as photocatalysts. Different categories of heterojunctions, including S-S heterojunction (Figure 2A), S-M heterojunction (Figure 2B), S-C heterojunction (Figure 2C), multicomponent heterojunction, phase heterojunction and facet heterojunction (Figure 2D) are reviewed individually. In addition, the unique functions of cocatalysts among different heterostructured photocatalytic systems (etc. photosensitizer, CO2 reduction promoter, water oxidation promoter and surface plasmon resonance (SPR) source) as well as the photoreduction mechanisms of CO2 are discussed in detail. In the end, we will look forward to the prospects, opportunities and challenges of photocatalytic CO2 reduction, predict the research directions of this field in the future, and put forward our opinions on the construction of efficient multifunctional integrated photocatalytic CO2 reduction systems. We believe that this review will provide some useful guidelines for the construction of heterostructured photocatalysts for photoreduction of CO2 with high performance in the future.
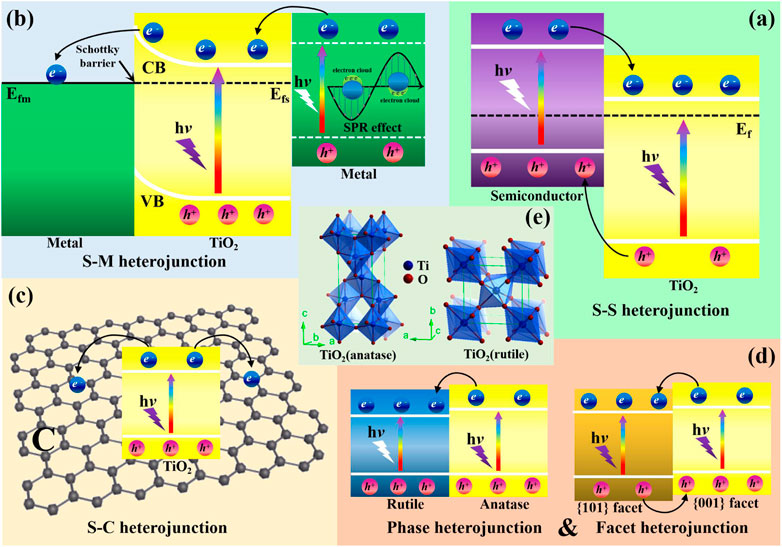
FIGURE 2. Separation and transfer of photogenerated charge carriers in the TiO2-based (A) S-S heterojunction, (B) S-M heterojunction, (C) S-C heterojunction and (D) phase and facet heterojunction; (E) Crystalline structures of TiO2 (anatase) and TiO2 (rutile).
Photoreduction of CO2 to Solar Fuels on TiO2-Based Heterojunctions
From the perspective of semiconductor photocatalysis, the premise of high photocatalytic efficiency is the efficient separation and rapid transfer of photogenerated e-/h+ pairs, thereby prolonging their lifetimes and inhibiting their recombination. The strategy for the construction of heterojunction by coupling semiconductor (TiO2) with a secondary substance including other semiconductors (Aguirre et al., 2017; Yang et al., 2017; She et al., 2018; Xu et al., 2018a; Jin et al., 2019; Low et al., 2019; Thi Thanh Truc et al., 2019; Wu et al., 2019), metal nanoparticles (Xie et al., 2013; Neaţu et al., 2014; Jiao et al., 2015; Xiong et al., 2015; Lee K. Y. et al., 2016; Yu et al., 2016; Cheng et al., 2017; Tahir et al., 2017; Xiong et al., 2017C; Chong et al., 2018; Low et al., 2018; Tan et al., 2018; Tasbihi et al., 2018a; Wei Y. et al., 2018; Zhao Y. et al., 2018; Khatun et al., 2019; Wang R. et al., 2019; Zeng et al., 2020; Ziarati et al., 2020; Wang et al., 2021) and carbon-based nanostructures (Xia et al., 2007; Tu et al., 2013; Gui et al., 2014; Chowdhury et al., 2015; Xiong et al., 2016; Lin et al., 2017; Tan et al., 2017; Wang et al., 2017; Li et al., 2018; Olowoyo et al., 2018; Shehzad et al., 2018a; Zhang J. et al., 2018; Zubair et al., 2018; Bie et al., 2019; Olowoyo et al., 2019; Wang R. et al., 2019; Rodríguez et al., 2020) has been generally applied. Since different phases (etc. anatase, brookite, or rutile) and exposed facets (etc. (001) or (101)) of TiO2 exhibit various of band structure and reactivity, TiO2-based phase heterojunction (Reñones et al., 2016; Hwang et al., 2019; Jin et al., 2019; Chen et al., 2020; Lee J. S. et al., 2016; Xiong et al., 2020) or facet heterojunction (Yu et al., 2014; Cao et al., 2016; Liu L. et al., 2016; Xiong et al., 2016; Xiong et al., 2017a; Di Liberto et al., 2019) are also fabricated for photocatalytic CO2 reduction, which exhibits enhanced photocatalytic efficiency in comparison with pristine TiO2. On the one hand, the heterostructure facilitates the separation of photoinduced charge carriers which then transfer across the heterointerface to restrain recombination. On the other hand, the additional active sites introduced by the cocatalysts favor for the adsorption and activation of CO2, thus enhancing the photoreduction efficiency of CO2. Besides, the promoted quantum efficiency and product selectivity can also be expected by the constructed heterojunctions, since light-excitation attributes, band structure, and separation efficiency of photogenerated charge carriers of heterojunctions play vital roles in the selectivity of CO2 photoreduction products. Moreover, various cocatalysts with different reactive active sites can also affect the product selectivity greatly, where the adsorption/activation of CO2 as well as the adsorption/desorption of the intermediates are tuned (Fu et al., 2019).
In this section, the rational design, fabrication, photocatalytic performance and photoreduction mechanism of CO2 over the TiO2-based typical categories of heterojunctions (S-S, S-M, S-C, multicomponent, phase and facet heterojunction) will be reviewed and discussed in detail. In addition, the selectivity toward photoreduction products is another focus. The relative mechanism was concluded and analyzed in the certain case.
TiO2 Based Z-scheme S-S Heterojunction for CO2 Photoreduction
Coupling n-type TiO2 with a p-type semiconductor possessing matching energy band structure to form a p-n heterojunction is one of the most classic S-S heterojunction (Zeng et al., 2014; Aguirre et al., 2017; Lee et al., 2017; Zhang L. et al., 2018; Iqbal et al., 2020; Zhang et al., 2020). As shown in Figure 3, the contacting of the two semiconductors leads to the diffusion of e- and h+, then forms a space-charge region at the interface of the p-n heterojunction (Wang et al., 2014). As a result, a strong built-in electrical field is created which can drive the photoinduced e- and h+ to transfer in the opposite directions, therefore enhancing the separation efficiency of charge carriers. In addition to p-n heterojunction, TiO2-based non-p-n heterojunctions are also common (Yang et al., 2017; She et al., 2018; Jin et al., 2019; Low et al., 2019; Thi Thanh Truc et al., 2019; Wu et al., 2019). Typically, two closely integrated semiconductors with staggered band configurations can form a type II-1 heterojunction (shown in Figure 4A) (Zhang and Jaroniec, 2018), in which the band bending facilitates the charge transfer at the heterointerface. Specifically, e- and h+ are separated individually in both semiconductor 1 (SC-1) and semiconductor 2 (SC-2) under the irradiation of incident light. The difference in energy level leads to the transfer of e- from the CB of SC-1 with more negative potential to the CB of SC-2. Meanwhile, h+ can transfer from the VB of SC-2 to the VB of SC-1 with more positive potential. Similar to the p-n heterojunction, the reverse migration of e- and h+ in the type II-1 heterojunction improves the separation efficiency of charge carriers, thus endowing the enhanced photocatalytic performance of the heterostructured system. However, the way of carrier transfer in the above heterojunctions will lead to a decrease in their redox ability, making it difficult to ensure the optimal photocatalytic activity.
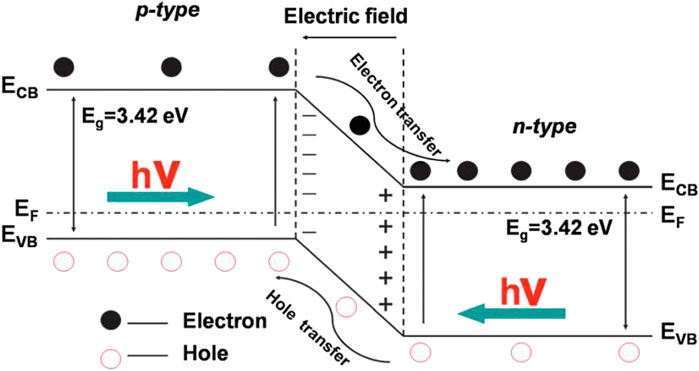
FIGURE 3. Schematic diagram showing the energy band structure and electron-hole pair separation in the p-n heterojunction. Reproduced from Wang et al. (2014) with permission from the Royal Society of Chemistry.
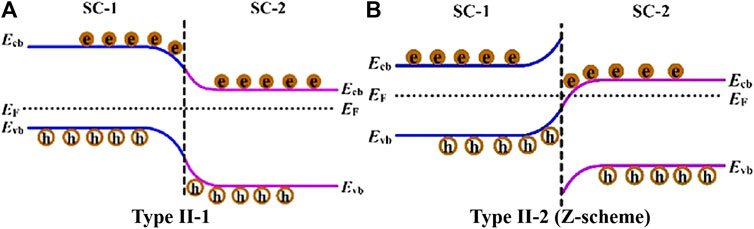
FIGURE 4. Photogenerated charge carrier transfer process for two types of non p-n heterojunctions: (A) type II-1, and (B) type II-2 (direct Z-scheme). Reproduced from Zhang and Jaroniec (2018) with permission from Elsevier and Copyright Clearance Center.
Recently, study on the construction of all-solid-state Z-scheme heterojunction has gained great attention of researchers (Kuai et al., 2015; Aguirre et al., 2017; Takayama et al., 2017; Yang et al., 2017; She et al., 2018; Low et al., 2019; Thi Thanh Truc et al., 2019; Wang et al., 2019; Raza et al., 2020; Wang et al., 2020). Comparing to the p-n and type II-1 heterojunctions, the carrier transfer mode in the Z-scheme heterojunction is more favorable for photocatalytic application. In general, the band bending at the interface of direct Z-scheme heterojunction (type II-2) is conducive to the recombination of photoinduced e- and h+ with weaker reduction and oxidation ability, so that e- in the more negative CB of SC-1 and h+ in the more positive VB of SC-2 can be remained (shown in Figure 4B). As a result, both high separation efficiency and optimal redox ability of photoinduced charge carriers can be realized, thus endowing the high photocatalytic performance of the Z-scheme system. In this section, recent advances for the construction of TiO2-based all-solid-state indirect and direct Z-scheme heterojunctions as well as their application for photocatalytic CO2 reduction with water oxidation will be reviewed and discussed in detail. Photocatalytic CO2 reduction performance of the typical TiO2-based all-solid-state Z-scheme heterojunctions are listed in Table 3.
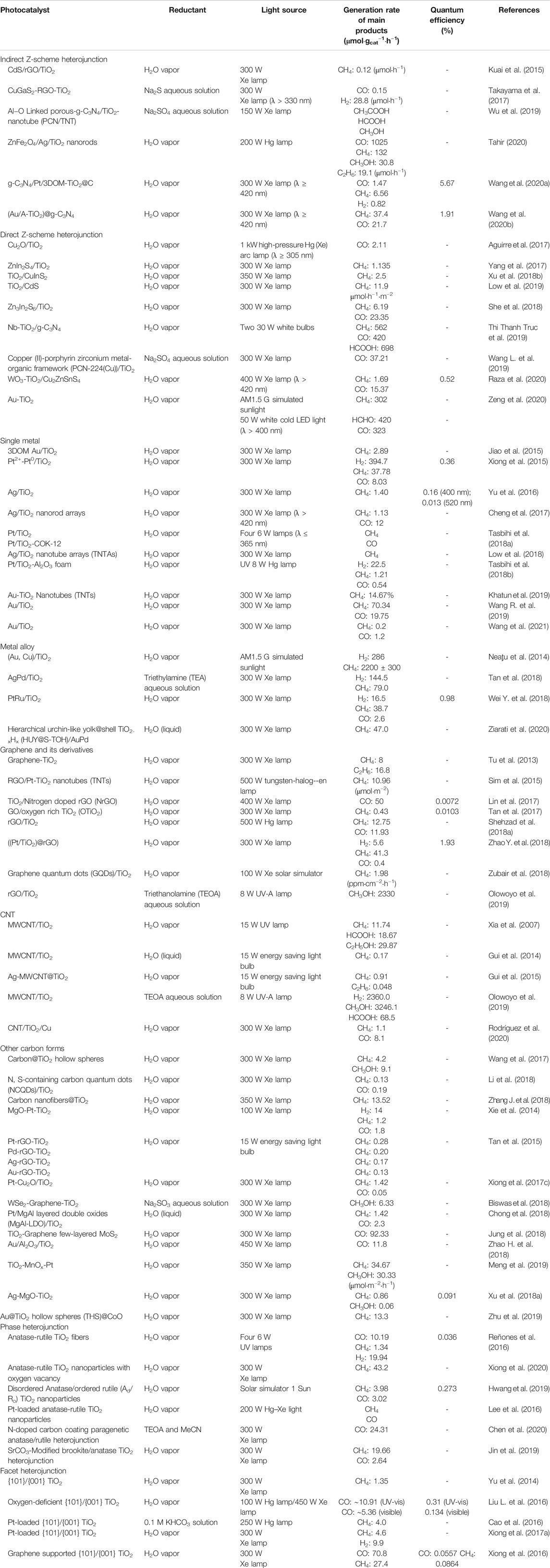
TABLE 3. Photocatalytic CO2 reduction performance on typical TiO2-based S-S (Z-scheme), S-M, S-C, multicomponent, phase and facet heterojunctions.
Construction of indirect Z-scheme system between TiO2 and another semiconductor using noble metals such as Pt (Wang et al., 2020a), Au (Wang et al., 2020b) and Ag (Tahir, 2020) as electron mediators has gained increased attention due its enhanced separation efficiency of photogenerated e−/h+ pairs with the recombination of inefficient charge carriers, thereby improving the photoreduction efficiency of CO2. As reported by Tahir, ZnFe2O4/Ag/TiO2 nanocomposite was fabricated by physical mixing Ag/TiO2 nanorods and ZnFe2O4 nanospheres in methanol solution under continuous stirring (Tahir, 2020). Compared to the point contact between 0D TiO2 nanoarticles and 0D ZnFe2O4 nanospheres, the stronger interaction between 0D ZnFe2O4 nanospheres and 1D TiO2 nanorods is beneficial to the transfer of photogenerated electrons and holes at the interface. At the same time, the migration of these charge carriers along the 1D nanostructure is more efficient, which significantly inhibits their recombination. Moreover, the UV irradiation induced Z-scheme carrier transfer pathway ensures the high redox capability of the remaining carriers with the recombination of inefficient species within Ag nanoparticles, resulting in the superior CO generation rate of 1025 μmol gcat−1 h−1. Compared to ZnFe2O4, graphic-C3N4 (g-C3N4) is more preferred for the construction of TiO2-based Z-scheme heterojunction due to its fully visible light utilization, improved CO2 adsorption capacity (derived from its surface π bond) and proper band structure for CO2 photoreduction with H2O oxidation (Wu et al., 2019; Wang et al., 2020a). Moreover, it can also trap photogenerated electrons to enhance charge separation efficiency within the heterojunction. On this basis, g-C3N4 was coated on the surface of Au/TiO2 hybrid to form a Z-scheme photocatalyst (Figure 5A,B) for visible-light-driven (VLD) photocatalytic CO2 reduction (Wang et al., 2020a). In particular, the efficient separation of photogenerated e−/h+ pairs within anatase TiO2 is attributed to the formation of {001}/{101} facet heterojunction. Then, photogenerated electrons in the CB of TiO2 are directionally transferred through Au and recombine with photogenerated holes in the VB of g-C3N4, thereby boosting the photoreduction of CO2 by photogenerated electrons in the CB of g-C3N4 (shown in Figure 5C). Notably, the high selectivity toward CO2 photoreduction (>99%) was realized with few H2 generation, while the selectivity for CH4 generation (63.3%) was also enhanced compared to pure g-C3N4 (1.4%). The following work of this group (Wang et al., 2020a) devoted to further improving the selectivity for CH4 generation with high apparent quantum efficiency (AQE) under visible light irradiation, in which 3D ordered macroporous (3DOM) TiO2@C was coupled with g-C3N4 using Pt as electron mediator (3DOM-CNPTC) (shown in Figure 5D,E). The DFT calculation revealed the enrichment of photogenerated electorns by abundant N-sites on the interface between Pt and g-C3N4, which can reduce the adsorbed CO2 to CH4 directly in the presence of H2O, thereby improving the selectivity for CH4 generation (81.7%). In addition, the strong visible light adsorption by g-C3N4 and Pt as well as the multiple scattering of incident light within the 3DOM structure (Figure 5F) result in the high AQE of the Z-scheme heterojunction (5.67%), which is 140 folds than that of P25 (0.04%). Interestingly, the interaction between TiO2 and g-C3N4 could also be strengthened by Al-O links which was introduced into the Z-scheme through impregnation (Wu et al., 2019). Specifically, TiO2 nanotubes (TNTs) fabricated via anodization of Ti foils are dipped in AlCl3 solution followed by calcination to obtain Al-O-modified TNTs, which is then combined with porous g-C3N4 (PCN) via solid sublimation and transition of urea/NaHCO3 hybrid to from Al-O linked PCN/TNT composites. Results show that the low charge transfer efficiency at the interface between TiO2 and g-C3N4 caused by lattice mismatch of the two components can be significantly improved by introducing Al-O links to replace surface hydroxyl groups, thereby enhancing the separation efficiency of photogenerated charge carriers and benefiting for photoreduction of CO2 with increased yields of acetic acid, formic acid and methanol. According to Kuai’s research, rGO could also serve as electron mediator for the construction of Z-scheme heterojunction between TiO2 and CdS (Kuai et al., 2015). The remarkably prolonged photoluminasence (PL) decay time of CdS/rGO/TiO2 (2.4 ns) reveals the different electron migration mechanism compared to CdS/TiO2 (0.38 ns), which follows the carrier transfer mode in type II heterojunction. Obviously, the presence of rGO leads to the establishment of Z-scheme system, in which photogenerated electrons in the CB of TiO2 are extracted by rGO and then transferred to the VB of CdS to recombine with photogenerated holes there, resulting in the enrichment of photogenerated electrons and holes in the CB of CdS and the VB of TiO2, respectively. Although the photoreduction efficiency of CO2 is still low on CdS/rGO/TiO2, the attempt to construct Z-scheme heterojunction with low-cost carbon material instead of noble metal as electron mediator is successful, while the high selectivity for CH4 generation is also promising.
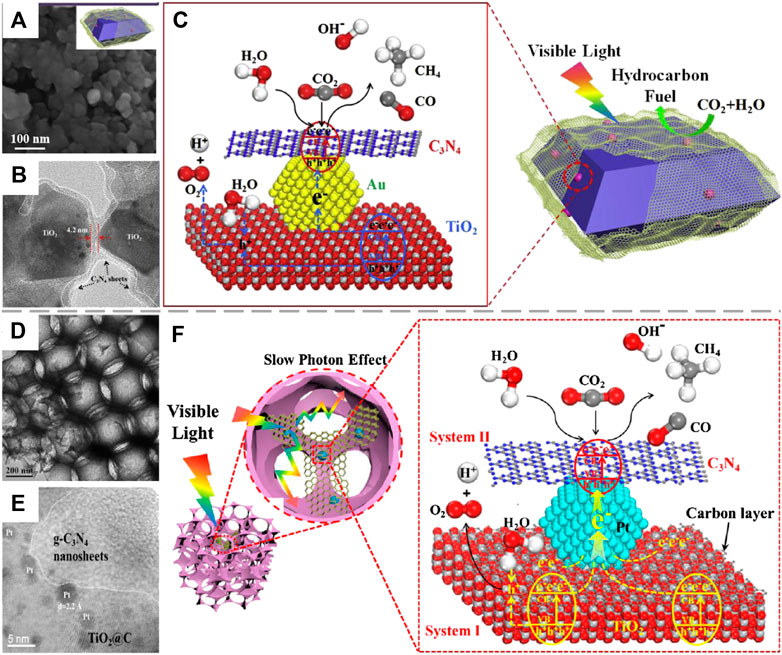
FIGURE 5. (A) SEM and (B) HRTEM images of (Au/A-TiO2)@g-C3N4 catalyst; (C) Schematic for photorecatalytic CO2 reduction with H2O to produce CH4 and CO over (Au/A-TiO2)@g-C3N4. Reproduced from Wang et al., 2020a with permission from Elsevier and Copyright Clearance Center. (D) TEM and (E) HRTEM images of 3DOM-CNPTC photocatalyst; (F) The mechanism of 3DOM-CNPTC catalyst for photocatalytic CO2 reduction with H2O to CH4. Reproduced from Wang et al. (2020) with permission from Elsevier and Copyright Clearance Center.
Recently, construction of direct Z-scheme system by coupling two different semiconductors with matching geometric and band structure has become research hotspot in the field of photocatalytic CO2 reduction, which is more facile to synthesis and more convenient for charge transfer at the interface. In particular, semiconductors with narrow band gap are more preferred in the TiO2-based direct Z-scheme heterojunction to improve the utilization of visible light. Typically, ZnInS2 nanosheets were decorated onto the surface of 1D TiO2 nanobelts via hydrothermal process (Yang et al., 2017). The authors claimed the Z-scheme electron transfer mechanism between ZnInS2 and TiO2 based on the experimental results of CH4 generation. Since the CB potential of TiO2 is lower than the redox potential of CO2/CH4, it is reasonable to believe that photogenerated electrons in the CB of ZnInS2 are retained due to the Z-scheme electron transfer mechanism and take in charge for photocatalytic CO2 reduction to produce CH4. However, stronger evidence is needed to prove this conjecture. In another work, a similar 3D hierarchical nanostructure was constructed by depositing CuInS2 nanoplates on TiO2 nanofibers (Xu et al., 2018b). DFT calculations revealed the higher Fermi level (EF) of CuInS2 than that of TiO2, which forces electrons transfer from CuInS2 to TiO2 after their contact and creates a build in internal electric filed at the interface. The recombination of photogenerated electrons in the CB of TiO2 and photogenerated holes in the VB of CuInS2 under the guidance of the internal electric filed leads to the accomplishment of high efficient Z-scheme charge transfer pathway. As a result, photogenerated electrons enriched in the CB of CuInS2 facilitate the photocatalytic reduction of CO2 to produce CH4 and CH3OH in the presence of protons provided by water oxidation. In situ irradiated X-ray photoelectron spectroscopy (ISI-XPS) was also applied to provide direct evidence of Z-scheme electron transfer mechanism (Low et al., 2019). The binding energy shifts of Ti 2p (by 0.3 eV) and Cd 3 days (by -0.2 eV) under light irradiation indicate the decreased electron density of TiO2 as well as the increased electron density of CdS, suggesting that photogenerated electrons migrates from TiO2 to CdS, which agrees well with Z-scheme mechanism. The ternary semiconductor of Zn3In2S6 was also used by She et al. for the construction of direct Z-scheme heterojunction with TiO2 (She et al., 2018). Higher CO and CH4 yields were realized on Zn3In2S6/TiO2 in comparison with ZnInS2/TiO2 and CuInS2/TiO2, which could be attributed to the higher crystallinity of the two constituents that favored for charge separation (shown in Figure 6). In addition, modification on TiO2 to narrow its band gap for the improved visible light adsorption is also an efficient strategy to further enhance the photocatalytic performance of the TiO2-based Z-scheme heterojunction. As reported by Truc et al., Eg of TiO2 (3.2 eV) was reduced to 2.91 eV after Nb doping (Thi Thanh Truc et al., 2019). The as-obtained Nb-TiO2 was grinded with melamine followed by calcination at 550 °C to form Nb-TiO2/g-C3N4 heterojunction with a clear boundary at the interface. The well matched lattice spacing of the TiO2 {101} (0.353 nm) and g-C3N4 {002} (0.320 nm) facets benefits to the electron transfer at the interface following Z-scheme mechanism, resulting in high efficiency for photocatalytic CO2 reduction. The advantages of low cost, full visible light spectrum responsibility (400–700 nm) and superior generation rate of CH4 (562 μmol gcat−1 h−1), CO (420 μmol gcat−1 h−1) and HCOOH (698 μmol gcat−1 h−1), make Nb-TiO2/g-C3N4 a promising VLD photocatalyst for practical application in the future to reduce the CO2 level in the atmosphere. Moreover, the high O2 yield of Nb-TiO2/g-C3N4 (1702 μmol gcat−1 h−1) indicates that the artificial Z-scheme system can mimic the nature photosynthesis by green plants well.
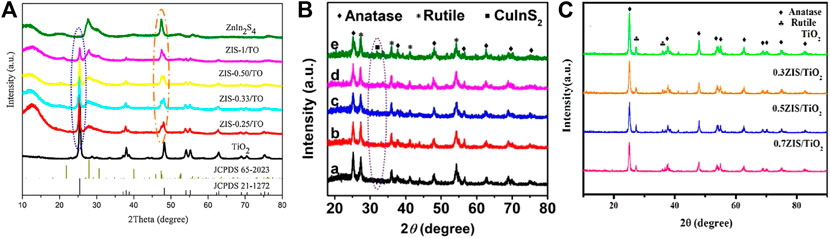
FIGURE 6. XRD patterns of (A) ZnInS2/TiO2 series, (B) CuInS2/TiO2 series and (C) Zn3In2S6/TiO2 series. Reproduced from Yang et al. (2017) and Xu et al. (2018) with permission from Elsevier and Copyright Clearance Center, and She et al. (2018) with permission from Wiley-VCH, respectively.
For a long time, stability is one of the main defects facing the photocatalysts that restricts their long-term performance. The photocatalytic activity decreased continuously in the process of illumination due to photocorrosion. Construction of Z-scheme heterojunction can also protect the narrow band gap semiconductor coupled with TiO2 from photo-oxidation. As reported by Aguirre et al., XPS spectra of Cu2O exhibited an increased Cu(II) content with the extension of illumination time, indicating that Cu(I) was partially oxidized by photogenerated holes (Aguirre et al., 2017). On the contrary, Cu(I) in the Cu2O/TiO2 heterojunction showed no obvious change in valence state, revealing the protection of TiO2 toward Cu2O by injecting photogenerated electrons into the VB of Cu2O to recombine with photogenerated holes there, which also demonstrated the Z-scheme electron transfer mechanism between TiO2 and Cu2O. Interestingly, a stable direct Z-scheme heterojunction can also be formed between TiO2 and metal organic frameworks (MOFs) as PCN-224(Cu) (Wang L. et al., 2019). It is worth nothing that the high specific surface area as well as porous structure of MOFs benefits for CO2 adsorption, while the alternative ligands endow MOFs with adjustable band structure and spectra response range, thus providing a series of promising candidates for the design and construction of direct Z-scheme systems for efficient photocatalytic CO2 reduction. With deepening of the research on the Z-scheme photocatalytic system and the recognition of its photocatalytic performance, more and more different types of Z-scheme photocatalysts have been developed (Raza et al., 2020; Zeng et al., 2020), accelerating the process of photocatalytic CO2 reduction from basic research to practical application.
TiO2 Based S-M Heterojunction for CO2 Photoreduction
As reported by previous literatures, TiO2 modified by metal nanoparticles exhibits enhanced photocatalytic performance due to the promoted charge carrier separation efficiency, expanded light adsorption range as well as high selectivity toward reduction products. In general, Schottky barrier at the interface of semiconductor and metal prevents recombination of photogenerated e−/h+ pairs (Ma et al., 2014; Wang et al., 2014; Ola and Maroto-Valer, 2015; Li et al., 2019). Specifically, the higher work function of metal (Wm) than that of semiconductor (Ws) results in the higher Fermi level of semiconductor (EFs) than that of metal (EFm) (shown in Figure 7A). Contacting semiconductor with metal leads to charge transfer at the interface until the alignment of their Fermi levels. During the process, migration of electrons from semiconductor to metal results in band bending of the semiconductor and creates a space charge region at the interface (Schottky barrier), which could prevent backflow of photogenerated electrons to inhibit their recombination with photogenerated holes (shown in Figure 7B). The promoted charge separation efficiency benefits for photocatalytic CO2 reduction as well as the enhanced water oxidation efficiency. In addition, metals can enrich electrons to create high electron density regions on their surfaces, which are favoring for photoreducing CO2 to hydrocarbons in the presence of water due to their lower redox potential than CO. Besides, local surface plasmon resonance (LSPR) effect of certain metals can enhance visible light adsorption of the Schottky heterojunction and inject hot electrons into the CB of semiconductor, thereby facilitating the photoreduction of CO2 (shown in Figure 7C). In this section, strategies for coupling TiO2 with different metal nanoparticles as well as their different enhancement mechanisms of photocatalytic performance will be reviewed and discussed in detail. Photocatalytic CO2 reduction performance of the typical TiO2-based S-M heterojunctions are listed in Table 3.
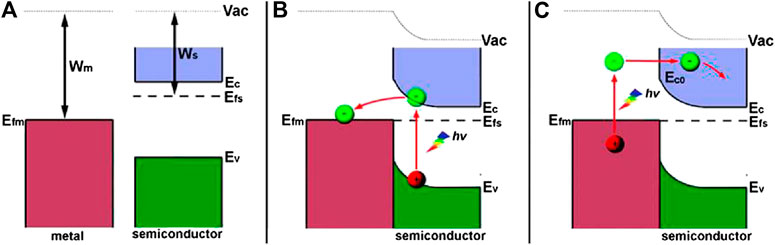
FIGURE 7. Band structure of semiconductor (A) before contact and (B) after contact with metal to form the Schottky barrier; (C) the SPR effect induced hot electron transfer. Reproduced from Bai et al. (2015) with permission from the Royal Society of Chemistry.
As a classic noble metal cocatalyst, Pt has been widely used in the photocatalysis field for both water splitting and CO2 reduction. In particular, TiO2 loaded with Pt nanoparticles has been demonstrated to be efficient for photoreduction CO2 to CH4. As reported by Fresno et al., a series of Pt/TiO2 photocatalysts with different Pt loading amount were fabricated by treating P25 in the Pt precursor-contained aqueous solution via the deposition-precipitation procedure (Tasbihi et al., 2018a). The as-obtained sample (Figure 8A) displays ca. 100% selectivity toward CH4 generation at the optimum Pt content (>0.58 wt% of TiO2), which can be ascribed to the strong chemisorption of CO on Pt nanoparticles (proved by the FTIR spectra in ATR mode (shown in Figure 8B) along with in-situ NAP-XPS analysis) and the further reduction of CO to CH4 (shown in Figure 8C). However, the adsorption features of CO can not be observed on bare TiO2, which yields CO as the main product under the same condition. The correspondingly net selectivities and quantum yield indices (QYI) are shown in Figure 8D. In another work, Pt/TiO2 was synthesized by the hydrolysis of Titanium (IV) butoxide (TEOT) in the presence of H2PtCl6∙6H2O, resulting in the doping of Pt2+ into the lattice of TiO2 and loading of Pt nanoparicles (Pt0) on the surface of TiO2 (Xiong et al., 2015). The low recombination efficiency of photogenetated e−/h+ pairs due to the deposition of Pt0 as well as strong visible light adsorption attributed to Pt2+ doping significantly enhance photocatalytic performance of Pt2+-Pt0/TiO2 with higher quantum yield (1.42%) for CO2 conversion than that of bare TiO2 (0.36%). Moreover, plenty of electrons enriched by Pt0 and protons supplied by water oxidation benefit for the high selectivity toward CH4 formation (Ered/SCE = −0.48 V). Compared with the formation of CO (Ered/SCE = -0.77 V), this reaction is more feasible in thermodynamics. In summary, the kinetic feasibility (strong chemisorption of CO on Pt) and thermodynamic convenience contribute to photoreduction of CO2 to CH4. On this basis, Pt/TiO2 were deposited on porous supports with large surface area (etc. COK-12 (Tasbihi et al., 2018a) and Al2O3 foam (Tasbihi et al., 2018b)) to promote active sites exposure and achieve higher CH4 yield.
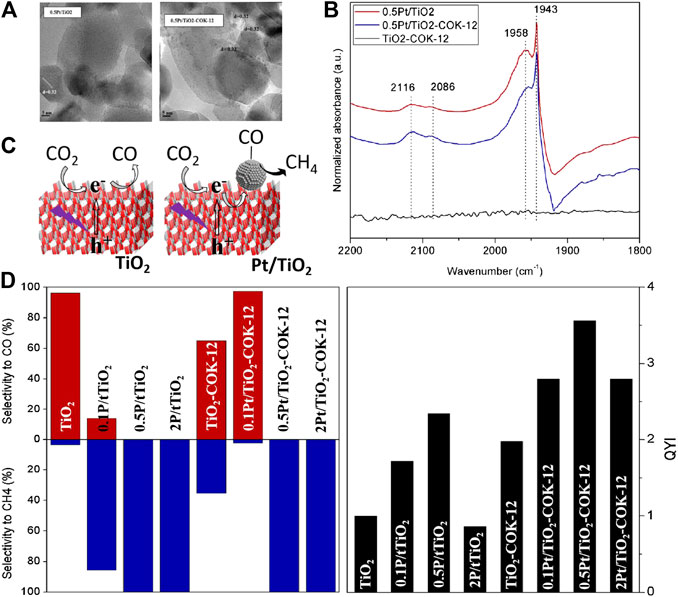
FIGURE 8. (A) TEM images of 0.5 Pt/TiO2 and 0.5 Pt/TiO2-COK-12, (B) FTIR spectra of the TiO2, 0.5 Pt/TiO2 and 0.5 Pt/TiO2-COK-12 catalysts after reaction, in the CO stretching region, (C) Schematic representation of the proposed reaction pathway over TiO2 and Pt/TiO2 catalysts, and (D) Net selectivities and quantum yield indices (QYI) obtained with the different catalysts. The QYI with TiO2 is 1 by definition. Reproduced from Tasbihi et al. (2018a) with permission from Elsevier and Copyright Clearance Center.
Coupling TiO2 with noble metals that can induce the LSPR effect has also been adapted by researchers for efficient photocatalytic CO2 reduction. As reported by Wang et al., 0D/2D Au/TiO2 was synthesized by in situ growth of Au nanoparticles on the surface of TiO2 nanosheets via chemically reduction (Wang R. et al., 2019). The hot electrons induced by the LSPR effect of Au under visible light irradiation (550 nm) could inject into the CB of TiO2 and reduce CO2 to CO. However, as the only electron source (TiO2 can not be excited by visible light), the limited hot electrons cannot further reduce CO to CH4. Interestingly, the Au/TiO2 hybrid yielded CH4 as the main product under 300 W Xe lamp irradiation that contained a certain amount of UV light. Specifically, recombination of photogenerated electrons and holes was suppressed owing to the Schottky barrier that facilitated transfer of e− from the CB of TiO2 to Au. Moreover, h+ remained in the VB of TiO2 could oxidize water to provided plenty of protons for CH4 generation. On this basis, facet engineering was introduced by Wang et al. for the rational design of interface between Au and exposed facet of TiO2 to realize higher charge separation efficiency (Wang et al., 2021). Results showed that the lower height of Schottky barrier on the Au/TiO2{101} interface resulted in more smooth migration of photogenerated electrons from the CB of TiO2 to Au compared to the Au/TiO2{001} interface, thereby exhibiting better performance for photocatalytic CO2 reduction. Notably, the architecture of TiO2 among Au/TiO2 heterojunction also plays an important role for efficient photoreduction of CO2 (Jiao et al., 2015; Khatun et al., 2019). Jiao et al. (Jiao et al., 2015) prepared 3D ordered macroporous (3DOM) TiO2 to support Au nanopaticles, which were uniformly dispersed in the inner wall of the 3DOM structure. The multiple scattering of incident light within the 3DOM structure enhanced light utilization efficiency of the heterojunction, while the ordered macroporous also improved the mass transfer efficiency of the reactants. In addition, the SPR effect of Au induced by visible light irradiation provided extra electrons for photocatalytic CO2 reduction, which was benefited for CH4 generation. In another work, electrochemical anodization was used to fabricate TiO2 nanotubes (TNTs) with light adsorption edge in the visible region, indicating its weak photocatalytic activity illuminated by visible light (Khatun et al., 2019). Coupling with Au by electronchemical deposition significantly improved visible light adsorption of TNTs and promoted charge separation efficiency due to the LSPR effect of Au nanoparticles. The excellent CH4 yield (14.67% of CO2 was converted to CH4) under visible light irradiation made Au-TNTs a very promising solar-driven photocatalyst to convert CO2 into hydrocarbon fuels. Similarly, plasmonic Ag were electronchemical deposited into the inner space of TiO2 nanotube arrays (Figure 9A) to investigate the enhancement of SPR effect on photocatalytic performance, while the morphology and structure of as-obtained Ag-TNTAs-E are shown in Figures 9B,C (Low et al., 2018). The direct evidences of the existence of Schottky barrier between Ag and TiO2 as well as migration of hot electrons induced by the SPR effect of Ag nanoparticles can be found in the synchronous-illumination X-ray photoelectron spectroscopy (SIXPS) spectra based on the shift of Ti 2p3/2 peak before and after illumination (Figures 9D,E). Moreover, the SPR effect of Ag nanoparticles was strengthened by the multiple scatted light in TNTAs (Figure 9F), while the derived near field effect accelerated charge transfer at the heterointerface to promote separation efficiency of photogenerated e−/h+ pairs, thereby endowing enhanced VLD activity of Ag-TNTAs for CO2 photoreduction. Although promoting photoreduction efficiency of CO2 and clarifying the involved mechanisms are the focus of current research, the improvement of photocatalyst synthesis methods also deserves attention. The silver mirror reaction was adopted by Yu et al. to deposited Ag on TiO2 nanoparticles (Yu et al., 2016). CH3OH generated in CO2-saturated 1 M NaHCO3 solution is the main product of photocatalytic CO2 reduction, which is more valuable than the primary products such as CO and CH4. In another work, Ag(I) adsorbed by TiO2 nanorod arrays were completely reduced by cold plasma within 30 s to form uniformly distributed Ag nanoparticles (Cheng et al., 2017). This fast and efficient strategy is very promising for the fabrication of metal nanoparticles decorated semiconductor photocatalysts on a large scale.
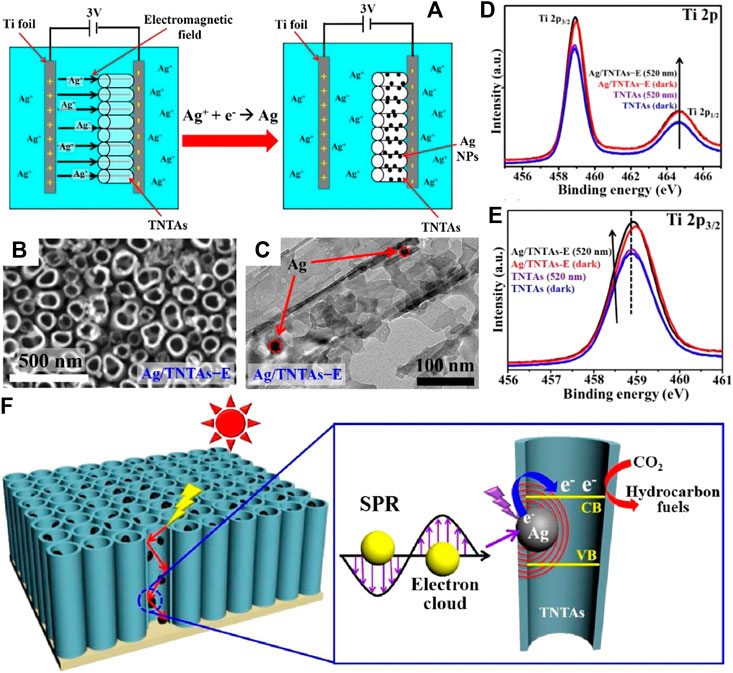
FIGURE 9. (A) Schematic illustration of electrochemical deposition methods for loading Ag NPs into the TNTAs, (B) SEM image (top view) and (C) TEM image (side view) of Ag/TNTAs-E, Comparison of high-resolution SIXPS spectra of (D) Ti 2p and (E) Ti 2p3/2 for TNTAs and Ag/TNTAs-E in the dark and under 520 nm LED light irradiation, and (F) Schematic illustration of the enhanced SPR effect of Ag NPs in the TNTAs structure. Reproduced from Low et al. (2018) with permission from Elsevier and Copyright Clearance Center.
Bimetallic nanoalloys that combined advantages of the two metal components are efficient cocatalysts for photocatalytic CO2 reduction and have been introduced in the TiO2-based photocatalytic systems. As reported by Neaţu et al., Au and Cu species were deposited on TiO2 nanopartcles stepwisely followed by calcining in H2 atmosphere to form Au-Cu alloy (Neaţu et al., 2014). In this case, Au is served as visible light harvester due to its LSPR effect while Cu can covalently bind with CO reduced from CO2 and direct the generation of CH4. Therefore, high VLD photocatalytic activity with outstanding CH4 selectivity (97%) was achieved. Other bimetallic nanoalloys, such as Au-Ag (Tahir et al., 2017), Au-Pd (Ziarati et al., 2020), Ag-Pd (Tan et al., 2018) and Pt-Ru (Wei Y. et al., 2018) nanoparticles are also been used to enhance photocatalytic performance of TiO2 for selective reduction of CO2. Among them, the combination of bimetallic nanoalloys and modified TiO2 (etc. hydrogenated black TiO2 (TiO2-xHx) (Ziarati et al., 2020) and N-doped TiO2 (Tan et al., 2018)) exhibited considerably enhanced visible light utilization and charge separation efficiency, which could become the future development trend of TiO2-based S-M heterojunction for solar-driven CO2 photoreduction. Moreover, construction of S-M heterojunction with hierachical architecture is also in great demand (Ziarati et al., 2020).
TiO2 Based S-C Heterojunction for CO2 Photoreduction
Recently, coupling TiO2 with carbon-based nanomaterials including graphene and its derivatives (etc. graphene (GR) (Tu et al., 2013; Xiong et al., 2016; Biswas et al., 2018; Jung et al., 2018; Shehzad et al., 2018; Zhao et al., 2018; Zubair et al., 2018; Bie et al., 2019), graphene oxide (GO) (Chowdhury et al., 2015; Tan et al., 2017) and reduced graphene oxide (rGO) (An et al., 2014; Kuai et al., 2015; Sim et al., 2015; Tan et al., 2015; Lin et al., 2017; Olowoyo et al., 2019)), carbon nanotubes (CNTs) (Xia et al., 2007; Gui et al., 2014; Gui et al., 2015; Olowoyo et al., 2018; Rodríguez et al., 2020) and carbon quantum dots (CQDs) (Li et al., 2018; Wang K. et al., 2019) to construct TiO2-carbon heterojunction for photocatalytic reduction of CO2 has been widely concerned. The unique physicochemical properties of nanocarbon that responsible for the enhanced photocatalytic performance of the S-C heterojunction can be concluded as follows: 1) the large surface area and high mechanical stability of nanocarbon could provide a stable support for the uniformly distributed TiO2 nanoparticles with increased exposure of active sites and enhanced CO2 adsorption capacity; 2) the high charge carrier mobility, large capacitance of nanocarbon as well as the formation of Ti-O-C bond at the highly dispersed S-C interface facilitates the migration of electrons from TiO2 to carbon materials, thereby enhancing the separation efficiency of photogenerated e− and h+ and inhibiting their recombination; 3) the optical properties of carbon materials, such as good optical transparency and wide spectrum adsorption range (especially for CQDs, expands to near IR region), contribute to the utilization of visible light of the TiO2-based S-C heterojunction and result in the improved quantum efficiency. In this section, S-C heterojunctions including TiO2-GR series, TiO2-CNT and TiO2-CQDs are reviewed and discussed in detail, respectively. Photocatalytic CO2 reduction performance of the typical TiO2-based S-C heterojunctions are listed in Table 3.
Coupling TiO2 With Graphene and Its Derivatives
Construction of TiO2-carbon heterojunction using graphene or its derivatives as the guest/host component derives improved photocatalytic performance due to its excellent electrical properties and chemical stability. It is worth nothing that the path of graphite-GO-rGO has been generally adopted by researchers to obtain graphene, whereas various of strategies have been developed for the fabrication of TiO2-graphene nanocomposites. As reported by Tu et al., in situ simultaneous reduction-hydrolysis technique was developed for the fabrication of TiO2-graphene 2D sandwich-like hybrid nanosheets (Tu et al., 2013). During the process, GO was reduced to graphene (rGO) by ethylenediamine (En) while Ti (IV) was hydrolyzed to TiO2 nanoparticles and loaded on rGO through Ti-O-C bonds. The abundant surface Ti3+ sites generated from En reduction could trap photogenerated electrons efficiently, thereby decreasing the recombination efficiency of charge carriers. Moreover, the synergism of Ti3+ sites and garphene favors for the generation of C2H6, which is inspiring for C-C coupling during the photoreduction process of CO2. In another work, the suspension of GO and TiO2 in ethanol was ultrasonicated and refluxed to form Ti-O-C bonds, while GO was partially reduced to rGO during the process (Shehzad et al., 2018a). The tightly connected two phases improve charge separation at the heterointerface, while the enlarged light absorption coefficient is attributed to the reduced bandgap energy by the formation the Ti-O-C bonds. As a result, the rGO/TiO2 nanocomposites exhibited greater yields of CH4 (12.75 μmol gcat−1 h−1) and CO (11.93 μmol gcat−1 h−1) than anatase for 4 folds. Theoretical calculation was applied by Olowoyo et al. to investigate the enhanced photocatalytic performance of rGO/TiO2 in reducing CO2 (Olowoyo et al., 2019). Results reveal that the high electron density of rGO has significant influence on the TiO2 bands and endows visible light responsibility of the composite. Moreover, the different electron migration paths within rGO/TiO2 under different light sources were observed. Compared to the electron transfer from TiO2 to rGO under UVA, irradiation by visible light leads to the direct generation of electorns and holes in rGO or TiO2, respectively. Both of the two pathways are efficient for photogenerated charge separation and favor for methanol production. In addition, the large adsorption capacity of CO2 is another feature of TiO2/graphene that contribute to photocatalytic CO2 reduction (Chowdhury et al., 2015). As reported by Chowdhury et al., TiO2/GO nanocomposites was obtained from the aqueous suspension of GO and TiO2 under ultrasonication flowed by continuous stirring. The synergism of physisorption (intermolecular electrostatic interactions as van der Waals forces or London dispersion forces) and chemisorption (coordination of O atoms with surface Lewis acid center (Ti sites), or coordination of C atom with surface Lewis acid center (oxygen functionalities of GO or TiO2)) led to the high adsorption amount of CO2 (1.88 mmol g−1), which facilitated its activation and photocatalytic reduction. Considering that photoreduction of CO2 requires the participation of electrons and protons produced by photooxidation of H2O, the adsorption capability of H2O molecule by photocatalyst is also critical. In view of the compete adsorption of H2O and CO2 on the active sites, proper partial pressures of CO2 and H2O are in great demand that determines the CH4 yield on GO-doped oxygen-rich TiO2 (Tan et al., 2017). Notably, the CO2 adsorption capacity and charge separation efficiency can be further improved by introducing nitrogen dopants into rGO to form NrGO/TiO2 system (Lin et al., 2017). On the one hand, the positive electrostatic potential regions created by nitrogen dopants on the surface of NrGO benefit for CO2 adsorption and activation. On the other hand, the injection of electrons from quaternary-N species existed in NrGO matrix to the delocalized π-system facilitates the transfer of electrons and inhibits the recombination of photogenerated e−/h+ pairs. These findings are very helpful for the design of photocatalytic system based on heteroatom-doped graphene for CO2 conversion with high efficiency. Furthermore, construction of well-defined nanostructure offers another thought to improve photocatalytic performance of TiO2/graphene heterojunction (Zubair et al., 2018). Typically, TiO2 nanotube arrays (TNT) with attractive 1D vectorial charge transfer can suppress recombination of photogenerated charge carriers efficiently. Graphene quantum dots (GQDs) decorated on TiO2 nanotube (G-TNT) promotes charge transfer at the heterointerface, which could also enhance the light utilization of the heterojunction due to its superior visible light responsibility. Besides, the high surface area of the heterojunction contributes to the exposure of active sites that favor for CO2 adsorption and activation. Consequently, a 5.6 fold CH4 yield was obtained on G-TNT in comparison with pure TNT. On this basis, Pt nanoparticles were employed for the construction of rGO/Pt-TNT ternary composite with promoted visible light responsibility and photoreduction selectivity of CO2 to produce CH4 (Sim et al., 2015). In particular, the LSPR effect of Pt expands light adsorption range of the heterojunction to 450 nm that can be activated by visible light. Similarly, the high selectivity for CH4 generation (99.1%, compared to CO yield) was also proved by Zhao et al. on (Pt/TiO2)@rGO system (Zhao H. et al., 2018), in which vectorial electron transfer from TiO2 to rGO through Pt was also demonstrated.
Coupling TiO2 With CNT
Generally, charge transfer along the 1D CNT leads to high separation efficiency of photogenerated e−/h+ pairs and endows superior photocatalytic performance of TiO2/CNT heterojunction. Moreover, CNT can also serve as support to reduce the aggregation of TiO2 nanoparticles, thus resulting in the formation of highly dispersed heterointerface and large exposure of active sites. As reported by Xia et al., the multi-walled CNT (MWCNT)/TiO2 hybrid fabricated via sol-gel method yielded C2H5OH as the main photoreduction product of CO2 under UV light irradiation (Xia et al., 2007). In a further study, MWCNT/TiO2 with core-shell nanostructure was demonstrated to be visible light active (due to the excellent visible light adsorption ability of CNT) that can convert CO2 to CH4 (Gui et al., 2014). A following work carried out by this group introduced Ag nanoparticles to MWCNT/TiO2 system (Ag-MWCNT@TiO2) for the further enhanced photocatalytic performance (Gui et al., 2015). In particular, the Schottky barrier between Ag and TiO2 prevents backflow of photogenerated electrons that transferred from TiO2 to Ag. The synergism of MWCNT and Ag nanoparticles greatly enhances separation efficiency of photogenerated charge carriers and restricts their recombination, thereby resulting in higher CH4 formation rate (0.91 μmol gcat−1 h−1) in comparison with the binary system (MWCNT@TiO2, 0.17 μmol gcat−1 h−1). Further studies revealed the mechanism of electron transfer between TiO2 and CNT (Olowoyo et al., 2019). Specifically, strong attachment between MWCNT and the {101} facet of anatase TiO2 introduces common orbitals within the band gap of TiO2, which is the fundamental of charge transfer between the two phases and enables visible light responsibility of the composites. Electron transfer from TiO2 with higher density of initial states to MWCNT under irradiation of both UVA and visible light is more probable basing on the computation results. In addition, the tight contact between MWCNT and TiO2 due to the combination of sonothermal and hydrothermal methods results in extremely high photocatalytic activity with CH3OH generation rate of 2360 μmol gcat−1 h−1, much higher than that of the similar photocatalytic systems. A recent study reported the fabrication of TiO2/CNT composite in the medium of supercritical CO2 (Rodríguez et al., 2020). Although photocatalytic activity of the product is relatively weak (CO, 8.1 μmol gcat−1 h−1; CH4, 1.1 μmol gcat−1 h−1), it can also provide a novel strategy for the synthesis of TiO2-based photocatalyst and leave a large space for performance improvement.
Coupling TiO2 With CQDs
As a new class of 0D carbon-based nanomaterial, the excellent photoelectric properties of CQDs, such as wide spectral response range, photo-induced charge transfer ability, up-conversion function and anti-photocorrosion property, make it a promising cocatalyst to enhance photocatalytic performance of traditional semiconductor photocatalyst as TiO2 (Li et al., 2018; Wang K. et al., 2019). As reported by Li et al., N, S-containing CQDs (NCQDs) was synthesized via a microwave-assisted method using thiourea and citric acid as precursors, which then assembled with P25 under continuous stirring at 80°C to form NCQDs-TiO2 nanocomposites. Particularly, TiO2 sensitized by NCQDs can be activated by visible light, while electrons transferred from rutile TiO2 in P25 to NCQDs prevented recombination of photogenerated e−/h+ pairs, thus enhancing photoreduction efficiency of CO2. Although the photoelectric properties of CQDs are attractive, relative research about coupling CQDs with TiO2 or other semiconductors to construct heterostructured photocatalyst for photocatalytic CO2 reduction is still few. In our opinion, as an important characteristic distinguished from other carbon-based materials, up-conversion function of CQDs is worth developing to improve the light energy utilization of heterostructured photocatalysts, especially for the wide band gap semiconductor-contained systems.
In addition to carbon-based nanomaterials analyzed above, other carbon forms can also combine with TiO2 to obtain heterojunction for photocatalytic CO2 reduction with high efficiency. For instance, Zhang et al. coated TiO2 on electrospun carbon nanofibers to promote active sites exposure as well as charge separation and transfer of the nanocomposites (Zhang J. et al., 2018). Besides, the heat produced by carbon nanofibers due to its photothermal conversion function accelerates the diffusion kinetics of reactants and products during photocatalytic process, thus further enhancing the photoreduction efficiency of CO2. The local photothermal effect induced by carbon species is also highlighted by Wang et al. among the hybrid carbon@TiO2 hollow spheres (Wang et al., 2017). Moreover, the multiple scattering of incident light within the hollow structure improves light utilization of the hybrid (shown in Figure 10) and contributes to improve the quantum efficiency of photocatalytic CO2 reduction. This result indicates that not only component, but also architecture of the heterojunction photocatalysts plays important role in improving photocatalytic performance.
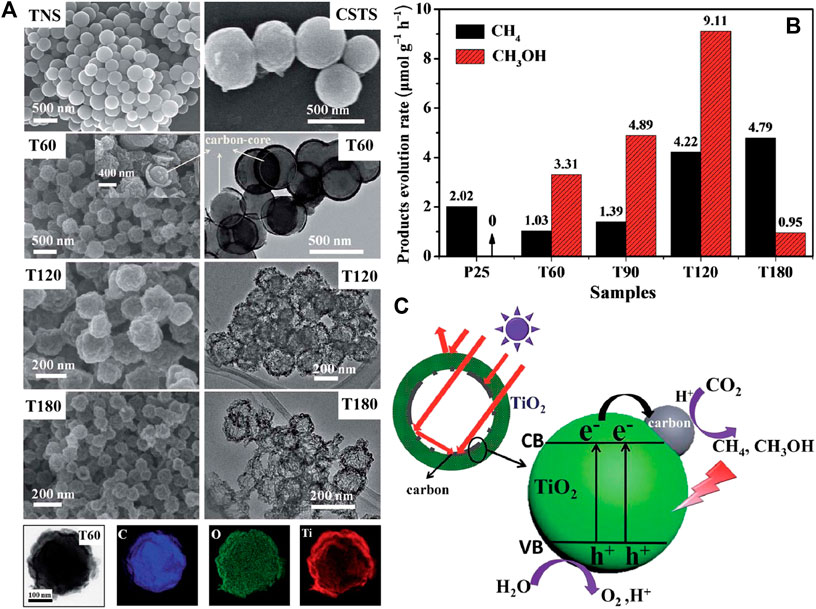
FIGURE 10. (A) FESEM images of TNS, CSTS, T60, T120 and T180, and TEM images of T60, T120 and T180, and STEM image of T60 and the corresponding elemental mapping images of C, O and Ti; (B) Comparison of the photocatalytic CH4 or CH3OH evolution rate of carbon@TiO2 composite samples and P25 (under simulated solar light); and (C) Photoexcitation process of the carbon@TiO2 composite photocatalyst with hollow structure. Reproduced from Wang et al. (2017) with permission from the Royal Society of Chemistry.
TiO2 Based Multicomponent Heterojunction for CO2 Photoreductions
Construction of TiO2-based multicomponent heterojunction to introduce two different functional co-catalysts for efficient VLD photocatalytic CO2 reduction has been widely adopted, in which TiO2 combined with any two of another semiconductor (AS), metal nanoparticles (MNPs) and nanocarbon (C) to form ternary composites is currently the most studied system. Previous research revealed the highest selectivity of Pt for CH4 generation compared to other noble metal cocatalysts (Pt > Pd > Au > Rh > Ag) due to its excellent electron extraction ability that derives high electron density around it and facilitates CO2 photoreduction (Xie et al., 2014). However, the consequent increase in H2 production is unfavorable and should be suppressed to realize further enhanced photoreduction efficiency of CO2. Xie et al. coated MgO amorphous layers on Pt/TiO2 hybrid to improve chemisorption of CO2, which was then reduced to CH4 directly by photogenerated electrons enriched on adjacent Pt nanoparticles with high efficiency, thus benefiting for the selective formation of CH4. The similar function of Cu2O was demonstrated by Xiong et al. from the Pt-Cu2O/TiO2 nanocomposite (Xiong et al., 2017c). Notably, the charge separation efficiency of the ternary system decreased with the increasing amount of MgO, indicating that excess MgO may restrict electrons transfer from TiO2 to Pt. Therefore, TiO2/MNPs/AS ternary heterojunctions with rational designed architecture and efficient carrier migration path are necessary. According to Meng’s research, MnOx and Pt were selectively deposited on the {001} and {101} facet of TiO2 (Figure 11A,B,C), respectively (Meng et al., 2019). The series connection of S-M (Pt and TiO2{101}), facet (TiO2{101} and {001}) and p-n (TiO2{101} and MnOx) heterojunction accelerated migration of photogenerated electrons along the path of MnOx→TiO2{001}→TiO2{101}→Pt while photogenerated holes in the opposite direction (shown in Figure 11D,F). As a result, the separation efficiency of photogenerated charge carriers is greatly improved, so as to the enhanced photocatalytic performance with CH4 and CH3OH as the main products. In another work, Z-scheme heterojunction that is favoring for the recombination of inefficient charge carriers was constructed by coupling TiO2 and ZnFe2O4 using Ag as electron mediator (Tahir, 2020). Superior CO yield (1025 μmol gcat−1 h−1) accompanied with the generation of CH4 (132 μmol gcat−1 h−1) and CH3OH (31 μmol gcat−1 h−1) should be attributed to the enhanced charge separation efficiency under UV light irradiation. It is worth nothing that the magnetic properties of ZnFe2O4 should not be ignored which facilitate the recovery of photocatalyst from solid-liquid suspension, although the solid-gas mode is undertaken in this study. Moreover, Ag could also promote visible light adsorption of the ternary system due to its strong LSPR effect, which had been demonstrated by Xu et al. using MgO-Ag-TiO2 as photocatalyst (Xu and Carter, 2019). In order to further investigate the synergism of the LSPR effect and chemisorption of CO2 on the improvement of photoreduction efficiency of CO2, TiO2/MNPs/AS heterojunctions as Au/Al2O3/TiO2 (Zhao Y. et al., 2018) and Au@TiO2 hollow spheres (THS)@CoO (Zhu et al., 2019) were synthesized. On this basis, MgAl layered double oxides (MgAl-LDO) were developed to provide both Lewis basic sites (MgO) and Lewis acid sites (Al2O3) for CO2 chemisorption and H2O dissociation among the Pt/MgAl-LDO/TiO2 nanocomposite, respectively (Chong et al., 2018). Benefiting from the generation of monodentate carbonate (m-CO32-) on the surface of MgO accompanied with the supply of H+ from H2O dissociation by Al2O3, the activation of CO2 became easier and led to the increased CH4 yield by 11 folds compared to Pt/TiO2.
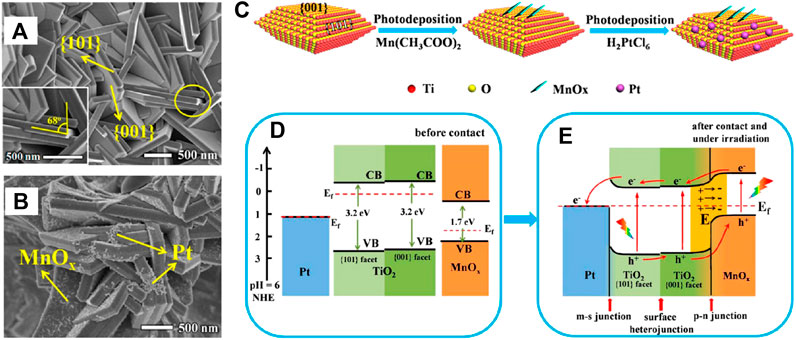
FIGURE 11. SEM images of as-prepared (A) TiO2 and (B) TiO2-MnOx-Pt (TMP); (C) Schematic diagram of selective photodeposition process of MnOx nanoflakes and Pt nanoparticles on anatase TiO2 {001} and {101} facets; Schematic diagram of proposed photocatalytic CO2 reduction mechanism of sample TMP. The relative band energy positions of TiO2, Pt, and MnOx(A) before contact and (B) after contact and under irradiation. Reproduced from Meng et al. (2019) with permission from the American Chemical Society.
In a typical TiO2/C/AS system, nanocarbon is served as electron channel to guide photogenerated electrons flow from TiO2 to the AS while photogenerated holes left in the VB of TiO2, thereby resulting in the spatial separation of photoinduced redox reactions with enhanced CO2 photoreduction efficiency. As reported by Jung et al., mesoporous TiO2 and a few layers of MoS2 were assembled with graphene aerogel via one-pot hydrothermal method to construct a 3D hierarchical structure (Jung et al., 2018). In addition to high separation efficiency of photogenerated e−/h+ pairs, the improvement of light utilization and mass transfer efficiency based on the 3D structure of graphene with efficient visible light adsorption (Biswas et al., 2018) is also important factor that contribute to photocatalytic CO2 reduction. Compared to semiconductors as MoS2 (Jung et al., 2018), CuGaS2 (Takayama et al., 2017) or WSe2 (Biswas et al., 2018), noble metal nanoparticles are more efficient for the accumulation of photogenerated electrons transferred through electron channel (graphene) and favoring for the generation of more valuable products as CH4 and CH3OH (Tan et al., 2015; Meng et al., 2019; Tahir, 2020). However, the high cost as well as relative low resistance toward photocorrosion restricts their application on a large scale. Photocatalytic CO2 reduction performance of the typical TiO2-based multicomponent heterojunctions above are listed in Table 3. In the future, the development of noble metal-free multicomponent heterojunction based on TiO2 for efficient solar-driven photocatalytic CO2 reduction will become one of the main directions in this field.
TiO2 Based Phase and Facet Heterojunctions for CO2 Photoreduction
TiO2 Based Phase Heterojunction
Phase heterojunction composed of different crystal phases of the same semiconductor exhibits greater photocatalytic activity than the single-phased photocatalyst (Ma et al., 2014; Wei L. et al., 2018; Nguyen et al., 2020). This is because the contact of crystal phases with different energy band structure leads to an increase in carrier separation efficiency at the interface. Moreover, the unique interfacial trapping sites may become new photocatalytic active sites. As is known, there are four main crystal phases of TiO2 (including anatase, rutile, brookite, and TiO2 (B)) existed in nature, in which rutile is the most thermodynamically stable phase and can be obtained by calcining the other three polymorphs (Ma et al., 2014). Among them, as the two most widely studied TiO2 crystal phases with photocatalytic activity, the difference in the lattice structure of anatase and rutile leads to different electronic band structures, and ultimately results in a difference in band gap width. Compared to rutile (Eg = 3.02 eV for bulk material), anatase tends to show higher photocatalytic activity due to its wider band gap (Eg = 3.20 eV for bulk material) that gives stronger redox ability to the photogenerated carriers. In addition, the higher concentration of oxygen vacancies in anatase leads to more efficient charge separation, whereas the larger specific surface area leads to more active sites exposure, which are also important factors for its better photocatalytic performance than rutile. However, the narrower band gap enables rutile to respond to photons close to the visible region. Besides, the higher crystallinity results in the better charge carrier mobility within rutile. At present, the integration of the advantages of anatase and rutile to construct a phase heterojunction for photocatalytic CO2 reduction has attracted increasing attention of researchers. As a classic anatase-rutile phase heterojunction, the commercial Degussa P25 has been regarded as a benchmark for both photocatalytic oxidation and reduction reactions. As reported by Reñones et al., hierarchical TiO2 nanofibres composed of anatase and rutile nanoparticles were synthesized by the calcination of electrospun TiO2 fibers under Ar atmosphere (Reñones et al., 2016). The phase composition of the fibers depend on the calcination conditions, in which higher rutile amount (81:19) was obtained under static Ar atmosphere (Fibers B) than the dynamic sample (Fibers A, 93:7). Notably, the faster charge transport along the grain boundaries in fibers is attributed to the improved nanocrystals connection, whereas the fibers with higher anatase content exhibits lower recombination efficiency of e-/h+ pairs, thus endowing greater photocatalytic efficiency for reducing CO2 to CO (10.19 μmol gcat−1 h−1). In addtion, the overall apparent quantum yields (AQY) of Fibers B (0.036%) is also higher than P25 (0.030%), indicating the enhanced utilization of incident light. However, the large amount of hydrogen evolution (19.94 μmol gcat−1 h−1) during the photocatalytic process needs to be suppressed to further improve the photoreduction efficiency of CO2. A hydrothermal method was developed by Xiong et al. for the fabrication of anatase-rutile heterophase TiO2 nanoparticles using K2TiO(C2O4)2∙2H2O as Ti source, which simplifies the synthesis process and makes the reaction conditions more mild (Xiong et al., 2020). Ethylene glycol (EG) was added to the hydrothermal system in order to introduce oxygen vacancy into TiO2 (TiO2-x), which could trap photogenerated e- to restrain the recombination of e−/h+ pairs, thereby enhancing the photocatalytic performance of the catalysts. Electron paramagnetic resonance (EPR) spectra revealed that the concentration of oxygen vacancy increased with the increasing amount of EG during the formation process of TiO2-x. However, the excess oxygen vacancies, especially the bulk oxygen vacancies, will act as the recombination center of carriers, resulting in the lower photocatalytic efficiency of as-obtained anatase-rutile heterojunction. Thus, the concentration and distribution of oxygen vacancies should be reasonably designed and constructed to promote the performance of the semiconductor photocatalysts. As reported by Xiong et al. (Xiong et al., 2020), the TiO2-EG10 sample synthesized using 30 ml H2O and 10 ml EG as solvent exhibits the optimized photocatalytic activity in reducing CO2 to CH4 (43.2 μmol gcat−1 h−1), which is 54 times higher than that of P25 (0.8 μmol gcat−1 h−1). Usually, the optimization of oxygen vacancy concentration in photocatalyst is based on the feedback of experimental results rather than theoretical design. Therefore, the critical oxygen vacancy concentration in different photocatalytic systems is various, which is difficult to give a definite value. Considering the poor visible light responsibility of anatase/rutile phase heterojunction, it is necessary to reduce its band gap width to improve the utilization of incoming solar spectrum. In addition, the combination of disordered anatase (Ad) with more active sites and ordered rutile (Ro) for fast transport of e- and h+ to suppress charge recombination can further enhance the photoreduction efficiency of CO2 by TiO2. On this basis, a phase-selective Ad/Ro TiO2 was prepared by treating P25 in the sodium alkyl amine solutions at room temperature and ambient atmosphere, in which anatase among P25 was selective reduced to produce more Ti3+ defects (Hwang et al., 2019). The existence of multi-internal energy bands of Ti3+ defect sites in Ad reduces the band gap of Ad/Ro TiO2 to 2.62 eV, while the newly conduction band (-0.27 eV) is well match the redox potential of CO2/CH4 (-0.24 V vs. NHE). As a result, the VLD Ad/Ro TiO2 exhibits enhanced reactivity to convert CO2 into CH4 (3.98 μmol gcat−1 h−1), which is higher than metal (W, Ru, Ag, and Pt)-doped P25. To further improve the CH4 generation selectivity, 0.1% mass Pt was loaded on the H2O2 modified TiO2 (M-TiO2, containing two phases of anatase and rutile) through photoelectrodeposition (Pt/M-TiO2) (Lee J. S. et al., 2016). As electron sinks, photogenerated electrons are enriched by Pt nanoparticles and form high charge density areas near their surface, which is favoring for the photoreduction of CO2 to CH4 in cooperation with sufficient protons generated by water oxidation. Notably, the yield of CH4 on Pt/M-TiO2 is about 60 times that of bare M-TiO2, whereas no CO formation can be observed, indicating its high selectivity toward photoreduction products of CO2. Compared with the common method that fabricates phase heterojunction by calcining TiO2 gel, the MOFs (NH2-MIL-125) derived method was adopted by Chen et al. (Chen et al., 2020) to fabricate anatase-rutile junction with large surface area and porous structure that favored for CO2 adsorption. Besides, the in situ phase transformation from anatase (211) plane to rutile (211) plane results in the highly dispersed anatase/rutile interface for efficient interfacial charge separation, inhibiting the recombination of photogenerated e-/h+ pairs significantly. Moreover, the N-doped carbon layer (generated by the pyrolysis of organic ligands) coating on the anatase/rutile heterostructure promotes the electric conductivity of the photocatalytic system, and expandes its light absorption range to 700 nm. The synergism of N-doped carbon and paragenetic anatase/rutile heterostructure derives the enhanced photoreduction efficiency of CO2 to CO, which is 7.6 folds compared with P25. Although the added value of the product (CO) is limited, this method has guiding significance for the design and synthesis of other MOFs derived heterostructured photocatalytic systems. In addition to rutile, anatase can also form a phase heterojunction with brookite for photocatalytic CO2 reduction (Jin et al., 2019). Generally, Sr2+ ions were introduced to the TiCl4-involed hydrothermal system for the fabrication of SrCO3-modified brookite/anatase TiO2 heterophase junctions (HPJs). Similar to the anatase-rutile system, the interfacial electron transfer from brookite to anatase promotes the photogenerated charge separation. Moreover, the surface modification of HPJs by SrCO3 can improve the adsorption of CO2/H2O, which can also serve as an efficient cocatalyst for the selective reduction of CO2 to CH4. Especially, 1.0 w/w% SrCO3/HPJs composite shows the selectivity of ca. 7.45 for CH4/CO (19.66/2.64 μmol gcat−1 h−1), which is ca. 32.4 folds compared with pristine brookite TiO2 (0.79/3.46 μmol gcat−1 h−1). This work can also provide guidance for the development of heterojunctions composed of TiO2 HPJs and alkaline earth metal carbonates via a facile one pot hydrothermal route.
TiO2 Based Facet Heterojunction
The difference of geometrical and electronic structures between different crystal facets of the same semiconductor results in the distinctness of their photocatalytic activity. Facet engineering has been applied to control the exposed crystal facet of semiconductors, in order to increase the exposure of active sites and promote the adsorption and activation of substrates, so as to achieve enhanced photocatalytic performance. In terms of anatase TiO2, the {101} has the lowest surface energy (0.44 J m−2) among the low-index facets (including {001}, {010} and {101}) and dominant for CO2 adsorption basing on first-principles calculations (Yu et al., 2014). The photogenerated electrons transferred from the {101} of TiO2 to CO2 facilitates its activation and reduction. Moreover, the enriched photogenerated holes on the {001} of TiO2 can accelerate the oxidation reactions. Photocatalytic CO2 reduction over anatase TiO2 with coexposed {001} and {101} facets was reported by Yu et al. for the first time with the propose of “facet heterojunction” concept (Yu et al., 2014). The facet ratio of {001} and {101} can be tuned by adjusting the amount of HF during the fabrication process, as see in Figures 12A,B, while the correspondingly schematic illustration is displayed in Figure 12C. In particular, the formation of facet heterojunction between {001} and {101} contributes to the transfer and separation of photogenerated carriers, which is beneficial to the enrichment of e− and h+ on {101} and {001}, respectively (shown in Figure 12D). As a result, photocatalytic CO2 reduction occurs selectively on the {101} facet, while the optimized photocatalytic performance is realized when the facet ratio of {101} to {001} is 45–55, in which CO2 is reduced to CH4 with a generation rate of 1.35 μmol gcat−1 h−1 (Figure 12E). On this basis, oxygen vacancies were introduced to the {101} and {001} facets coexposed anatase TiO2 for the further enhancement of photocatalytic performance, where TiO2 fabricated via hydrothermal route in the presence of HF was reduced by NaBH4 to generate surface oxygen defects (Liu L. et al., 2016). In addition to the high charge separation efficiency at the interface of {001} and {101} facets, the visible light responsibility attributed to the newly generated Ti3+ energy state as well as the improved CO2 adsorption and activation derived from the synergism of Ti3+ and oxygen vacancies resulted in the enhanced photoreduction efficiency of CO2. An explicit atomistic model of the interface was applied for further investigation of charge transfer between coexposed {101} and {001} facets of anatase TiO2 (Di Liberto et al., 2019). The first principles calculations revealed that the localization of h+ on oxygen ion of the {001} side and the migration of e− to Ti ion of the {101} side promoted the charge separation and suppressed their recombination, hence responsible for the enhanced photocatalytic activity of the facet junction. In another work, HF was also used by Cao et al. (Cao et al., 2016) for tuning the ratio of coexposed {101} and {001} facets of anatase TiO2 in a hydrothermal system where nanotube titanic acid (NTA) was used as precursor to facilitate the mass transfer of HF, thereby simplifying the generation of {001} facet. Notably, the anatase TiO2 nanocrystals with coexposed 51% {001} and 49% {101} facets exhibit the highest photocatalytic activity for reducing CO2 to CH4 (1.58 μmol gcat−1 h−1) among pure TiO2 series, which can be further improved to 4.0 μmol gcat−1 h−1 by decorating 1wt% Pt0 nanoparticles on their surfaces. The effect of Pt loading for the enhanced photocatalytic performance of anatase TiO2 facet heterojunction ({101}/{001}) was further investigated by Xiong et al. (Xiong et al., 2017a). Pt precursors (H2PtCl6 or Pt (NH3)4Cl2) and deposition methods (photodeposition or chemical reduction) had significant influence on size, distribution, and valence states of Pt nanoparticles, hence led to the difference of photocatatic performance. In particular, the well dispersed Pt nanoparticles fabricated by chemical reducing H2PtCl6 (HC) possessed suitable particle size and high Pt0/PtII ratio (1.15), which led to efficient separation of photogenerated carriers and high efficiency for photoreduction CO2. Coupling with graphene is also an efficient strategy to enhance the activity of anatase TiO2 facet heterojunction ({101}/{001}) for the photoreduction of CO2 (Xiong et al., 2016). Charge transfer between {101} and {001} facets along with the migration of electrons from TiO2 to graphene greatly improved the separation efficiency of photogenerated carriers, thus increasing the yield of CO by photoreducing CO2. Photocatalytic CO2 reduction performance of the typical TiO2-based phase or facet heterojunctions above are listed in Table 3.
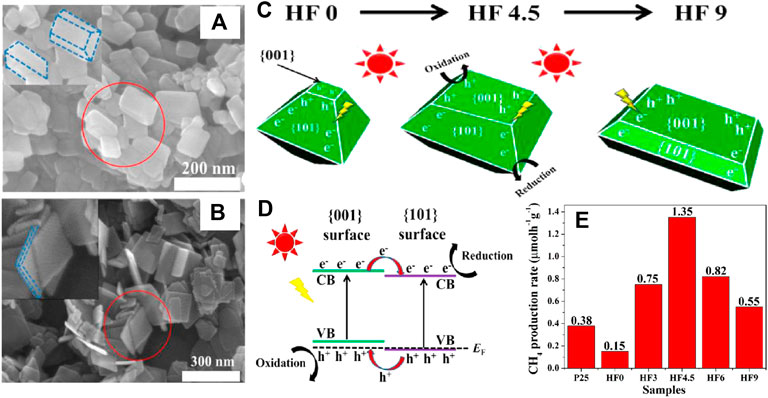
FIGURE 12. FESEM images of (A) HF4.5 and (B) HF9; Schematic illustration of (C) tuning the ratio of {101} to {001} facets of anatase TiO2 by adjusting the amount of HF, and (D) charge transfer at the interface of {101}-{001} facet heterojunction of anatase TiO2; (E) Comparison of the photocatalytic CH4-production activity of P25 and the TiO2 samples prepared by varying HF amount. Reproduced from Yu et al. (2014) with permission from the American Chemical Society.
Conclusions and Prospects
This review summarizes the recent advances in the rational design, fabrication and photocatalytic performance of TiO2-based heterojunctions for converting CO2 into solar fuels with water oxidation. Generally, photocatalytic CO2 reduction that mimic the nature photosynthesis of green plants exhibits great potential for the reduction of CO2 level in the atmosphere and storage of solar energy in hydrocarbon fuels, so as to alleviate the impact of energy crisis and climate change on the development of human society. However, some obstacles, such as low solar energy conversion efficiency, slow generation rate and poor selectivity toward reduction products of CO2, and common photocorrosion phenomenon facing the current photocatalytic systems, restricts the practical application of this very promising technology. In recent years, tremendous efforts have been devoted to fabricate TiO2-based heterojunctions, in order to realize enhanced photocatalytic performance for CO2 conversion, thus giving new life to this traditional and systematically studied photocatalyst. Although the composition, morphology, architecture and photocatalytic mechanism of TiO2-based heterojunctions are various, they have much in common that favors for photoreduction of CO2 as follows: 1) the efficient electron transfer at the heterointerface that promotes spatial separation of photogenerated e−/h+ pairs and prolongs their lifetime to participate in the photoinduced redox reactions; 2) the expanded light adsorption range and enhanced visible light responsibility, making it possible for solar-driven photocatalytic CO2 reduction; 3) the enlarged CO2 adsorption capacity due to the high specific surface area with highly exposed active sites that combine CO2 by chemical action, which could also activate the adsorbed CO2 molecules and facilitate hydrocarbon generation; 4) the increase in selectivity toward specific photoreduction products of CO2 is attributed to the contribution of the cocatalysts.
Although considerable progress has been made on TiO2-based heterojunction for photocatalytic CO2 reduction, it is still far from practical application. On the one hand, the formation of multi-carbon products has always been a bottleneck in this field. The study of CO2 photoreduction intermediates combined with in-situ analysis technology and theoretical calculations needs to be more in-depth in order to clarify the formation mechanisms of different hydrocarbons and guide for the rational design of photocatalysts for the generation of multi-carbon product with high selectivity. On the other hand, pre-defined design of the components and their spatial arrangement in the heterojunction for the optimized phtotcatalytic performance is still a great challenge. It is worth nothing that the difference in synthesis conditions limits the flexibility of component selection, which also complicates the synthesis procedure and reduces the yield of expected heterojunction photocatalyst. At the same time, the randomness arrangement of different components in many cases and the variability of the catalyst morphology and structure affect the photocatalytic performance significantly, which also makes it difficult to clarify the contribution of each component and the synergism mechanism. How to overcome the above limitations to select components of the heterojunction based on photocatalytic performance only, and achieve precise control at the structural unit level, thus realizing efficient synergy of each component for photocatalytic CO2 reduction is one of the main directions of future development in this field. To our knowledge, recent advances in DNA origami superlattice structure (Tian et al., 2016;Tian et al., 2020) may provide a possible solution and guide the design and construction of well-ordered heterojunction photocatalysts in the future. Specifically, the precisely control of the topological structure, arrangement sequence, and assembly quantity of each building block (polyhedral DNA frame) during the self-assembly process makes it possible for heterojunction photocatalysts with controllable structure and adjustable performance, which is expected to become the future research hotspot in the field of photocatalytic CO2 reduction. In addition, efficient solar harvesting systems are in great demand to replace artificial light sources with high energy consumption, since efficient photoreduction of CO2 is based on high light intensity. On the one hand, upconversion quantum dots can be introduced into the heterostructured photocatalyst, in which some of the long-waved visible light in the incident light can be converted into short-waved partial that can excite the photocatalyst to generate e− and h+ pairs. On the other hand, focusing lens system can be added to the photoreactor to enhance the concentration of sunlight, then the photocatalyst can operate under higher light intensity and exhibit optimized activity. Although the efficiency of photocatalytic CO2 reduction is far less than that of electrocatalysis, relying entirely on solar energy will become its irreplaceable advantage. Furthermore, the enrichment of CO2 in air will become an important consideration in the design of heterojunction photocatalysts, which meet the needs of practical applications. Fortunately, the research on CO2 storage and controlled release provides the possible solution while the visible light-triggered capture and release of CO2 from stable MOFs become the most promising candidate (Park et al., 2011; Li et al., 2016; Lyndon et al., 2015). Obviously, combination of photocatalyst and the above MOFs can realize the efficient recycling of CO2 and improve the efficiency of its conversion into solar fuel, which is expected to become a research hot spot in the future.
In summary, the heterojunction photocatalysts with well-organized structure, optimized solar energy conversion efficiency, ideal turnover frequency of CO2, and high reduction product selectivity are still the direction of efforts in the future. We hope that this review can inspire new ideas to guide the design and synthesis of high-performance photocatalysts for photoreduction of CO2 into solar fuels, thus accelerating the industrialization process of this very promising technology and providing practical help to alleviate energy and environmental crises.
Author Contributions
KL designed and wrote the review with input from CT, YT, SW, and QM for conceiving, writing, and editing the manuscript.
Funding
This study was supported by Shenzhen Science and Technology Innovation Program (Grand No. GJHZ20190819151807167), Post-doctoral Foundation Project of Shenzhen Polytechnic (Grand No. 6020330008K0), the Fundamental Research Funds for the Central Universities (Grand No. 020514380141), and China Postdoctoral Science Foundation (Grand No. 2020M671437).
Conflict of Interest
The authors declare that the research was conducted in the absence of any commercial or financial relationships that could be construed as a potential conflict of interest.
References
Aguirre, M. E., Zhou, R., Eugene, A. J., Guzman, M. I., and Grela, M. A. (2017). Cu2O/TiO2 heterostructures for CO2 reduction through a direct Z-scheme: protecting Cu2O from photocorrosion. Appl. Catal. B 217, 485–493. doi:10.1016/j.apcatb.2017.05.058
An, X., Li, K., and Tang, J. (2014). Cu2O/reduced graphene oxide composites for the photocatalytic conversion of CO2. ChemSusChem 7 (4), 1086–1093. doi:10.1002/cssc.201301194
Aresta, M., Dibenedetto, A., and Angelini, A. (2014). Catalysis for the valorization of exhaust carbon: from CO2 to chemicals, materials, and fuels. Technological use of CO2. Chem. Rev. 114 (3), 1709–1742. doi:10.1021/cr4002758
Bai, S., Jiang, J., Zhang, Q., and Xiong, Y. (2015). Steering charge kinetics in photocatalysis: intersection of materials syntheses, characterization techniques and theoretical simulations. Chem. Soc. Rev. 44, 2893–2939. doi:10.1039/c5cs00064e
Barber, J. (2009). Photosynthetic energy conversion: natural and artificial. Chem. Soc. Rev. 38 (1), 185–196. doi:10.1039/b802262n
Bie, C., Zhu, B., Xu, F., Zhang, L., and Yu, J. (2019). In situ grown monolayer N‐doped graphene on CdS hollow spheres with seamless contact for photocatalytic CO2 reduction. Adv. Mater. 31 (42), 1902868. doi:10.1002/adma.201902868
Biswas, M. R. U. D., Ali, A., Cho, K. Y., and amd Oh, W. C. (2018). Novel synthesis of WSe2 -graphene-TiO2 ternary nanocomposite via ultrasonic technics for high photocatalytic reduction of CO2 into CH3OH. Ultrason. Sonochem. 42, 738–746. doi:10.1016/j.ultsonch.2017.12.030
Brockway, P. E., Owen, A., Brand-Correa, L. I., and Hardt, L. (2019). Estimation of global final-stage energy-return-on-investment for fossil fuels with comparison to renewable energy sources. Nat. Energ. 4 (7), 612–621. doi:10.1038/s41560-019-0425-z
Cao, S., Shen, B., Tong, T., Fu, J., and Yu, J. (2018). 2D/2D heterojunction of ultrathin MXene/Bi2WO6 nanosheets for improved photocatalytic CO2 reduction. Adv. Funct. Mater. 28 (21), 1800136. doi:10.1002/adfm.201800136
Cao, Y., Li, Q., Li, C., Li, J., and Yang, J. (2016). Surface heterojunction between (001) and (101) facets of ultrafine anatase TiO2 nanocrystals for highly efficient photoreduction CO2 to CH4. Appl. Catal. B 198, 378–388. doi:10.1016/j.apcatb.2016.05.071
Chen, S., Gao, H., Han, M., Chen, X., Zhang, X., Dong, W., et al. (2020). In situ self-transformation synthesis of N-doped carbon coating paragenetic anatase/rutile heterostructure with enhanced photocatalytic CO2 reduction activity. ChemCatChem 12, 3274–3284. doi:10.1002/cctc.202000137
Chen, X., Zhou, Y., Liu, Q., Li, Z., Liu, J., and Zou, Z. (2012). Ultrathin, single-crystal WO3 nanosheets by two-dimensional oriented attachment toward enhanced photocatalystic reduction of CO2 into hydrocarbon fuels under visible light. ACS Appl. Mater. Inter. 4 (7), 3372–3377. doi:10.1021/am300661s
Cheng, X., Dong, P., Huang, Z., Zhang, Y., Chen, Y., Nie, X., et al. (2017). Green synthesis of plasmonic Ag nanoparticles anchored TiO2 nanorod arrays using cold plasma for visible-light-driven photocatalytic reduction of CO2. J. Co2Util. 20, 200–207. doi:10.1016/j.jcou.2017.04.009
Chong, R., Su, C., Du, Y., Fan, Y., Ling, Z., Chang, Z., et al. (2018). Insights into the role of MgAl layered double oxides interlayer in Pt/TiO2 toward photocatalytic CO2 reduction. J. Catal. 363, 92–101. doi:10.1016/j.jcat.2018.04.020
Chowdhury, S., Parshetti, G. K., and Balasubramanian, R. (2015). Post-combustion CO2 capture using mesoporous TiO2/graphene oxide nanocomposites. Chem. Eng. J. 263, 374–384. doi:10.1016/j.cej.2014.11.037
Corma, A., and Garcia, H. (2013). Photocatalytic reduction of CO2 for fuel production: possibilities and challenges. J. Catal. 308, 168–175. doi:10.1016/j.jcat.2013.06.008
Di Liberto, G., Tosoni, S., and Pacchioni, G. (2019). Role of heterojunction in charge carriers separation in coexposed anatase (001)-(101) surfaces. J. Phys. Chem. Lett. 10, 2372–2377. doi:10.1021/acs.jpclett.9b00504
Dogutan, D. K., and Nocera, D. G. (2019). Artificial photosynthesis at efficiencies greatly exceeding that of natural photosynthesis. Acc. Chem. Res. 52 (11), 3143–3148. doi:10.1021/acs.accounts.9b00380
Fu, J., Jiang, K., Qiu, X., Yu, J., and Liu, M. (2019). Product selectivity of photocatalytic CO2 reduction reactions. Mater. Today 32, 222–243. doi:10.1016/j.mattod.2019.06.009
Fujishima, A., and Honda, K. (1972). Electrochemical photolysis of water at a semiconductor electrode. Nature 238 (5358), 37–38. doi:10.1038/238037a0
Ganesh, I. (2014). Conversion of carbon dioxide into methanol-a potential liquid fuel: fundamental challenges and opportunities (a review). Renew. Sustain. Energ. Rev 31, 221–257. doi:10.1016/j.rser.2013.11.045
Gui, M. M., Chai, S. P., Xu, B. Q., and Mohamed, A. R. (2014). Enhanced visible light responsive MWCNT/TiO2 core–shell nanocomposites as the potential photocatalyst for reduction of CO2 into methane. Sol. Energ. Mater. Sol. Cell 122, 183–189. doi:10.1016/j.solmat.2013.11.034
Gui, M. M., Wong, W. M. P., Chai, S. P., and Mohamed, A. R. (2015). One-pot synthesis of Ag-MWCNT@TiO2 core-shell nanocomposites for photocatalytic reduction of CO2 with water under visible light irradiation. Chem. Eng. J. 278, 272–278. doi:10.1016/j.cej.2014.09.022
Habisreutinger, S. N., Schmidt-Mende, L., and Stolarczyk, J. K. (2013). Photocatalytic reduction of CO2 on TiO2 and other semiconductors. Angew. Chem. Int. Ed. 52 (29), 7372–7408. doi:10.1002/anie.201207199
He, Y., Zhang, L., Fan, M., Wang, X., Walbridge, M. L., Nong, Q., et al. (2015). Z-scheme SnO2−x/g-C3N4 composite as an efficient photocatalyst for dye degradation and photocatalytic CO2 reduction. Sol. Energ. Mater. Sol. Cell 137, 175–184. doi:10.1016/j.solmat.2015.01.037
Höök, M., and Tang, X. (2013). Depletion of fossil fuels and anthropogenic climate change-A review. Energy Policy 52, 797–809. doi:10.1016/j.enpol.2012.10.046
Hwang, H. M., Oh, S., Shim, J. H., Kim, Y. M., Kim, A., Kim, D., et al. (2019). Phase-selective disordered anatase/ordered rutile interface system for visible-light-driven, metal-free CO2 reduction. ACS Appl. Mater. Inter. 11, 35693–35701. doi:10.1021/acsami.9b10837
Inoue, T., Fujishima, A., Konishi, S., and Honda, K. (1979). Photoelectrocatalytic reduction of carbon dioxide in aqueous suspensions of semiconductor powders. Nature 277 (5698), 637–638. doi:10.1038/277637a0
Iqbal, F., Mumtaz, A., Shahabuddin, S., Abd Mutalib, M. I., Shaharun, M. S., Nguyen, T. D., et al. (2020). Photocatalytic reduction of CO2 to methanol over ZnFe2O4/TiO2 (p‐n) heterojunctions under visible light irradiation. J. Chem. Technol. Biot. 95 (8), 2208–2221. doi:10.1002/jctb.6408
Jiao, J., Wei, Y., Zhao, Z., Zhong, W., Liu, J., Li, J., et al. (2015). Synthesis of 3D ordered macroporous TiO2-supported Au nanoparticle photocatalysts and their photocatalytic performances for the reduction of CO2 to methane. Catal. Today 258, 319–326. doi:10.1016/j.cattod.2015.01.030
Jin, J., Chen, S., Wang, J., Chen, C., and Peng, T. (2019). SrCO3-modified brookite/anatase TiO2 heterophase junctions with enhanced activity and selectivity of CO2 photoreduction to CH4. Appl. Surf. Sci. 476, 937–947. doi:10.1016/j.apsusc.2019.01.176
Jung, H., Cho, K. M., Kim, K. H., Yoo, H. W., Al-Saggaf, A., Gereige, I., et al. (2018). Highly efficient and stable CO2 reduction photocatalyst with a hierarchical structure of mesoporous TiO2 on 3D graphene with few-layered MoS2. ACS Sustain. Chem. Eng. 6 (5), 5718–5724. doi:10.1021/acssuschemeng.8b00002
Kegel, J., Povey, I. M., and Pemble, M. E. (2018). Zinc oxide for solar water splitting: a brief review of the material’s challenges and associated opportunities. Nano Energy 54, 409–428. doi:10.1016/j.nanoen.2018.10.043
Khatun, F., Abd Aziz, A., Sim, L. C., and Monir, M. U. (2019). Plasmonic enhanced Au decorated TiO2 nanotube arrays as a visible light active catalyst towards photocatalytic CO2 conversion to CH4. J. Environ. Chem. Eng. 7, 103233. doi:10.1016/j.jece.2019.103233
Kim, K. H., Kim, S., Moon, B. C., Choi, J. W., Jeong, H. M., Kwon, Y., et al. (2017). Quadruple metal-based layered structure as the photocatalyst for conversion of carbon dioxide into a value added carbon monoxide with high selectivity and efficiency. J. Mater. Chem. A. 5 (18), 8274–8279. doi:10.1039/c7ta01623a
Kondratenko, E. V., Mul, G., Baltrusaitis, J., Larrazabal, G. O., and Perez-Ramirez, J. (2013). Status and perspectives of CO2 conversion into fuels and chemicals by catalytic, photocatalytic and electrocatalytic processes. Energy Environ. Sci. 6 (11), 3112–3135. doi:10.1039/c3ee41272e
Kuai, L., Zhou, Y., Tu, W., Li, P., Li, H., Xu, Q., et al. (2015). Rational construction of a CdS/reduced graphene oxide/TiO2 core-shell nanostructure as an all-solid-state Z-scheme system for CO2 photoreduction into solar fuels. RSC Adv. 5 (107), 88409–88413. doi:10.1039/c5ra14374h
Kuehnel, M. F., Orchard, K. L., Dalle, K. E., and Reisner, E. (2017). Selective photocatalytic CO2 reduction in water through anchoring of a molecular Ni catalyst on CdS nanocrystals. J. Am. Chem. Soc. 139 (21), 7217–7223. doi:10.1021/jacs.7b00369
Lee, J. D., Sorescu, C., and Deng, X. Y. (2011). Electron-induced dissociation of CO2 on TiO2(110). J. Am. Chem. Soc. 133 (26), 10066–10069. doi:10.1021/ja204077e
Lee, J. S., Won, D. I., Jung, W. J., Son, H. J., Pac, C., and Kang, S. O. (2016). Widely controllable syngas production by a dye-sensitized TiO2 hybrid system with ReI and CoIII catalysts under visible-light irradiation. Angew. Chem. Int. Ed. 56 (4), 976–980. doi:10.1002/anie.201608593
Lee, K. M., Lai, C. W., Ngai, K. S., and Juan, J. C. (2016). Recent developments of zinc oxide based photocatalyst in water treatment technology: a review. Water Res. 88, 428–448. doi:10.1016/j.watres.2015.09.045
Lee, K. Y., Sato, K., and Mohamed, A. R. (2016). Facile synthesis of anatase-rutile TiO2 composites with enhanced CO2 photoreduction activity and the effect of Pt loading on product selectivity. Mater. Lett. 163, 240–243. doi:10.1016/j.matlet.2015.10.094
Lee, J. H., Kim, S. I., Park, S. M., and Kang, M. (2017). A p-n heterojunction NiS-sensitized TiO2 photocatalytic system for efficient photoreduction of carbon dioxide to methane. Ceram. Int. 43 (2), 1768–1774. doi:10.1016/j.ceramint.2016.10.122
Li, H., Sadiq, M. M., Suzuki, K., Ricco, R., Doblin, C., Hill, A. J., et al. (2016). Magnetic metal-organic frameworks for efficient carbon dioxide capture and remote trigger release. Adv. Mater. 28 (9), 1839–1844. doi:10.1002/adma.201505320
Li, M., Wang, M., Zhu, L., Li, Y., Yan, Z., Shen, Z., et al. (2018). Facile microwave assisted synthesis of N-rich carbon quantum dots/dual-phase TiO2 heterostructured nanocomposites with high activity in CO2 photoreduction. Appl. Catal. B 231, 269–276. doi:10.1016/j.apcatb.2018.03.027
Li, X., Yu, J., Jaroniec, M., and Chen, X. (2019). Cocatalysts for selective photoreduction of CO2 into solar fuels. Chem. Rev. 119, 3962–4179. doi:10.1021/acs.chemrev.8b00400
Lin, L. Y., Nie, Y., Kavadiya, S., Soundappan, T., and Biswas, P. (2017). N-doped reduced graphene oxide promoted nano TiO2 as a bifunctional adsorbent/photocatalyst for CO2 photoreduction: effect of N species. Chem. Eng. J. 316, 449–460. doi:10.1016/j.cej.2017.01.125
Liu, L., Jiang, Y., Zhao, H., Chen, J., Cheng, J., Yang, K., et al. (2016). Engineering coexposed {001} and {101} facets in oxygen-deficient TiO2 nanocrystals for enhanced CO2 photoreduction under visible light. ACS Catal. 6 (2), 1097–1108. doi:10.1021/acscatal.5b02098
Liu, X., Inagaki, S., and Gong, J. (2016). Heterogeneous molecular systems for photocatalytic CO2 reduction with water oxidation. Angew. Chem. Int. Ed. 55 (48), 14924–14950. doi:10.1002/anie.201600395
Liu, Y., Shen, D., Zhang, Q., Lin, Y., and Peng, F. (2021). Enhanced photocatalytic CO2 reduction in H2O vapor by atomically thin Bi2WO6 nanosheets with hydrophobic and nonpolar surface. Appl. Catal. B 283, 119630. doi:10.1016/j.apcatb.2020.119630
Low, J., Qiu, S., Xu, D., Jiang, C., and Cheng, B. (2018). Direct evidence and enhancement of surface plasmon resonance effect on Ag-loaded TiO2 nanotube arrays for photocatalytic CO2 reduction. Appl. Surf. Sci. 434, 423–432. doi:10.1016/j.apsusc.2017.10.194
Low, J., Dai, B., Tong, T., Jiang, C., and Yu, J. (2019). In situ irradiated X-ray photoelectron spectroscopy investigation on a direct Z-scheme TiO2/CdS composite film photocatalyst. Adv. Mater. 31, 1802981. doi:10.1002/adma.201802981
Lyndon, R., Konstas, K., Thornton, A. W., Seeber, A. J., Ladewig, B. P., and Hill, M. R. (2015). Visible light-triggered capture and release of CO2 from stable metal organic frameworks. Chem. Mater. 27 (23), 7882–7888. doi:10.1021/acs.chemmater.5b02211
Ma, Y., Wang, X., Jia, Y., Chen, X., Han, H., and Li, C. (2014). Titanium dioxide-based nanomaterials for photocatalytic fuel generations. Chem. Rev. 114 (19), 9987–10043. doi:10.1021/cr500008u
Mao, J., Peng, T., Zhang, X., Li, K., and Zan, L. (2012). Selective methanol production from photocatalytic reduction of CO2 on BiVO4 under visible light irradiation. Catal. Commun. 28, 38–41. doi:10.1016/j.catcom.2012.08.008
Meinshausen, M., Meinshausen, N., Hare, W., Raper, S. C. B., Frieler, K., Knutti, R., et al. (2009). Greenhouse-gas emission targets for limiting global warming to 2 °C. Nature 458 (7242), 1158–1162. doi:10.1038/nature08017
Meng, A., Zhang, L., Cheng, B., and Yu, J. (2019). TiO2-MnOx-Pt hybrid multiheterojunction film photocatalyst with enhanced photocatalytic CO2-reduction activity. ACS Appl. Mater. Inter. 11, 5581–5589. doi:10.1021/acsami.8b02552
Neaţu, Ş., Maciá-Agulló, J. A., Concepción, P., and Garcia, H. (2014). Gold-copper nanoalloys supported on TiO2 as photocatalysts for CO2 reduction by water. J. Am. Chem. Soc. 136 (45), 15969–15976. doi:10.1021/ja506433k
Nguyen, T. P., Nguyen, D. L. T., Nguyen, V. H., Le, T. H., Vo, D. V. N., Trinh, Q. T., et al. (2020). Recent advances in TiO2-based photocatalysts for reduction of CO2 to fuels. Nanomaterials 10 (2), 337. doi:10.3390/nano10020337
Nie, N., Zhang, L., Fu, J., Cheng, B., and Yu, J. (2018). Self-assembled hierarchical direct Z-scheme g-C3N4/ZnO microspheres with enhanced photocatalytic CO2 reduction performance. Appl. Surf. Sci. 441, 12–22. doi:10.1016/j.apsusc.2018.01.193
Ola, O., and Maroto-Valer, M. M. (2015). Review of material design and reactor engineering on TiO2 photocatalysis for CO2 reduction. J. Photochem. Photobiol. C 24, 16–42. doi:10.1016/j.jphotochemrev.2015.06.001
Olah, G. A., Prakash, G. K. S., and Goeppert, A. (2011). Anthropogenic chemical carbon cycle for a sustainable future. J. Am. Chem. Soc. 133 (33), 12881–12898. doi:10.1021/ja202642y
Olowoyo, J. O., Kumar, M., Jain, S. L., Babalola, J. O., Vorontsov, A. V., and Kumar, U. (2018). Insight into reinforced photocatalytic activity of CNT-TiO2 nanocomposite for CO2 reduction and water splitting. J. Phys. Chem. C 123, 367–378. doi:10.1021/acs.jpcc.8b07894
Olowoyo, J. O., Kumar, M., Singh, B., Oninla, V. O., Babalola, J. O., Valdés, H., et al. (2019). Self-assembled reduced graphene oxide-TiO2 nanocomposites: synthesis, DFTB+ calculations, and enhanced photocatalytic reduction of CO2 to methanol. Carbon 147, 385–397. doi:10.1016/j.carbon.2019.03.019
Park, J., Yuan, D., Pham, K. T., Li, J. R., Yakovenko, A., and Zhou, H. C. (2011). Reversible alteration of CO2 adsorption upon photochemical or thermal treatment in a metal-organic framework. J. Am. Chem. Soc. 134 (1), 99–102. doi:10.1021/ja209197f
Patil, S. B., Basavarajappa, P. S., Ganganagappa, N., Jyothi, M. S., Raghu, A. V., and Reddy, K. R. (2019). Recent advances in non-metals-doped TiO2 nanostructured photocatalysts for visible-light driven hydrogen production, CO2 reduction and air purification. Inter. J. Hydrogen Energ. doi:10.1016/j.ijhydene.2019.03.164
Raza, A., Shen, H., Haidry, A. A., Sun, L., Liu, R., and Cui, S. (2020). Studies of Z-scheme WO3-TiO2/Cu2ZnSnS4 ternary nanocomposite with enhanced CO2 photoreduction under visible light irradiation. J. Co2Util. 37, 260–271. doi:10.1016/j.jcou.2019.12.020
Reñones, P., Moya, A., Fresno, F., Collado, L., Vilatela, J. J., and de la Peña O’Shea, V. A. (2016). Hierarchical TiO2 nanofibres as photocatalyst for CO2 reduction: influence of morphology and phase composition on catalytic activity. J. Co2Util. 15, 24–31. doi:10.1016/j.jcou.2016.04.002
Rodríguez, V., Camarillo, R., Martínez, F., Jiménez, C., and Rincón, J. (2020). CO2 photocatalytic reduction with CNT/TiO2 based nanocomposites prepared by high-pressure technology. J. Supercrit. Fluids 163, 104876. doi:10.1016/j.supflu.2020.104876
She, H., Wang, Y., Zhou, H., Li, Y., Wang, L., Huang, J., et al. (2018). Preparation of Zn3In2S6/TiO2 for enhanced CO2 photocatalytic reduction activity via Z-scheme electron transfer. ChemCatChem 11, 753–759. doi:10.1002/cctc.201801745
Shehzad, N., Tahir, M., Johari, K., Murugesan, T., and Hussain, M. (2018a). Improved interfacial bonding of graphene-TiO2 with enhanced photocatalytic reduction of CO2 into solar fuel. J. Environ. Chem. Eng. 6, 6947–6957. doi:10.1016/j.jece.2018.10.065
Shehzad, N., Tahir, M., Johari, K., Murugesan, T., and Hussain, M. (2018b). A critical review on TiO2 based photocatalytic CO2 reduction system: strategies to improve efficiency. J. Co2 Util. 26, 98–122. doi:10.1016/j.jcou.2018.04.026
Shi, W., Guo, X., Cui, C., Jiang, K., Li, Z., Qu, L., et al. (2019). Controllable synthesis of Cu2O decorated WO3 nanosheets with dominant (001) facets for photocatalytic CO2 reduction under visible-light irradiation. Appl. Catal. B 243, 236–242. doi:10.1016/j.apcatb.2018.09.076
Shindell, D., and Smith, C. J. (2019). Climate and air-quality benefits of a realistic phase-out of fossil fuels. Nature 573 (7774), 408–411. doi:10.1038/s41586-019-1554-z
Sim, L. C., Leong, K. H., Saravanan, P., and Ibrahim, S. (2015). Rapid thermal reduced graphene oxide/Pt-TiO2 nanotube arrays for enhanced visible-light-driven photocatalytic reduction of CO2. Appl. Surf. Sci. 358, 122–129. doi:10.1016/j.apsusc.2015.08.065
Solomon, S., Plattner, G. K., Knutti, R., and Friedlingstein, P. (2009). Irreversible climate change due to carbon dioxide emissions. Proc. Natl. Acad. Sci. U. S. A. 106, 1704–1709. doi:10.1073/pnas.0812721106
Stott, P. A., Tett, S., Jones, G., Allen, M., Mitchell, J., and Jenkins, G. (2000). External control of 20th century temperature by natural and anthropogenic forcings. Science 290 (5499), 2133–2137. doi:10.1126/science.290.5499.2133
Tahir, M., Tahir, B., and Amin, N. A. S. (2017). Synergistic effect in plasmonic Au/Ag alloy NPs co-coated TiO2 NWs toward visible-light enhanced CO2 photoreduction to fuels. Appl. Catal. B 204, 548–560. doi:10.1016/j.apcatb.2016.11.062
Tahir, M. (2020). Well-designed ZnFe2O4/Ag/TiO2 nanorods heterojunction with Ag as electron mediator for photocatalytic CO2 reduction to fuels under UV/visible light. J. Co2Util. 37, 134–146. doi:10.1016/j.jcou.2019.12.004
Takayama, T., Sato, K., Fujimura, T., Kojima, Y., Iwase, A., and Kudo, A. (2017). Photocatalytic CO2 reduction using water as an electron donor by a powdered Z-scheme system consisting of metal sulfide and an RGO-TiO2 composite. Faraday Discuss. 198, 397–407. doi:10.1039/c6fd00215c
Tan, L. L., Ong, W. J., Chai, S. P., and Mohamed, A. R. (2015). Noble metal modified reduced graphene oxide/TiO2 ternary nanostructures for efficient visible-light-driven photoreduction of carbon dioxide into methane. Appl. Catal. B 166-167, 251–259. doi:10.1016/j.apcatb.2014.11.035
Tan, L. L., Ong, W. J., Chai, S. P., and Mohamed, A. R. (2017). Photocatalytic reduction of CO2 with H2O over graphene oxide-supported oxygen-rich TiO2 hybrid photocatalyst under visible light irradiation: process and kinetic studies. Chem. Eng. J. 308, 248–255. doi:10.1016/j.cej.2016.09.050
Tan, D., Zhang, J., Shi, J., Li, S., Zhang, B., Tan, X., et al. (2018). Photocatalytic CO2 transformation to CH4 by Ag/Pd bimetals supported on N-doped TiO2 nanosheet. ACS Appl. Mater. Inter. 10 (29), 24516–24522. doi:10.1021/acsami.8b06320
Tasbihi, M., Fresno, F., Simon, U., Villar-García, I. J., Pérez-Dieste, V., Escudero, C., et al. (2018a). On the selectivity of CO2 photoreduction towards CH4 using Pt/TiO2 catalysts supported on mesoporous silica. Appl. Catal. B 239, 68–76. doi:10.1016/j.apcatb.2018.08.003
Tasbihi, M., Kočí, K., Edelmannová, M., Troppová, I., Reli, M., and Schomäcker, R. (2018b). Pt/TiO2 photocatalysts deposited on commercial support for photocatalytic reduction of CO2. J. Photochem. Photobiol. A. 366, 72–80. doi:10.1016/j.jphotochem.2018.04.012
Thi Thanh Truc, N., Giang Bach, L., Thi Hanh, N., Pham, T. D., Thi Phuong Le Chi, N., Trinh Tran, D., et al. (2019). The superior photocatalytic activity of Nb doped TiO2/g-C3N4 direct Z-scheme system for efficient conversion of CO2 into valuable fuels. J. Colloid Interf. Sci. 540, 1–8. doi:10.1016/j.jcis.2019.01.005
Tian, Y., Lhermitte, J. R., Bai, L., Vo, T., Xin, H. L., Li, H., et al. (2020). Ordered three-dimensional nanomaterials using DNA-prescribed and valence-controlled material voxels. Nat. Mater. doi:10.1038/s41563-019-0550-x
Tian, Y., Zhang, Y., Wang, T., Xin, H. L., Li, H., and Gang, O. (2016). Lattice engineering through nanoparticle-DNA frameworks. Nat. Mater. 15, 654–661. doi:10.1038/NMAT4571
Tu, W., Zhou, Y., Liu, Q., Yan, S., Bao, S., Wang, X., et al. (2013). An in situ simultaneous reduction-hydrolysis technique for fabrication of TiO2-graphene 2D sandwich-like hybrid nanosheets: graphene-promoted selectivity of photocatalytic-driven hydrogenation and coupling of CO2 into methane and ethane. Adv. Funct. Mater. 23 (14), 1743–1749. doi:10.1002/adfm.201202349
Tu, W., Zhou, Y., and Zou, Z. (2014). Photocatalytic conversion of CO2 into renewable hydrocarbon fuels: state-of-the-art accomplishment, challenges, and prospects. Adv. Mater. 26 (27), 4607–4626. doi:10.1002/adma.201400087
Tu, W., Guo, W., Hu, J., He, H., Li, H., Li, Z., et al. (2020). State-of-the-art advancements of crystal facet-exposed photocatalysts beyond TiO2: design and dependent performance for solar energy conversion and environment applications. Mater. Today 33, 75–86. doi:10.1016/j.mattod.2019.09.003
Wang, H., Zhang, L., Chen, Z., Hu, J., Li, S., Wang, Z., et al. (2014). Semiconductor heterojunction photocatalysts: design, construction, and photocatalytic performances. Chem. Soc. Rev. 43 (15), 5234. doi:10.1039/c4cs00126e
Wang, W., Xu, D., Cheng, B., Yu, J., and Jiang, C. (2017). Hybrid carbon@TiO2 hollow spheres with enhanced photocatalytic CO2 reduction activity. J. Mater. Chem. A. 5 (10), 5020–5029. doi:10.1039/c6ta11121a
Wang, H., Yong, D., Chen, S., Jiang, S., Zhang, X., Shao, W., et al. (2018). Oxygen-vacancy-mediated exciton dissociation in BiOBr for boosting charge-carrier-involved molecular oxygen activation. J. Am. Chem. Soc. 140 (5), 1760–1766. doi:10.1021/jacs.7b10997
Wang, H., Zhang, L., Wang, K., Sun, X., and Wang, W. (2019). Enhanced photocatalytic CO2 reduction to methane over WO3·0.33H2O via Mo doping. Appl. Catal. B 243, 771–779. doi:10.1016/j.apcatb.2018.11.021
Wang, K., Jiang, R., Peng, T., Chen, X., Dai, W., and Fu, X. (2019). Modeling the effect of Cu doped TiO2 with carbon dots on CO2 methanation by H2O in a photo-thermal system. Appl. Catal. B 256, 117780. doi:10.1016/j.apcatb.2019.117780
Wang, L., Jin, P., Huang, J., She, H., and Wang, Q. (2019). Integration of copper(II)-porphyrin zirconium metal-organic framework and titanium dioxide to construct Z-scheme system for highly improved photocatalytic CO2 reduction. ACS Sustain. Chem. Eng. 7, 15660–15670. doi:10.1021/acssuschemeng.9b03773
Wang, R., Shen, J., Sun, K., Tang, H., and Liu, Q. (2019). Enhancement in photocatalytic activity of CO2 reduction to CH4 by 0D/2D Au/TiO2 plasmon heterojunction. Appl. Surf. Sci. 493, 1142–1149. doi:10.1016/j.apsusc.2019.07.121
Wang, C., Liu, X., He, W., Zhao, Y., Wei, Y., Xiong, J., et al. (2020a). All-solid-state Z-scheme photocatalysts of g-C3N4/Pt/macroporous-(TiO2@carbon) for selective boosting visible-light-driven conversion of CO2 to CH4. J. Catal. 389, 440–449. doi:10.1016/j.jcat.2020.06.026
Wang, C., Zhao, Y., Xu, H., Li, Y., Wei, Y., Liu, J., et al. (2020b). Efficient Z-scheme photocatalysts of ultrathin g-C3N4-wrapped Au/TiO2-nanocrystals for enhanced visible-light-driven conversion of CO2 with H2O. Appl. Catal. B 263, 118314. doi:10.1016/j.apcatb.2019.118314
Wang, A., Wu, S., Dong, J., Wang, R., Wang, J., Zhang, J., et al. (2021). Interfacial facet engineering on the Schottky barrier between plasmonic Au and TiO2 in boosting the photocatalytic CO2 reduction under ultraviolet and visible light irradiation. Chem. Eng. J. 404, 127145. doi:10.1016/j.cej.2020.127145
Wei, L., Yu, C., Zhang, Q., Liu, H., and Wang, Y. (2018). TiO2-based heterojunction photocatalysts for photocatalytic reduction of CO2 to solar fuels. J. Mater. Chem. A. 6, 22411–22436. doi:10.1039/c8ta08879a
Wei, Y., Wu, X., Zhao, Y., Wang, L., Zhao, Z., Huang, X., et al. (2018). Efficient photocatalysts of TiO2 nanocrystals-supported PtRu alloy nanoparticles for CO2 reduction with H2O: synergistic effect of Pt-Ru. Appl. Catal. B 236, 445–457. doi:10.1016/j.apcatb.2018.05.043
Wei, Z. H., Wang, Y. F., Li, Y. Y., Zhang, L., Yao, H. C., and Li, Z. J. (2018). Enhanced photocatalytic CO2 reduction activity of Z-scheme CdS/BiVO4 nanocomposite with thinner BiVO4 nanosheets. J. Co2 Util. 28, 15–25. doi:10.1016/j.jcou.2018.09.008
White, J. L., Baruch, M. F., Pander, J. E., Hu, Y., Fortmeyer, I. C., Park, J. E., et al. (2015). Light-driven heterogeneous reduction of carbon dioxide: photocatalysts and photoelectrodes. Chem. Rev. 115 (23), 12888–12935. doi:10.1021/acs.chemrev.5b00370
Woo, S. J., Choi, S., Kim, S. Y., Kim, P. S., Jo, J. H., Kim, C. H., et al. (2019). Highly selective and durable photochemical CO2 reduction by molecular Mn(I) catalyst fixed on particular dye-sensitized TiO2 platform. ACS Catal. 9 (3), 2580–2593. doi:10.1021/acscatal.8b03816
Wu, J., Li, X., Shi, W., Ling, P., Sun, Y., Jiao, X., et al. (2018). Efficient visible-light-driven CO2 reduction mediated by defect-engineered BiOBr atomic layers. Angew. Chem. Int. Ed. 130 (28), 8855–8859. doi:10.1002/ange.201803514
Wu, J., Feng, Y., Logan, B. E., Dai, C., Han, X., Li, D., et al. (2019). Preparation of Al−O-Linked porous-g-C3N4/TiO2-nanotube Z-scheme composites for efficient photocatalytic CO2 conversion and 2,4-dichlorophenol decomposition and mechanism. ACS Sustain. Chem. Eng. 7, 15289–15296. doi:10.1021/acssuschemeng.9b02489
Xia, X. H., Jia, Z. J., Yu, Y., Liang, Y., Wang, Z., and Ma, L. L. (2007). Preparation of multi-walled carbon nanotube supported TiO2 and its photocatalytic activity in the reduction of CO2 with H2O. Carbon 45 (4), 717–721. doi:10.1016/j.carbon.2006.11.028
Xie, S., Wang, Y., Zhang, Q., Fan, W., Deng, W., and Wang, Y. (2013). Photocatalytic reduction of CO2 with H2O: significant enhancement of the activity of Pt-TiO2 in CH4 formation by addition of MgO. Chem. Commun. 49 (24), 2451–2453. doi:10.1039/c3cc00107e
Xie, S., Wang, Y., Zhang, Q., Deng, W., and Wang, Y. (2014). MgO-and Pt-promoted TiO2 as an efficient photocatalyst for the preferential reduction of carbon dioxide in the presence of water. ACS Catal. 4, 3644–3653. doi:10.1021/cs500648p
Xie, S., Zhang, Q., Liu, G., and Wang, Y. (2016). Photocatalytic and photoelectrocatalytic reduction of CO2 using heterogeneous catalysts with controlled nanostructures. Chem. Commun. 52 (1), 35–59. doi:10.1039/c5cc07613g
Xiong, Z., Wang, H., Xu, N., Li, H., Fang, B., Zhao, Y., et al. (2015). Photocatalytic reduction of CO2 on Pt2+-Pt0/TiO2 nanoparticles under UV/Vis light irradiation: a combination of Pt2+ doping and Pt nanoparticles deposition. Inter. J. Hydrogen Energ. 40 (32), 10049–10062. doi:10.1016/j.ijhydene.2015.06.075
Xiong, Z., Luo, Y., Zhao, Y., Zhang, J., Zheng, C., and Wu, J. C. S. (2016). Synthesis, characterization and enhanced photocatalytic CO2 reduction activity of graphene supported TiO2 nanocrystals with coexposed {001} and {101} facets. Phys. Chem. Chem. Phys. 18 (19), 13186–13195. doi:10.1039/c5cp07854g
Xiong, Z., Lei, Z., Chen, X., Gong, B., Zhao, Y., Zhang, J., et al. (2017a). CO2 photocatalytic reduction over Pt deposited TiO2 nanocrystals with coexposed {101} and {001} facets: effect of deposition method and Pt precursors. Catal. Commu. 96, 1–5. doi:10.1016/j.catcom.2017.03.013
Xiong, Z., Lei, Z., Gong, B., Chen, X., Zhao, Y., Zhang, J., et al. (2017b). A novel reaction mode using H2 produced from solid-liquid reaction to promote CO2 reduction through solid-gas reaction. Catal. Commun. 89, 4–8. doi:10.1016/j.catcom.2016.10.010
Xiong, Z., Lei, Z., Kuang, C. C., Chen, X., Gong, B., Zhao, Y., et al. (2017c). Selective photocatalytic reduction of CO2 into CH4 over Pt-Cu2O TiO2 nanocrystals: the interaction between Pt and Cu2O cocatalysts. Appl. Catal. B 202, 695–703. doi:10.1016/j.apcatb.2016.10.001
Xiong, Z., Lei, Z., Li, Y., Dong, L., Zhao, Y., and Zhang, J. (2018). A review on modification of facet-engineered TiO2 for photocatalytic CO2 reduction. J. Photochem. Photobiol. C 36, 24–47. doi:10.1016/j.jphotochemrev.2018.07.002
Xiong, J., Zhang, M., and Cheng, G. (2020). Facile polyol-triggered anatase-rutile heterophase TiO2-x nanoparticles for enhancing photocatalytic CO2 reduction. J. Colloid Interf. Sci. 579, 872–877. doi:10.1016/j.jcis.2020.06.103
Xu, Q., Yu, J., Zhang, J., Zhang, J., and Liu, G. (2015). Cubic anatase TiO2 nanocrystals with enhanced photocatalytic CO2 reduction activity. Chem. Commun. 51 (37), 7950–7953. doi:10.1039/c5cc01087j
Xu, F., Meng, K., Cheng, B., Yu, J., and Ho, W. (2018a). Enhanced photocatalytic activity and selectivity for CO2 reduction over a TiO2 nanofibre mat using Ag and MgO as bi-cocatalyst. ChemCatChem 11, 465–472. doi:10.1002/cctc.201801282
Xu, F., Zhang, J., Zhu, B., Yu, J., and Xu, J. (2018b). CuInS2 sensitized TiO2 hybrid nanofibers for improved photocatalytic CO2 reduction. Appl. Catal. B 230, 194–202. doi:10.1016/j.apcatb.2018.02.042
Xu, S., and Carter, E. A. (2019). Theoretical insights into heterogeneous (photo)electrochemical CO2 reduction. Chem. Rev. 119, 6631–6669. doi:10.1021/acs.chemrev.8b00481
Yang, G., Chen, D., Ding, H., Feng, J., Zhang, J. Z., Zhu, Y., et al. (2017). Well-designed 3D ZnIn2S4 nanosheets/TiO2 nanobelts as direct Z-scheme photocatalysts for CO2 photoreduction into renewable hydrocarbon fuel with high efficiency. Appl. Catal. B 219, 611–618. doi:10.1016/j.apcatb.2017.08.016
Ye, L., Jin, X., Liu, C., Ding, C., Xie, H., Chu, K. H., et al. (2016). Thickness-ultrathin and bismuth-rich strategies for BiOBr to enhance photoreduction of CO2 into solar fuels. Appl. Catal. B 187, 281–290. doi:10.1016/j.apcatb.2016.01.044
You, F., Wan, J., Qi, J., Mao, D., Yang, N., Zhang, Q., et al. (2020). Lattice distortion in hollow multi‐shelled structures for efficient visible light CO2 reduction with SnS2/SnO2 junction. Angew. Chem. Int. Ed. 132 (2), 731–734. doi:10.1002/ange.201912069
Yu, J., Low, J., Xiao, W., Zhou, P., and Jaroniec, M. (2014). Enhanced photocatalytic CO2-reduction activity of anatase TiO2 by coexposed {001} and {101} facets. J. Am. Chem. Soc. 136 (25), 8839–8842. doi:10.1021/ja5044787
Yu, B., Zhou, Y., Li, P., Tu, W., Li, P., Tang, L., et al. (2016). Photocatalytic reduction of CO2 over Ag/TiO2 nanocomposites prepared with a simple and rapid silver mirror method. Nanoscale 8 (23), 11870–11874. doi:10.1039/c6nr02547a
Zeng, G., Qiu, J., Li, Z., Pavaskar, P., and Cronin, S. B. (2014). CO2 reduction to methanol on TiO2-passivated GaP photocatalysts. ACS Cataly 4 (10), 3512–3516. doi:10.1021/cs500697w
Zeng, S., Vahidzadeh, E., VanEssen, C. G., Kar, P., Kisslinger, R., Goswami, A., et al. (2020). Optical control of selectivity of high rate CO2 photoreduction via interband- or hot electron Z-scheme reaction pathways in Au-TiO2 plasmonic photonic crystal photocatalyst. Appl. Catal. B 267, 118644. doi:10.1016/j.apcatb.2020.118644
Zhang, B., and Sun, L. (2019). Artificial photosynthesis: opportunities and challenges of molecular catalysts. Chem. Soc. Rev. 48, 2216–2264. doi:10.1039/c8cs00897c
Zhang, J., Fu, J., Chen, S., Lv, J., and Dai, K. (2018). 1D carbon nanofibers@TiO2 core-shell nanocomposites with enhanced photocatalytic activity toward CO2 reduction. J. Alloys Compd. 746, 168–176. doi:10.1016/j.jallcom.2018.02.265
Zhang, L., Cao, H., Pen, Q., Wu, L., Hou, G., Tang, Y., et al. (2018). Embedded CuO nanoparticles@TiO2-nanotube arrays for photoelectrocatalytic reduction of CO2 to methanol. Electrochim. Acta 283, 1507–1513. doi:10.1016/j.electacta.2018.07.072
Zhang, L., and Jaroniec, M. (2018). Toward designing semiconductor-semiconductor heterojunctions for photocatalytic applications. Appl. Surf. Sci. 430, 2–17. doi:10.1016/j.apsusc.2017.07.192
Zhang, L., Cao, H., Lu, Y., Zhang, H., Hou, G., Tang, Y., et al. (2020). Effective combination of CuFeO2 with high temperature resistant Nb-doped TiO2 nanotube arrays for CO2 photoelectric reduction. J. Colloid Interf. Sci. 568, 198–206. doi:10.1016/j.jcis.2020.01.082
Zhao, H., Zheng, X., Feng, X., and Li, Y. (2018). CO2 reduction by plasmonic Au nanoparticle-decorated TiO2 photocatalyst with an ultrathin Al2O3 interlayer. J. Phys. Chem. C 122, 18949–18956. doi:10.1021/acs.jpcc.8b04239
Zhao, Y., Wei, Y., Wu, X., Zheng, H., Zhao, Z., Liu, J., et al. (2018). Graphene-wrapped Pt/TiO2 photocatalysts with enhanced photogenerated charges separation and reactant adsorption for high selective photoreduction of CO2 to CH4. Appl. Catal. B 226, 360–372. doi:10.1016/j.apcatb.2017.12.071
Zhu, S., Liao, W., Zhang, M., and Liang, S. (2019). Design of spatially separated Au and CoO Ziaratidual cocatalysts on hollow TiO2 for enhanced photocatalytic activity towards the reduction of CO2 to CH4. Chem. Eng. J. 361, 461–469. doi:10.1016/j.cej.2018.12.095
Ziarati, A., Badiei, A., Luque, R., Dadras, M., and Burgi, T. (2020). Visible light CO2 reduction to CH4 using hierarchical yolk@shell TiO2-xHx modified with plasmonic Au-Pd nanoparticles. ACS Sustain. Chem. Eng. 8, 3689–3696. doi:10.1021/acssuschemeng.9b06751
Keywords: TIO2-based photocatalysts, heterostructures, CO2 photoreduction, water oxidation, high efficiency
Citation: Li K, Teng C, Wang S and Min Q (2021) Recent Advances in TiO2-Based Heterojunctions for Photocatalytic CO2 Reduction With Water Oxidation: A Review. Front. Chem. 9:637501. doi: 10.3389/fchem.2021.637501
Received: 03 December 2020; Accepted: 01 February 2021;
Published: 15 April 2021.
Edited by:
Ping Yu, Institute of Chemistry, ChinaReviewed by:
Chang-Yong Nam, Brookhaven National Laboratory, United StatesYuqing Lin, Capital Normal University, China
Limin Zhang, East China Normal University, China
Copyright © 2021 Li, Teng, Wang and Min. This is an open-access article distributed under the terms of the Creative Commons Attribution License (CC BY). The use, distribution or reproduction in other forums is permitted, provided the original author(s) and the copyright owner(s) are credited and that the original publication in this journal is cited, in accordance with accepted academic practice. No use, distribution or reproduction is permitted which does not comply with these terms.
*Correspondence: Chao Teng, dGVuZ2NoYW9Ac3pwdC5lZHUuY24=; Qianhao Min, bWlucWlhbmhhb0BuanUuZWR1LmNu