- 1State Key Laboratory for Chemistry and Molecular Engineering of Medicinal Resources, School of Chemistry and Pharmaceutical Science, Guangxi Normal University, Guilin, China
- 2Guilin Normal College, Guilin, China
Recently, inorganic nanomaterials have received considerable attention for use in biomedical applications owing to their unique physicochemical properties based on their shapes, sizes, and surface characteristics. Photodynamic therapy (PDT), sonodynamic therapy (SDT), and chemical dynamic therapy (CDT), which are cancer therapeutics mediated by reactive oxygen species (ROS), have the potential to significantly enhance the therapeutic precision and efficacy for cancer. To facilitate cancer therapeutics, numerous inorganic nanomaterials have been developed to generate ROS. This mini review provides an overview of the generation mechanisms of ROS by representative inorganic nanomaterials for cancer therapeutics, including the structures of engineered inorganic nanomaterials, ROS production conditions, ROS types, and the applications of the inorganic nanomaterials in cancer PDT, SDT, and CDT.
Introduction
Reactive oxygen species (ROS) are reactive radicals or non-radical oxygen-containing molecules with reactive properties. ROS primarily include singlet oxygen (1O2), hydrogen peroxide (H2O2), hydroxyl radical (•OH), and superoxide anion (O2•−) (Yang et al., 2019). Intrinsic ROS are derived from various metabolic reactions that mainly occur in the mitochondria. These reactions are essential for the development of living organisms. The overexpression of ROS causes oxidative stress and can lead to the destruction of cellular components. This property is beneficial for the design of ROS-related inorganic nanomaterials for therapeutic applications, including PDT, SDT, and CDT (Yang et al., 2019). PDT is a therapeutic strategy in which a photosensitizer (PS) or a photosensitizing nanoplatform selectively absorbs light of a specific wavelength to produce ROS, which can cause cell death (Liu et al., 2020a). SDT employs high-penetrating acoustic waves to trigger the ultrasound-responsive materials generating ROS for the cancer treatment (Wu et al., 2019). CDT agents can effectively kill cancer cells by catalyzing H2O2 production in the tumor microenvironment (TME) (Lin et al., 2018). Therefore, ROS-related cancer therapeutics show potential to significantly enhance the precision and efficacy of cancer therapeutics.
Given the tremendous progress in the field of nanotechnology, a large number of inorganic nanomaterials with intrinsic ROS-regulating properties have been prepared for widespread biomedical applications. This mini review provides an overview of the mechanisms by which representative inorganic nanomaterials generate ROS for cancer therapeutics, including the structures of engineered inorganic nanomaterials, ROS production conditions, ROS types, and the applications of the nanomaterials in cancer PDT, SDT, and CDT.
Inorganic Nanomaterials and Their Mechanisms of ROS Generation
Remarkable progress has been made with respect to inorganic nanomaterials that can facilitate intrinsic ROS generation. However, the mechanisms of ROS generation by representative inorganic nanomaterials are rarely summarized. This section discusses the mechanisms of ROS generation by inorganic nanomaterials including carbon nanomaterials, semiconductor nanomaterials, defective inorganic nanomaterials, and Fenton agents.
Carbon Nanomaterials Generate ROS Mechanisms
Carbon nanomaterials are a class of carbonaceous materials, including fullerenes, carbon dots (CDs), carbon nanotubes, carbon nanohorns, graphene (GR), and graphene oxide (GO) (Panwar et al., 2019). The fundamental structures of carbon nanomaterials are based on sp2-hybridized carbon atoms that are generally arranged in hexagonal lattices which can form various topological distortions (Karousis et al., 2016). This endows carbon nanomaterials with unique physical and chemical properties (Panwar et al., 2019), leading to numerous applications in biosensing, bioimaging, and biomedicine (Cui et al., 2018). Since many carbon nanomaterials have shown great potential for ROS generation, they are expected to be applicable as activatable PSs for PDT. However, the mechanisms of ROS generation by different carbon materials are diverse.
Liu et al. investigated the relationship between oxidative stress and the surface areas of various graphenic carbon materials. They found that the reaction between graphenic carbon materials and dissolved dioxygen usually takes place at the graphenic edge or defect sites, generating ROS such as O2•− and H2O2via a heterogeneous process; these ROS then oxidize GSH (Figure 1A) (Liu et al., 2011). Dong et al. also discovered the electrocatalytic activity depended on the content of topological C defects (Dong et al., 2020). When two-dimensional graphene sheets were converted to zero-dimensional CDs, phenomena associated with edge effects and quantum confinement were observed due to size effects. In 2014, Ge et al. developed a type of graphene quantum dot (GQD) with PDT properties. The GQDs demonstrated an 1O2 quantum yield of more than 1.3 via a multistate sensitization process. As shown in Figure 1B, the high 1O2 yield of the GQDs is derived from two pathways: the conventional pathway, namely, energy transfer from the excited triplet state (T1) to the ground state (S0); and energy transfer from the excited singlet state (S1) to T1via the intersystem crossing transition. Consequently, the 1O2 quantum yield reached 1.3 (Ge et al., 2014). Subsequently, Shen’s group prepared hyaluronic acid (HA)-derived CDs which can produce O2•−via a photoinduced electron transfer process (Zhang et al., 2018). These studies demonstrate that pure CDs can produce ROS upon photoexcitation owning to the band-edge defect effect. Therefore, it can improve the ROS generation by adjusting the edge defects of carbon materials.
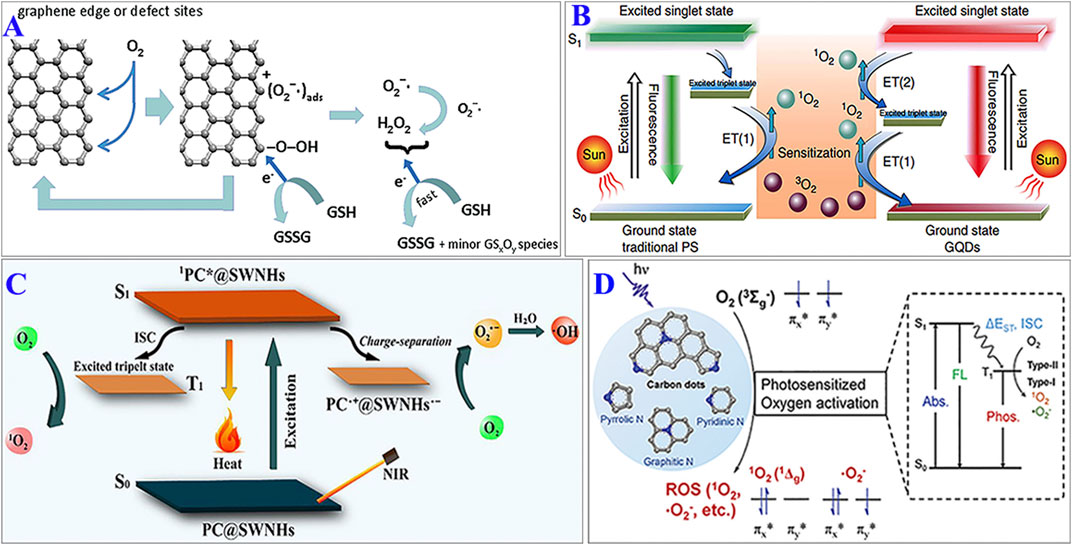
FIGURE 1. (A) Defect sites in carbon nanomaterials and ROS generation. Reprinted with permission from Liu et al. Copyright (2011) Wiley-VCH. (B) Schematic of the mechanisms of 1O2 production by traditional PDT agents (left) and GQDs (right). Reprinted with permission from Ge et al. Copyright (2014) Nature Publishing Group. (C) Schematic of the mechanism of ROS generation by PC@SWNHs under 650-nm laser irradiation. Reprinted with permission from Lin et al. Copyright (2019) Elsevier. (D) Nitrogen-doped CDs for photosensitized oxygen activation. Reprinted with permission from Wu et al. Copyright (2020) The Royal Society of Chemistry.
Surface functionalization is an effective strategy to improve the photosensitization performance of CDs. For example, Shen et al. used tetrasulfonic acid copper phthalocyanine (TSCuPc) as a PS and GR or single-walled carbon nanohorns (SWNHs) as PS carriers to prepare water-soluble GR-TSCuPc and SWNHs-TSCuPc nanohybrids. These GR-TSCuPc and SWNHs-TSCuPc nanohybrids demonstrated excellent ROS production ability. When TSCuPc is photoexcited, electron transfer occurs from the excited state of TSCuPc to the SWNHs (or GR). This results in the formation of a charge-separated state (SWNHs•−–TSCuPc•+ or GR•−–TSCuPc•+), which further transfers the electrons to surrounding O2 to generate O2•− and then •OH (Jiang et al., 2014a; Jiang et al., 2014b). In another works, the functionalized SWNHs exhibited the similar •OH generation process. Meanwhile, 1O2 was generated via the conventional energy transfer pathway from T1 to S0 (Figure 1C) (Lin et al., 2019a). These works indicated surface functionalization can induce ROS generation.
Heteroatom doping is an effective approach to improve the surface functionalization of CDs, which is important for ROS generation. Wu’s group synthesized a series of N-doped CDs (N-CDs) with a variety of N types to explore how the speciation of the nitrogen dopant affected the oxygen photosensitization performance of the N-CDs. It is found that among N types, graphitic N and pyrrolic N had the greatest effects on the photosensitization properties of CDs. The presence of graphitic N in N-CDs can reduce the energy gap between S1 and T1 (ΔEST) to promote ISC, which can improve the photosensitization performance. Pyrrolic N was the main site of oxygen adsorption, which contributed to the highly efficient oxygen photosensitization of N-CDs by shortening the distance between oxygen and the N-CDs (Figure 1D) (Wu et al., 2020). The Cu-doped CDs can also effectively generate ROS (Wu et al., 2015; Guo et al., 2018). These results indicate that heteroatom doping is an effective strategy to improve ROS generation by CDs.
Semiconductor Nanomaterials Generate ROS Mechanism
Semiconductor nanomaterials have excellent prospects for applications in photocatalysis due to their distinct physical and chemical properties. It is found that semiconductors can absorb sunlight to catalyze different photochemical reactions, and ROS are the primary intermediates in photocatalytic reactions (Nosaka and Nosaka, 2017). The generated ROS in photocatalytic reaction mechanism are executed by redox reactions via oxygen and water with photogenerated electrons (e−) and holes (h+) on the surface of the semiconductor photocatalyst. Thus, semiconductor photocatalysts have great potential for use as PSs for PDT. Studies have extended the optical responses of semiconductor photocatalysts into the visible and even NIR regions by introducing doping atoms or surface defects to modify the electronic band structure and improve the overall catalytic efficiency. For example, Qin et al. prepared a novel photocatalyst B@ZrO2−OV that can achieve photon harvesting in the full spectrum from visible light to NIR light (Figure 2A). The doped B atoms and OVs into ZrO2 result in more impurity electronic states and narrow bandgap, resulting in full spectrum absorption, and can efficiently produce electron−hole pairs under the NIR light irradiation, and react with adsorbed O2/H2O on the surface to produce O2•− and •OH (Qin et al., 2020). The surface modification or functionalization of semiconductors can also improve their biocompatibility and delivery efficiency in tumors. This full-spectrum responsive B@ZrO2−OV was modified with HA to obtain ZrO2−x−B@SiO2−HA, which exhibits improved water dispersibility and the ability to target tumor cells (Figure 2B). The obtained ZrO2−x−B@SiO2−HA also shows promise for high-resolution photoacoustic imaging along with remarkable synergistic PTT/PDT treatment effect upon 1064-nm laser irradiation (Zhu et al., 2020). Mou et al. developed green titania (G−TiO2−x) with excellent NIR absorption by altering the lattice of black titania (B−TiO2−x) via strong ultrasonication, and further conjugated G−TiO2−x with triphenylphosphonium (TPP) ligand to obtain G−TiO2−x−TPP, which exhibits mitochondria-targeting ability for combined PTT/PDT precise cancer therapy (Figure 2C) (Mou et al., 2017). These works have significantly broadened the application of semiconductors in nanomedicine through reasonable design and control of the nanostructures and physicochemical properties of semiconductor-based nanomaterials.
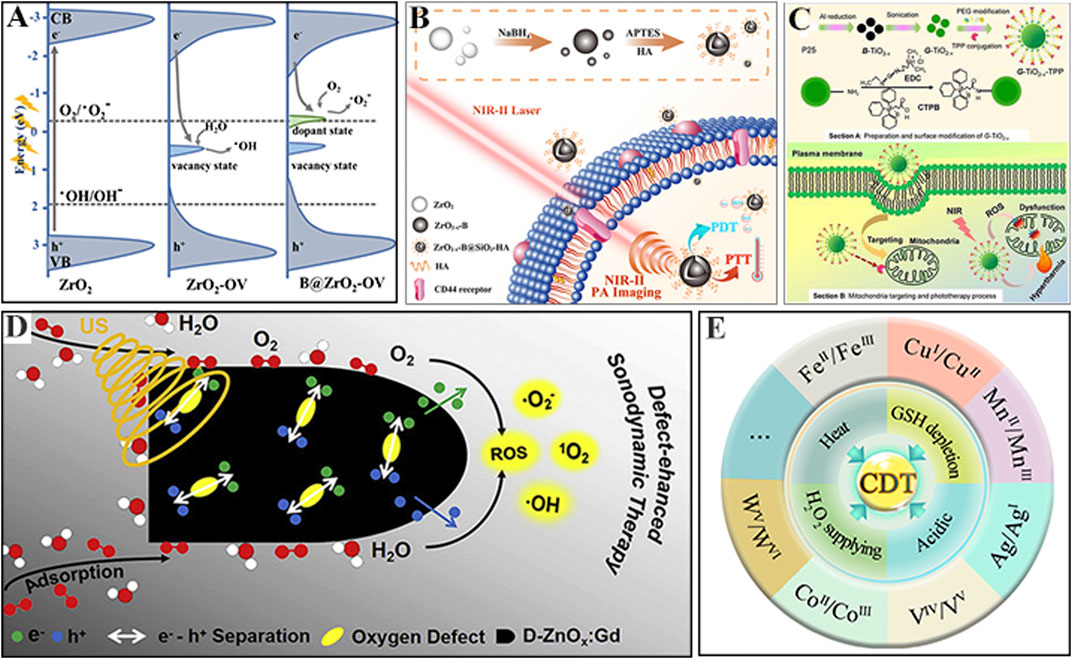
FIGURE 2. (A) Band edge alignment of B@ZrO2−OV for visible-to-NIR photon harvesting. Reprinted with permission from Qin et al. Copyright (2020) American Chemical Society. (B) Design of NIR-responsive ZrO2-x−B@SiO2−HA for cancer phototherapy. Reprinted with permission from Zhu et al. Copyright (2020) The Royal Society of Chemistry. (C) Design and schematic of G-TiO2-x-TPP with excellent NIR absorption for cancer treatment. Reprinted with permission from Mou et al. Copyright (2017) Ivyspring. (D) The proposed mechanism of defect-enhanced sonodynamic ROS generation in D−ZnOx:Gd. Reprinted with permission from Liu et al. Copyright (2020b) Elsevier. (E) Summary of metal-based inorganic nanomaterials for CDT and strategies for improving CDT.
Defective Inorganic Nanomaterials for ROS-Generation
In addition to carbon nanomaterials and semiconductors, defective inorganic nanomaterials have also been shown to generate ROS for cancer therapeutics. In the defect engineering of inorganic nanomaterials, the particle size, morphology, and crystal defects are controlled with appropriate synthetic methods so that the ROS generation activity can be tuned (Qin et al., 2020). This strategy has been used to produce many defective inorganic nanomaterials for ROS-related cancer therapeutics (Han et al., 2018). Therefore, the mechanism of defect-mediated tunable ROS generation by inorganic nanomaterials should be studied. Recently, Shen’s group successfully developed one type of brand-new artificial metalloprotein nanoanalogues (RuO2@OVA NAs) for enhanced photoimmunotherapy via the reasonable integration of defective RuO2 NPs and the model antigen ovalbumin (OVA) (Huang et al., 2020). The following possible mechanism of photoinduced 1O2 production by defective RuO2 NPs in RuO2@OVA NAs was proposed: O2 is sensitized to 1O2 by defects via energy transfer under 808-nm laser irradiation. This work significantly broadens the nanomedical applications of RuO2-based nanomaterials via the rational design of structural defects.
Defective inorganic nanomaterials are also employed as sonosensitizers to generate ROS for the cancer treatment through different mechanisms such as the sonoluminescence process, pyrolysis, and acoustic cavitation e
Fenton Agents for ROS Generation
Fenton and Fenton-like reactions have attracted extensive attention as an emerging cancer treatment that can transfer endogenous hydrogen peroxide (H2O2) into highly cytotoxic •OH. As shown in Figure 2E, although the majority of existing Fenton agents for cancer therapy are iron-based inorganic nanomaterials, other inorganic redox-active transition metals such as Cu, Mn, Ag, V, Co, and W along with nanozymes (e.g., Fe3O4) have been used to induce Fenton-like reactions (Han et al., 2020). For example, Lin et al. showed that manganese dioxide (MnO2) has dual Fenton-like Mn2+ delivery and GSH depletion properties, and can thus be used as a smart Fenton-like agent to deliver •OH into cells to destroy the cellular antioxidant defense system and thereby enhancing the CDT effect (Lin et al., 2018). Bu et al. developed AFeNPs@CAI nanocomposites from amorphous iron nanoparticles (AFeNPs) loaded with carbonic anhydrase IX inhibitor (CAI). The release of CAI led to the accumulation of H+, which accelerated the Fenton reaction and amplified the oxidative damage of cells (Chen et al., 2019). Fenton-like reactions catalyzed by Cu+ are kinetically more favorable than those catalyzed by Fe2+ under neutral and weakly acidic conditions. To further improve the reaction efficiency of Cu-based nanomaterials, intracellular H2O2 may be challenging. Li et al. reported self-assembled copper amino acid mercaptide nanoparticles induce •OH generation via Fenton-like reaction through their sequential oxidation–reduction reactions with intracellular GSH “AND” H2O2 (Ma et al., 2019). Chen et al. reported the fabrication of copper peroxide (CP) nanodots that enhance CDT by self-supplying H2O2. In the TME, the acidic environment of endo/lysosomes accelerates the dissociation of CP nanodots, simultaneously releasing Cu2+ and H2O2, which improves the efficiency of the Fenton-like reaction (Lin et al., 2019b).
Nanozymes are a class of nanomaterials with inherently enzyme-like properties. In 2007, Yan et al. first reported that Fe3O4 nanoparticles have intrinsic peroxidase-mimicking activity (Gao et al., 2007). The Fenton reaction properties of Fe3O4 have been reported in cancer treatment. For example, Shi et al. simultaneously loaded the natural enzyme glucose oxidase (GOD) and nanozyme Fe3O4 into large pore-sized and biodegradable dendritic silica nanoparticles to construct sequential GFD nanocatalysts. GOD can effectively consume glucose in tumor cells while also producing a large amount of H2O2 and reducing the acidity, which are used in the Fenton-like reaction of the nanoenzyme Fe3O4 to generate •OH (Huo et al., 2017). In 2020, Chen et al. synthesized vanadium oxide–based nanozymes (TA@VOx NSs) could catalyze H2O2 to generate •OH. Importantly, the authors also demonstrated that heat can effectively improve the •OH generation efficiency of Fenton-like reaction (Chen et al., 2020). The above studies show that various inorganic nanomaterials and strategies have been applied to enhance the efficiency of Fenton and Fenton-like reactions for ROS generation. These efforts can greatly promote the clinical application of Fenton and Fenton-like reactions in cancer treatment.
Conclusion and Challenges
This brief overview focuses on the mechanisms of ROS generation by representative inorganic nanomaterials for cancer therapeutics. Although various inorganic nanomaterials have been reported to generate ROS, several remaining challenges limit the precision and efficacy of ROS-based cancer therapeutics:
1. Inadequate accumulation in cancer tissues and relevant systemic toxicity. Although inorganic nanomaterials can effectively catalyze the production of ROS, their biodegradation mechanism in vivo remains unclear, and the lack of specificity in the delivery and distribution of inorganic nanomaterials in normal tissues is still a problem that needs to be solved (Qian et al., 2019).
2. The TME allows CDT, PDT, and SDT to be selective and minimally invasive; however, the hypoxic conditions, limited concentration of intracellular H2O2, and overexpression of GSH in the TME significantly reduce the efficacy of ROS-based cancer therapy. Therefore, it is necessary to develop an intelligent strategy to modulate the TME (Yin et al., 2019).
3. The ROS is a double-edged sword. On the one hand, ROS can induce cell apoptosis in PDT, SDT, and CDT. On the other hand, the ROS produced by foodborne CDs could disrupt the mitochondrial membrane and changed the transcriptional level of genes related to ROS, which will be the potential health risk after digestion and absorption (Wang et al., 2020).
Moreover, organic nanomaterials have been reported to generate ROS which were mainly through fluorescence resonance energy transfer (FRET)-mediated direct or indirect sensitization of oxygen molecules process (Ng and Zheng, 2015). Therefore, a comprehensive understanding of the mechanism of ROS generation will be conducive to the design and construction of nanomaterials that will play important roles in precise and effective cancer therapeutics.
Author Contributions
All authors listed have made a substantial, direct, and intellectual contribution to the work and approved it for publication.
Funding
This work was supported by National Natural Science Foundation of China (21977022, 21671046 and 51762007), the Natural Science Foundation of Guangxi Province (2017GXNSFGA198004, 2017GXNSFBA198208, and GuikeAD19110018).
Conflict of Interest
The authors declare that the research was conducted in the absence of any commercial or financial relationships that could be construed as a potential conflict of interest.
References
Chen, T., Huang, R., Liang, J., Zhou, B., Guo, X.-L., Shen, X.-C., et al. (2020). Natural polyphenol-vanadium oxide nanozymes for synergistic chemodynamic/photothermal therapy. Chem. Eur. J. 26, 1–12. doi:10.1002/chem.202002335
Chen, X., Zhang, H., Zhang, M., Zhao, P., Song, R., Gong, T., et al. (2019). Amorphous Fe-based nanoagents for self-enhanced chemodynamic therapy by re-establishing tumor acidosis. Adv. Funct. Mater. 30, 1908365. doi:10.1002/adfm.201908365
Cui, X., Xu, S., Wang, X., and Chen, C. (2018). The nano-bio interaction and biomedical applications of carbon nanomaterials. Carbon 138, 436–450. doi:10.1016/j.carbon.2018.07.069
Dong, Y., Zhang, Q., Tian, Z., Li, B., Yan, W., Wang, S., et al. (2020). Ammonia thermal treatment toward topological defects in porous carbon for enhanced carbon dioxide electroreduction. Adv. Mater. 32, 2001300. doi:10.1002/adma.202001300
Gao, L., Zhuang, J., Nie, L., Zhang, J., Zhang, Y., Gu, N., et al. (2007). Intrinsic peroxidase-like activity of ferromagnetic nanoparticles. Nat. Nanotechnol. 2, 577–583. doi:10.1038/nnano.2007.260
Ge, J., Lan, M., Zhou, B., Liu, W., Guo, L., Wang, H., et al. (2014). A graphene quantum dot photodynamic therapy agent with high singlet oxygen generation. Nat. Commun. 5, 4596. doi:10.1038/ncomms5596
Guo, X.-L., Ding, Z.-Y., Deng, S.-M., Wen, C.-C., Shen, X.-C., Jiang, B.-P., et al. (2018). A novel strategy of transition-metal doping to engineer absorption of carbon dots for near-infrared photothermal/photodynamic therapies. Carbon 134, 519–530. doi:10.1016/j.carbon.2018.04.001
Han, X., Huang, J., Jing, X., Yang, D., Lin, H., Wang, Z., et al. (2018). Oxygen-deficient black titania for synergistic/enhanced sonodynamic and photoinduced cancer therapy at near infrared-II biowindow. ACS Nano 12, 4545–4555. doi:10.1021/acsnano.8b00899
Han, Y., Gao, S., Zhang, Y., Ni, Q., Li, Z., Liang, X.-J., et al. (2020). Metal-based nanocatalyst for combined cancer therapeutics. Bioconjug. Chem. 31, 1247–1258. doi:10.1021/acs.bioconjchem.0c00194
Huang, R., Ding, Z., Jiang, B.-P., Luo, Z., Liang, H., Shen, X.-C., et al. (2020). Artificial metalloprotein nanoanalogues: in situ catalytic production of oxygen to enhance photoimmunotherapeutic inhibition of primary and abscopal tumor growth. Small 16, 2004345. doi:10.1002/smll.202004345
Huo, M., Wang, L., Chen, Y., and Shi, J. (2017). Tumor-selective catalytic nanomedicine by nanocatalyst delivery. Nat. Commun. 8, 357. doi:10.1038/s41467-017-00424-8
Jiang, B. P., Hu, L. F., Shen, X. C., Ji, S. C., Shi, Z., Liu, C. J., et al. (2014a). One-step preparation of a water-soluble carbon nanohorn/phthalocyanine hybrid for dual-modality photothermal and photodynamic therapy. ACS Appl. Mater. Interfaces 6, 18008–18017. doi:10.1021/am504860c
Jiang, B. P., Hu, L. F., Wang, D. J., Ji, S. C., Shen, X. C., and Liang, H. (2014b). Graphene loading water-soluble phthalocyanine for dual-modality photothermal/photodynamic therapy via a one-step method. J. Mater. Chem. B 2, 7141–7148. doi:10.1039/c4tb01038h
Karousis, N., Suarez-Martinez, I., Ewels, C. P., and Tagmatarchis, N. (2016). Structure, properties, functionalization, and applications of carbon nanohorns. Chem. Rev. 116, 4850–4883. doi:10.1021/acs.chemrev.5b00611
Lin, Z., Jiang, B.-P., Liang, J., Wen, C., and Shen, X.-C. (2019a). Phycocyanin functionalized single-walled carbon nanohorns hybrid for near-infrared light-mediated cancer phototheranostics. Carbon 143, 814–827. doi:10.1016/j.carbon.2018.12.011
Lin, L. S., Huang, T., Song, J., Ou, X. Y., Wang, Z., Deng, H., et al. (2019b). Synthesis of copper peroxide nanodots for H2O2 self-supplying chemodynamic therapy. J. Am. Chem. Soc. 141, 9937–9945. doi:10.1021/jacs.9b03457
Lin, L. S., Song, J., Song, L., Ke, K., Liu, Y., Zhou, Z., et al. (2018). Simultaneous fenton-like ion delivery and glutathione depletion by MnO2 -based nanoagent to enhance chemodynamic therapy. Angew. Chem. Int. Ed. Engl. 57, 4902–4906. doi:10.1002/anie.201712027
Liu, H., Yao, J., Guo, H., Cai, X., Jiang, Y., Lin, M., et al. (2020a). Tumor microenvironment-responsive nanomaterials as targeted delivery carriers for photodynamic anticancer therapy. Front. Chem. 8, 758. doi:10.3389/fchem.2020.00758
Liu, Y., Wang, Y., Zhen, W., Wang, Y., Zhang, S., Zhao, Y., et al. (2020b). Defect modified zinc oxide with augmenting sonodynamic reactive oxygen species generationed zinc oxide with augmenting sonodynamic reactive oxygen species generation. Biomaterials 251, 120075. doi:10.1016/j.biomaterials.2020.120075
Liu, H., Cheng, R., Dong, X., Wang, Q., Gu, Z., Zhao, Y., et al. (2020c). BiO2−x nanosheets as radiosensitizers with catalase-like activity for hypoxia alleviation and enhancement of the radiotherapy of tumors. Inorg. Chem. 59, 3482–3493. doi:10.1021/acs.inorgchem.9b03280
Liu, X., Sen, S., Liu, J., Kulaots, I., Geohegan, D., Kane, A., et al. (2011). Antioxidant deactivation on graphenic nanocarbon surfaces. Small 7, 2775–2785. doi:10.1002/smll.201100651
Ma, B., Wang, S., Liu, F., Zhang, S., Duan, J., Li, Z., et al. (2019). Self-assembled copper-amino acid nanoparticles for in situ glutathione “AND” H2O2 sequentially triggered chemodynamic therapy. J. Am. Chem. Soc. 141, 849–857. doi:10.1021/jacs.8b08714
Mou, J., Lin, T., Huang, F., Shi, J., and Chen, H. (2017). A new green titania with enhanced NIR absorption for mitochondria-targeted cancer therapy. Theranostics 7, 1531–1542. doi:10.7150/thno.17247
Ng, K. K., and Zheng, G. (2015). Molecular interactions in organic nanoparticles for phototheranostic applications. Chem. Rev. 115 (19), 11012–11042. doi:10.1021/acs.chemrev.5b00140
Nosaka, Y., and Nosaka, A. Y. (2017). Generation and detection of reactive oxygen species in photocatalysis. Chem. Rev. 117, 11302–11336. doi:10.1021/acs.chemrev.7b00161
Panwar, N., Soehartono, A. M., Chan, K. K., Zeng, S., Xu, G., Qu, J., et al. (2019). Nanocarbons for biology and medicine: sensing, imaging, and drug delivery. Chem. Rev. 119, 9559–9656. doi:10.1021/acs.chemrev.9b00099
Qian, X., Zhang, J., Gu, Z., and Chen, Y. (2019). Nanocatalysts-augmented Fenton chemical reaction for nanocatalytic tumor therapy. Biomaterials 211, 1–13. doi:10.1016/j.biomaterials.2019.04.023
Qin, Y., Ding, Z., Guo, W., Guo, X., Hou, C., Jiang, B.-P., et al. (2020). A full solar light spectrum responsive B@ZrO2-OV photocatalyst: a synergistic strategy for visible-to-NIR photon harvesting. ACS Sustain. Chem. Eng. 8, 13039–13047. doi:10.1021/acssuschemeng.0c04380
Wang, H., Su, W., and Tan, M. (2020). Endogenous fluorescence carbon dots derived from food items. Innov. 1, 100009. doi:10.1016/j.xinn.2020.04.009
Wu, M., Ding, Y., and Li, L. (2019). Recent progress in the augmentation of reactive species with nanoplatforms for cancer therapy. Nanoscale 11, 19658–19683. doi:10.1039/c9nr06651a
Wu, S., Zhou, R., Chen, H., Zhang, J., and Wu, P. (2020). Highly efficient oxygen photosensitization of carbon dots: the role of nitrogen doping. Nanoscale 12, 5543–5553. doi:10.1039/c9nr10986b
Wu, W., Zhan, L., Fan, W., Song, J., Li, X., Li, Z., et al. (2015). Cu-N dopants boost electron transfer and photooxidation reactions of carbon dots. Angew. Chem. Int. Ed. Engl. 54, 6540–6544. doi:10.1002/ange.20150191210.1002/anie.201501912
Yang, B., Chen, Y., and Shi, J. (2019). Reactive oxygen species (ROS)-based nanomedicine. Chem. Rev. 119, 4881–4985. doi:10.1021/acs.chemrev.8b00626
Yin, S.-Y., Song, G., Yang, Y., Zhao, Y., Wang, P., Zhu, L.-M., et al. (2019). Persistent regulation of tumor microenvironment via circulating catalysis of MnFe2O4@metal–organic frameworks for enhanced photodynamic therapy. Adv. Funct. Mater. 29, 1901417. doi:10.1002/adfm.201901417
Zhang, L., Lin, Z., Yu, Y. X., Jiang, B. P., and Shen, X. C. (2018). Multifunctional hyaluronic acid-derived carbon dots for self-targeted imaging-guided photodynamic therapy. J. Mater. Chem. B 6, 6534–6543. doi:10.1039/c8tb01957f
Keywords: reactive oxygen species, cancer therapeutics, inorganic nanomaterials, generation mechanisms, photodynamic therapy, chemodynamic therapy
Citation: Zhang L, Zhu C, Huang R, Ding Y, Ruan C and Shen X-C (2021) Mechanisms of Reactive Oxygen Species Generated by Inorganic Nanomaterials for Cancer Therapeutics. Front. Chem. 9:630969. doi: 10.3389/fchem.2021.630969
Received: 18 November 2020; Accepted: 25 January 2021;
Published: 18 March 2021.
Edited by:
Kelong Ai, Xiangya School of Medicine, Central South University, ChinaReviewed by:
Linlin Li, Beijing Institute of Nanoenergy and Nanosystems, ChinaYunlu Dai, University of Macau, China
Copyright © 2021 Zhang, Zhu, Huang, Ding, Ruan and Shen. This is an open-access article distributed under the terms of the Creative Commons Attribution License (CC BY). The use, distribution or reproduction in other forums is permitted, provided the original author(s) and the copyright owner(s) are credited and that the original publication in this journal is cited, in accordance with accepted academic practice. No use, distribution or reproduction is permitted which does not comply with these terms.
*Correspondence: Xing-Can Shen, eGNzaGVuQG1haWxib3guZ3hudS5lZHUuY24=