- 1Laboratory of Phytopathology and Microbial Biotechnology, Department of Botany, Mohanlal Sukhadia University, Udaipur, India
- 2Centre of Advanced Study in Botany, Institute of Science, Banaras Hindu University, Varanasi, India
- 3Department of Botany, Acharya Narendra Dev College, University of Delhi, New Delhi, India
- 4Plant Biotechnology Laboratory, Department of Botany, Mohanlal Sukhadia University, Udaipur, India
- 5Department of Biotechnology, Vigyan Bhawan, Mohanlal Sukhadia University, Udaipur, India
Nanotechnology has become a very advanced and popular form of technology with huge potentials. Nanotechnology has been very well explored in the fields of electronics, automobiles, construction, medicine, and cosmetics, but the exploration of nanotecnology’s use in agriculture is still limited. Due to climate change, each year around 40% of crops face abiotic and biotic stress; with the global demand for food increasing, nanotechnology is seen as the best method to mitigate challenges in disease management in crops by reducing the use of chemical inputs such as herbicides, pesticides, and fungicides. The use of these toxic chemicals is potentially harmful to humans and the environment. Therefore, using NPs as fungicides/ bactericides or as nanofertilizers, due to their small size and high surface area with high reactivity, reduces the problems in plant disease management. There are several methods that have been used to synthesize NPs, such as physical and chemical methods. Specially, we need ecofriendly and nontoxic methods for the synthesis of NPs. Some biological organisms like plants, algae, yeast, bacteria, actinomycetes, and fungi have emerged as superlative candidates for the biological synthesis of NPs (also considered as green synthesis). Among these biological methods, endophytic microorganisms have been widely used to synthesize NPs with low metallic ions, which opens a new possibility on the edge of biological nanotechnology. In this review, we will have discussed the different methods of synthesis of NPs, such as top-down, bottom-up, and green synthesis (specially including endophytic microorganisms) methods, their mechanisms, different forms of NPs, such as magnesium oxide nanoparticles (MgO-NPs), copper nanoparticles (Cu-NPs), chitosan nanoparticles (CS-NPs), β-d-glucan nanoparticles (GNPs), and engineered nanoparticles (quantum dots, metalloids, nonmetals, carbon nanomaterials, dendrimers, and liposomes), and their molecular approaches in various aspects. At the molecular level, nanoparticles, such as mesoporous silica nanoparticles (MSN) and RNA-interference molecules, can also be used as molecular tools to carry genetic material during genetic engineering of plants. In plant disease management, NPs can be used as biosensors to diagnose the disease.
Introduction
In recent years, nanomaterials have emerged as a novel type of material (Tayo, 2017; Hu et al., 2020). Nanotechnology is the latest technology with options for utilization in different fields like biology, sensing, medicine, chemistry and physics (Ramalingam et al., 2014; Ramalingam, 2019). Due to having various shapes and structures such as nanorods, nanospheres, nanocubes, nanobipyramids, nanobranches, nanoflowers, nanowires, nanocages, and nanoshells, nanomaterials appeared as the most stable materials (Li et al., 2015; Ramalingam et al., 2019; Xiao et al., 2019; Barupal et al., 2020a; Barupal et al., 2020b). Nanomaterials have unique electrical and optical properties that can be synthesized by different ways at low cost and have wide applications in several interdisciplinary branches of science (Gurav et al., 2019; Khan I. et al., 2019). Nano (dwarf) is the greek prefix which refers to the very small which in terms of nanoparticles, can refer to sizes up to 10–9 m i.e., one thousand millionth of a meter (Bayda et al., 2020; Chandran et al., 2020a; Chandran et al., 2020b). Nanotechnology belongs to the nanoscience in which nano-size molecules (1–100 nm) are utilized through practical applications using devices (Kumar and Kumbhat, 2016; Bayda et al., 2020). The term “nanotechnology” was first given by Taniguchi in 1974 to describe that which deals with the synthesis and application of nano-size particles (100 nm) (Filipponi, et al., 2010; Khan and Rizivi, 2014; El-Sayed and Kamel, 2020). According to the National Nanotechnology Initiative (NNI) United States, Nanotechnology is defined as a field of science, engineering, and technology where materials are practicised at the nanoscale size (1–100 nm), using unique phenomena in a wide range of biology, physics, chemistry, medicine, electronics and engineering fields (Chen et al., 2007; Lu et al., 2012; Kumari et al., 2018a; Kumari et al., 2018b). The most important properties of these nanoparticles (NPs) are their size which can manipulate the physiochemical and optical properties of a particular substance (Meena et al., 2015; Khan M. R. et al., 2019).
Different NPs, such as gold (Au), silver (Ag), nickel (Ni), platinum (Pt), titanium (Ti), zinc (Zn), and palladiumn (Pd) are synthesized in various shapes and colors for the delivery of chemical, biological sensing, bioimaging (Dreaden et al., 2012; Bareket et al., 2016; Islam et al., 2018; Yew et al., 2020; Figure 1), gas sensing (Mansha et al., 2016; Ullah et al., 2017; Zhang H. et al., 2019), capturing of CO2 (Ramacharyulu et al., 2015; Ganesh et al., 2017), and other related applications. NPs are composed of three layers. The first layer known as surface layer which is composed of various types of small molecules, surfactants, metal ions, and polymers which functionalized the NPs. The second layer consists of as a shell layer, composed of different chemical materials as compared to the core. The core is the central part of the NP and generally refers to the NP itself (Shin et al., 2016; Heinz et al., 2017). Due to such remarkable characteristics, these materials gained considerable interest from researchers in multi-disciplinary areas. Mesoporousity imparts additional characteristics to NPs (Khan I. et al., 2019). In this review article, we provided a common overview related to NPs such as their different types, methods for synthesis, characterizations, properties, and their applications. The green synthesis of nanoparticles, specifically endophytic microorganism associated synthesis is a more beneficial method as compared to other physical and chemical methods such as top-down and bottom up methods, due to it being ecofriendly and cost-effective with significant morphology and size (Messaoudi and Bendahou, 2020). Micoorganism mediated synthesis of NPs is a challenging green process to manufacture NPs (Grasso et al., 2020). NPs are produced by microorganisms either through intracellular or extracellular process based on the location of enzymatic activity involved (Messaoudi and Bendahou, 2020). Microbial-mediated NPs synthesis showed advantage over the biosynthesis of NPs by algae and plants (Rana et al., 2020). Endophytic methods earned more attention in the field of medical, pharmaceuticals, environmental and agronomical applications (Gour and Jain, 2019; Rana et al., 2020). The last section of this review is used to discuss the future aspects and recommendations of NPs.
Classification of Nanoparticles
Recently, various categories of NPs and their derivatives have been reported to have effective antimicrobial properties on the basis of their size, morphology and chemical properties. These derivatives of NPs can be Au, Ag, Cu, Ni, Pt, Ti, and Zn. Well-known classes of NPs have been described below on the basis of their physical and chemical characteristics.
Organic Nanoparticles
Organic NPs are solid particles ranging between 10 nm to 1 μm in diameter and consist of organic compounds like polymeric or lipids (Kumar and Lal, 2014). Organic NPs received little attention as compared to inorganic NPs. In recent years, the pharmaceutical industries led the research into the synthesis of organic NPs. The search for nano-medicine developed well-established techniques to synthesize novel materials. They have an affinity for encapsulating or carrying active molecules as conjugates of proteins, vehicles for DNA delivery, liposomes, and co-polymer micelles (Ulbrich et al., 2016). Organic compounds are inherently and ultimately slow soluble in water or aqueous environments as compared to their inorganic counterparts, but organic NPs will not remain in the environment for a long period which makes them environmentally friendly (Yu et al., 2018). There are some well-known organic NPs that have been reported, such as dendrimers, micelles, liposomes, and ferritin, that showed some characteristics properties such as non-toxicity and biodegradability (Lee et al., 2012). Micelles and liposomes have a hollow core, also known as nanocapsules, and are considered as more sensitive to thermal and electromagnetic radiation such as heat and light (Ealias and Saravanakumar, 2017). Therefore, these unique characteristics make them an ideal alternative for drug delivery. The drug-carrying capacity, stability and delivery systems of organic NPs, either in the form of entrapped drugs or adsorbed drugs system determines their field applications and their effectiveness (Ealias and Saravanakumar, 2017; Meena et al., 2020a; Meena et al., 2020b). Organic NPs are also most widely used in the biomedical field (Yang et al., 2019).
Carbon Nanomaterials
Carbon nanomaterials vary in shape, size, and function. There are three categories of carbon nanomaterials that are recognized: carbon nanotubes, graphene oxides, and fullerenes. The wall of carbon nanotubes can be single or multi, whereas graphene oxides and fullerenes are oxidized/reduced and C60 (buckyballs), respectively. Carbon nanomaterials are used in textiles engineering and medicines fields due to having antimicrobial activities against bacteria (Liu et al., 2009; Wang et al., 2013) and fungi (Sarlak et al., 2014; Wang X. et al., 2014), and also have been demonstrated and investigated as plant growth enhancers (Khodakovskaya et al., 2009; Tripathi et al., 2011; Wang et al., 2012; Elmer and White, 2018). Recently, it has been illustrated that carbon nanomaterials play a significant role in plant pathology. The reduced form of graphene oxide decreases 50% radial growth of Aspergillus oryzae, Aspergillus niger, and Fusarium oxysporum on agar plate at different concentration (100, 50, and 100 μg/ml) of graphene oxide. Single-walled carbon nanotubes were found to be more toxic to conidia of Fusarium poae and Fusarium graminearum (Wang X. et al., 2014). Nowadays, carbon nanotubes are explored as a phytosanitary treatment of pecan infected with Xylella fastidiosa (Hilton et al., 2017). Carbon nanomaterials open new areas of microbiological research by uncovering microbial growth inhibition mechanisms (Liu et al., 2009; Sawangphruk et al., 2012; Chen et al., 2013; Berry et al., 2014; Wang Y. et al., 2014). In Fusarium sp. the inhibition mechanism is governed by carbon nanotubes (single-walled) through the mechanism of water uptake and plasmolysis induction (Wang L. et al., 2017). Certain forms of carbon nanomaterials can be produced for antimicrobial activity at relatively low cost and it attracts researchers to develop an evaluation study for carbon nanomaterials in agriculture and other fields (Zehra et al., 2015).
Inorganic Nanoparticles
NPs composed of metal and metal oxide are generally classified as inorganic NPs, which are discussed below.
Metal-Based Nanoparticles
The NPs have characteristic properties such as sizes as low as 10–100 nm, pore size, high surface area to volume ratio, surface charge and density, amorphous and crystalline structures, spherical or cylindrical in shape, colored, high reactivity, and their sensitivity to environmental factors like moisture, air, heat, and sunlight (Ealias and Saravanakumar, 2017). Metal-based NPs are synthesized from metals such as aluminium (Al), cadmium (Cd), cobalt (Co), copper (Cu), gold (Au), iron (Fe), lead (Pb), silver (Ag) and zinc (Zn) (Monych et al., 2019) and can exist in solutions. These NPs have gained much attention in pharmaceutical industries for their use in manufacturing medicines (Padrela et al., 2018). These NPs can be modified by altering their chemical groups to binds with antibodies (Ruiz et al., 2019). Some noble metals such as Ag-, Au-, and Pt- synthesized NPs have specific properties which were used in biomedical fields to cure diseases (Kim et al., 2018). Therefore, these NPs used to prepare drugs had anticancer, radiotherapy enhancement, drug delivery, thermal ablation, antibacterial, diagnostic assays, antifungal, gene delivery, and many other properties (Jahangirian et al., 2017; Sharma et al., 2018). Fan et al. (2018) reported that metal NPs can be target to different cells along with different functional groups, such as peptides, antibodies, RNA, and DNA, and with potential biocompatible polymers (polyethylene glycol; PEG). L-Ascorbic acid has been used to synthesize Cu-NPs with size >2 nm as an antibacterial agent against Gram-negative and Gram-positive bacteria and has been reported as a stabilizer and reducing agent (Tomar and Garg, 2013; Meena et al., 2018; Sathiyabama and Manikandan, 2018).
Au NPs were found useful in the identification of different microorganisms by detecting and evaluating DNA and identifying protein interactions from biological samples. Au-NPs have been used widely and help to detect cancerous cells through bioimaging (Dreaden et al., 2012). They can be synthesized by different processes but are currently being produced by Pseudomonas endophytic microorganisms such as Pseudomonas fluorescens 417 or Fusarium solani (Syed et al., 2016; Clarance et al., 2020). Ag-NPs combined with amoxicillin, penicillin G, clindamycin, vancomycin, and erythromycin showed antimicrobial activities against the pathogenic strains (Rai et al., 2012). Ag NPs play a very important role in biomedicine, performing cell imaging, cancer therapy, genetic delivery, drug delivery, and different disease diagnose (Keat et al., 2015; Shanmuganathan et al., 2019). There are many endpohytic microorganisms that have been involved in AgNPs synthesis such as Bacillus siamensis C1, Pseudomonas poae CO, Aneurinibacillus migulanus, and Alternaria sp. (Ibrahim et al., 2020). Silicon (Si) nano substrates with Ag- or Cu-NPs showed antibacterial activities against Escherichia coli (Fellahi et al., 2013; Shahriary et al., 2018). It has been found that Si coated by Ag is highly biocompatible in the human lung, especially adenocarcinoma epithelial cells, whereas the Cu-coated Si showed high cytotoxicity which may lead to death (Wu et al., 2017). Pd-NPs are more prolific and act as anticancer and stabilizing agents and are used by many pharmaceutical industries to produce medicines (Siddiqi and Husen, 2017; Yaqoob et al., 2020). NPs are being used by many pharmaceutical industries and gained more attention in research fields (Gao and Lowry, 2018).
Metal Oxide–Based Nanoparticles
The metal oxide based NPs such as Ag2O, FeO, MnO2, CuO, Bi2O3, ZnO, MgO, TiO2, CaO, and Al2O3, enhance their activity and were found to have potent antibacterial activities (Yaqoob et al., 2020). The oxide of Ag-NPs (Ag2O) was recommended as a novel source of antibiotics (Torabi et al., 2020) and showed antibiotic properties against E. coli (Salas-Orozco et al., 2019). Whereas, ZnO NPs also showed antibacterial activities against high pressure and temperature tolerant Gram-positive microorganisms (Staphylococcus aureus and Bacillus subtilis), Gram-negative microorganisms (E. coli, Pseudomonas aeruginosa) and spores of Peronospora tabacina as compared to CuO and Fe2O3, NPs respectively (Azam et al., 2012a, b; Prasanna et al., 2019; Wagner et al., 2016). The antibacterial activity of ZnO NPs is inversely proportional to their size (Prasanna et al., 2019). While in the case of TiO2-NPs, its antibacterial activity depends upon its morphology, crystal structure, size, and shape. TiO2 emerged as an important antibacterial agent by enhancing the anti-microorganism effect of tetracycline, β-glycopeptides, aminoglycosides, lactums, cephalosporins, and macrolids against methicillin-resistant Staphylococcus aureus (Roy et al., 2010). It has been also reported that TiO2-NPs enhanced the antifungal activity against Candida albicans biofilms (Haghighi et al., 2013).
CuO-NPs have significant antimicrobial properties against Enterococcus faecalis and E. coli as compared to other various bacterial strains like Klebsiella pneumoniae, Proteus vulgaris, Shigella flexneri, Salmonella typhimurium, P. aeruginosa, and Staphylococcus aureus (Ahamed et al., 2014). As ZnO, CuO, Ag2O, Fe2O3, and TiO2 and some other metal oxide based NPs such as MnO2, Bi2O3, and FeO also showed their beneficial activity in biomedical fields through their use in drug delivery, bioimaging, and antimicrobial activities. FeO-NPs (4.8 nm) showed their higher relativity value (444.56 mM−1 s−1) in the bioimaging of tumor cells (Wang L. et al., 2017; Gao et al., 2018). Some NPs (MnO2) are very significant for medical applications such as bioimaging, biosensing, cancer therapy, molecular adsorption, and drug delivery due to their physicochemical, structural, and morphological-based properties (Chen et al., 2019a; Wu et al., 2019). MnO2 has been considered as a novel compound due to having lower cytotoxicity and higher hemo/histocompatibility. Bi2O3-NPs (35 nm) is recommended for use with phenothiazine photosensitizer for cancer treatment and in drug delivery (Ovsyannikov et al., 2015; Szostak et al., 2019). Some NPs show their activity under a specific environment; for example, CaO and MgO, show their anti-bacterial activity under alkaline and oxygenic environments and are considered as excellent biocompatible NPs. MgO-NPs have been studied as antibacterial agents against E. coli and Staphylococcus aureus under oxygenic conditions (Leung et al., 2016; Meena et al., 2019a; Meena et al., 2019b). Metal oxide based NPs can be synthesized at low cost using simply accessible materials and, can also be utilized in food processing and environmental conservation with biomedical uses. These NPs have excellent properties when compared with their metal counterparts.
Doped Metal/Metal/Metal Oxide–Based NPs
NPs can be modified chemically to make more stable materials that are safe for the ecosystem. The antimicrobial activities of ZnO-NPs against B. subtilis, Staphylococcus aureus, E. coli, and P. aeruginosa can be increased approximately by 5% by doping with Mg (magnesium), Sb (antimony) or Ta (tantalum) as compared to ZnO-NPs and have less self-toxicity issues (Guo et al., 2015). The considerable improvement by approximately 10,000 times was pragmatic in the antimicrobial activity of Zn and CuO-doped NPs as compared to the pure oxide of Cu- and Zn-NPs on the surface of cotton fabric by ultrasound irradiation (Malka et al., 2013). Doped Mn/ZnO NPs have been used to study the antibacterial and photocatalytic activity in pure ZnO-NPs by observing its optical properties and structural morphology; it was found that doped NPs showed more activity (Alshehri and Malik, 2019). TiO2 doped with Cu2O in the presence of rGO results in improved antimicrobial activity with a higher inhibition zone for microorganisms as compared to pure TiO2 (Wu, 2017). In biomedical applications, Ag-doped MgO emerged as a significant antimicrobial agent as compared to pure oxides of Mg against Staphylococcus aureus and P. aeruginosa (Llorens et al., 2012; Nganga et al., 2013; Meena and Swapnil, 2019). Ag- and carbon-monolith-doped NPs were also found to be more active antimicrobial agents against C. albicans, Staphylococcus aureus, and E. coli (Arakawa et al., 2019). Disk diffusion analyses have been performed against assured disease-causing pathogens like Staphylococcus aureus, E. coli, B. cereus, and P. aeruginosa to analyze their antimicrobial effects (Zhu et al., 2019). Therefore, doped metal-oxide based NPs showed more activity as antimicrobial agents as compared to pure oxides (Ewald et al., 2011).
Metal Sulfide–Based Nanoparticles
To protect the surface of the NPs, the amalgamation of semiconductor metal sulfide NPs into polymers has been performed through chemical methods (Mthethwa et al., 2011). Poly methyl methacrylate (PMMA) has been considered one of the most extensively studied polymers among a vast variety of available polymers due to having significant chemicophysical and mechanical properties (Kumar et al., 2019; Gross et al., 2007). Therefore, researches are focused on the synthesis of metal sulfides/polymer nanocomposites (ZnS/PMMA and CdS/PMMA), their characterization, and their optical properties via. direct blending to attain optically clear and thermally stable compounds with good mechanical properties (Thanh and Green, 2010; Agrawal et al., 2011; Ezhov et al., 2011; Hashmi, 2012; Prabhu and Pattabi, 2012; Ajibade and Mbese, 2014). Metal-based chalcogenides such as PbS, CdSe, CdSe-CdTe, and CdSe-ZnTe have multifarious structures (Li and Wong, 2017; Meena et al., 2016a; Meena et al., 2016b). Metallic sulfides containing chalcogenide sulfur have been analyzed and have emerged as an important toxic-free metal, earing much attention in the biomedical field (Dahoumane et al., 2016). AgS, FeS, CuS, and ZnS have been studied as the most well-known metal sulfides for biomedical applications in photothermal therapy, biosensing, drug deliveries, and biomolecular imaging (Goel et al., 2014). CuS-NPs and their derivatives have been widely used in molecule detection technology as metabolites (glucose) detectors, DNA detectors, and food-based pathogen detectors. The metal-sulphide based NPs got recognition in the field of biosensing which promotes electron transfer reactions.
Furthermore, CuS was exposed to anthropological immunoglobulin A (IgA) as a thin film–based immunosensor (Attarde and Pandit, 2020) and photothermal agents for the treatment of cancerous cells (Tian et al., 2011). Ag2S quantum dots have been used in the tracking and designing of cells in vivo, bioimaging, photodynamic treatment, and diagnostic purposes. Ag2S quantum dots can also be used as a significant active tracker for human mesenchymal stem cells (MSCs) and are also considered as antimicrobial agents (Meena et al., 2016c; Argueta-Figueroa et al., 2017). According to Ding et al. (2016), Fe3S4 showed pseudoenzyme activities to enterprise a measurable photometric enzyme and assess in human serum, which is oxidized by hydrogen peroxide through Fe3S4 NPs.
Synthesis of Nanoparticles
There are several methods that can be employed for the synthesis of NPs, which are most often divided into two main categories Bottom-up methods and Top-down methods (Wang and Xia, 2004; Meena et al., 2017a, b; Kishen et al., 2020).
In the top-down method (or destructive method) NPs are synthesized by decomposition of larger units into smaller units and these smaller units are further converted into appropriate NPs (nanometric scale particles) (Conf, 2017). This method is followed by various types of processes such as mechanical milling (Liversidge and Cundy, 1995; Merisko-Liversidge et al., 2003; Yadav et al., 2012), nanolithography, laser ablation (Hulteen et al., 1999; Amendola and Meneghetti, 2009) sputtering and thermal decomposition (Chrissafis and Bikiaris, 2011; Verma et al., 2018; Araújo et al., 2018) which have been described in Figure 2. While in bottom-up synthesis (physicochemical processes) NPs such as polymersomes (Kapakoglou et al., 2008; Christian et al., 2009), micelles (Zhu et al., 2011), liposomes and vesicles (Camelo et al., 2009) polymer conjugates (Grover and Maynard, 2010), capsules (Delcea et al., 2010; Moraes et al., 2011; Zhao et al., 2011), polymeric NPs (Grabnar and Kristl, 2011) and dendrimers (Ravoo, 2008) are synthesized by several processes like sol-gel method, green synthesis, spinning, and chemical vapour deposition (CVD) pyrolysis (Mann et al., 1997; Yarema et al., 2010; Iravani, 2011; Biswas et al., 2012; Ramesh et al., 2013; Mogilevsky et al., 2014; Liu D. et al., 2015; Needham et al., 2016; Parveen and Tremiliosi-Filho, 2016). These methods have been illustrated in Figure 3.
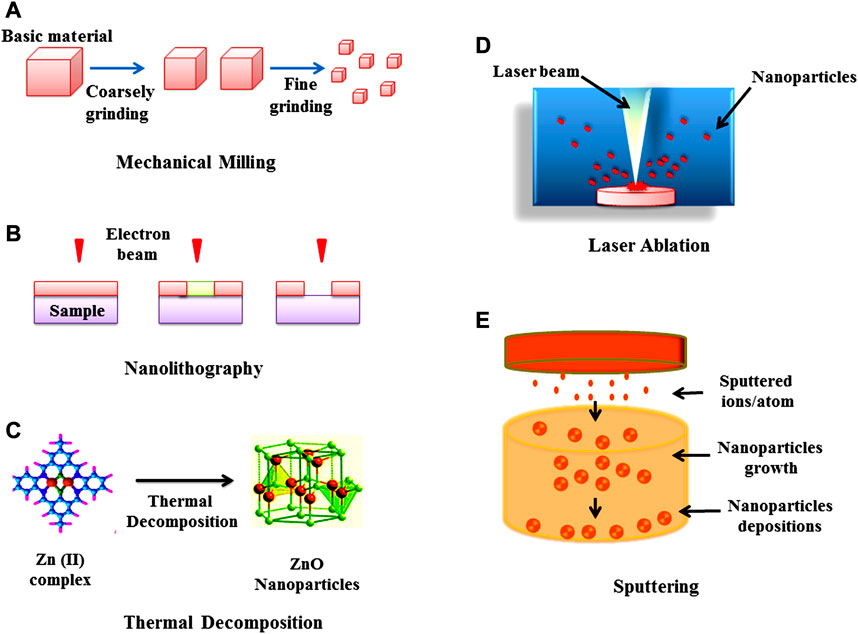
FIGURE 2. Top-down approach for the synthesis of nanoparticles (A) Mechanical milling (B) Nanolithography (C) Thermal decomposition (D) Laser ablation, and (E) Sputtering.
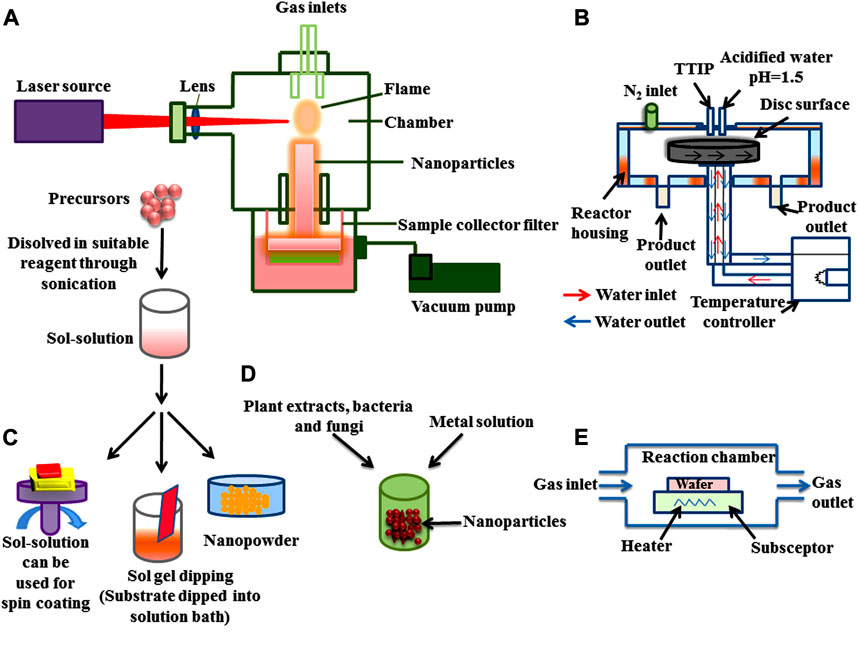
FIGURE 3. Bottom-up approach for the synthesis of nanoparticles (A) Pyrolysis (B) Spinning (C) Sol gel (D) Green synthesis, and (E) Chemical vapour deposition (CVD).
Amongst the above-mentioned methods, the green synthesis method has emerged as the most beneficial method (Iravani, 2011; Patra and Baek, 2014; Kitching et al., 2015; Park et al., 2016; Singh et al., 2016; Dahoumane et al., 2017; Singh et al., 2017). Green synthesis utilizes different metals which have been applied in different fields, such as medical (Shah et al., 2015; Al-Sheddi et al., 2018). The biological metallic NPs are synthesized by Nepeta deflersiana (Al-Sheddi et al., 2018), pink yeast, and Rhodotorula sp. ATL72 (Soliman et al., 2018) to cure various disorders in medical fields, for their antimicrobial activity, as sensors for various biomolecules, for gene delivery, and for labeling of cells in medicine and plants (Wang et al., 2006; Khandel et al., 2018).
Mechanisms of Microorganism Based Nanoparticle Biosynthesis
To reduce the metal ions into NPs, secondary metabolites secretion and intra and extra microbial enzyme (cellulary) play important roles. Under metal ion stress, microorganisms secrete enzymes and biomolecules which reduce the effect of metal ions and the toxicity of metal ions are then reduced by detoxification (Singh A. et al., 2018). There are three steps which have been reported for the biosynthesis of NPs by microorganisms shown in Figures 4, 5.
Metal Ions and Microbial Interaction
Through electrostatic interaction metallic ions attach to the negatively charged surface of a microbial cell wall and are transported inside the cell through cationic membrane transport systems (Ghashghaei and Emtiazi, 2015; Singh A. et al., 2018).
Bio-Reduction of Metallic Ions
Metallic ions can be bioreduced either by functional group (hydroxyl group or carboxyl group) associated with biomolecules having reduction capabilities or by microbial enzymes (NADH-dependent nitrate reductase) which catalyze the reduction of Ag ions to Ag NPs (Talekar et al., 2012; Velusamy et al., 2016). In this reaction, a mono or di valant oxidation state is converted into a zero valent oxidation state. After reduction, zero valent state AgNPs associate to form various morphological shaped (ovale, spheres, cubes, triangles, hexagons, etc.) NPs (Chokkareddy and Redhi, 2018).
Stabilization of NPs
This step is followed to stabilize the shape of biosynthesized NPs by preventing further growth and agglomeration (Singh A. et al., 2018) by controlled and optimized physicochemical parameters such as metal salt concentration, temperature, incubation period, pH, agitation, or concentration and nature of nutrients (carbon and nitrogen) in culture media (Khandel et al., 2018). The small size and particular shape of NPs biosynthesized by endophytic microorganisms provide good quality and a higher surface/volume ration which affects the activity positively (Niño-Martínez et al., 2019).
Nanoparticles Synthetized by Endophytic Microorganisms
Green synthesis or biological methods to synthesize metal NPs are becoming more popular. Among them, endophytic microorganisms such as bacteria, fungi, and actinomycetes have the tendency to convert metal ions into metallic NPs such as Ag, Au, Zn, and Cu with the help of secondary metabolites and cellular enzymes (Joshi et al., 2017; Soliman et al., 2018). Endophytic bacteria under high metallic ion stress establish the defense mechanism to reduce the toxicity of metal ions through the precipitation of metallic ions at the nanometer scale to synthesize NPs (Iravani et al., 2014). Due to having metallic ion stress tolerance tendency, endophytic bacteria emerged as good entrant for NPs synthesis (Syed et al., 2019). Ag NPs with antibiofilm, antibacterial and antifungal activity can be synthesized from Bacillus siamensis C1, Pseudomonas poae CO (Ibrahim et al., 2019; Ibrahim et al., 2020), or Aneurinibacillus migulanus (Prathna et al., 2010), while Au NPs (5–50 nm) synthesized by Pseudomonas fluorescens 417 have bactericidal activity (Syed et al., 2016). Ag NPs synthesized by Pseudomonas aeruginosa were reported as higher active NPs. Due to having metal uptake, their accumulation and toleration capable endophytic fungi attracted more attention in research fields (Moghaddam et al., 2015). There are several advantages to endophytic fungi which make it a better microorganism for NPs’ synthesis, such as trouble-free isolation from plants or soil (Xiaowen et al., 2019), more secretion of metabolites and extracellular enzymes for the reduction of metallic ions into NPs, and it being easy to grow rapidly. Au NPs synthesized through the isolation of Fusarium solani from Chonemorpha fragrans can be used to cure cervical cancer cells (Clarance et al., 2020). ZnO NPs (size ranges from 15 to 45 nm) are synthesized by culture filtrate of the Alternaria tenuissima (Abdelhakim et al., 2020). Exserohilum rostrata has been used to synthesize Ag NPs (size ranges from 15 to 45 nm) for their antioxidant and anti-inflammatory activities (Bagur et al., 2019). Actinomycete Streptomyces are known to produce a broad range of secondary metabolites and can be utilized for the clinical use as antifungals, antibiotics, anticancer, immunosuppressives, antivirals and insecticides (Messaoudi et al., 2015; El-Gamal et al., 2018; El-Moslamy et al., 2018; Singh et al., 2019). Streptomyces capillispiralis and Streptomyces zaomyceticus Oc-5 have been used for the synthesis of Cu NPs (Hassan et al., 2018; Hassan et al., 2019). Endophytic actinomycete Isoptericola SYSU 333150 have been used to synthesized AgNPs (size ranges from 11 to 40 nm) with sunlight exposition using photo-irradiation for different time periods which show antimicrobial, cytotoxic, antioxidant and antiinflamatory effects aginst pathogens (Verma et al., 2016; Singh et al., 2017; El-Gamal et al., 2018; El-Moslamy et al., 2018; Farsi and Farokhi 2018; Abdel-Azeem et al., 2019; Xiaowen et al., 2019; Ranjani et al., 2020). Methods for the characterization of endophytic microorganisms have been illustrated in Figure 6.
Besides endophytic microorganisms, there are several plant species (Sesbania plant, Medicago sativa, Brassica juncea, and Helianthus annuus) and microorganisms (bacteria; Desulfovibrio desulfuricans NCIMB 8307, Pseudomonas stuzeri, Clostridium thermoaceticum, Klebsiella aerogens and fungi; Phanerochaete chrysoparium, Aspergillus furnigatus, Aspergillus flavus, F. oxysporum, and Verticillium sp.) that have been used for the synthesis of NPs (Ghormade et al., 2011). In the spinning methods, NPs are synthesized by spinning disc reactor (SDR) (Bhaviripudi et al., 2007; Tai et al., 2007; Mohammadi et al., 2014). The main drawback of CVD is the high-cost related equipment and its highly toxic gaseous by-products (Ealias and Saravanakumar, 2017).
Another important method is pyrolysis for the production of NPs at a large scale. Pyrolysis is a simple, resourceful, low cost, high yield, and constant process. In this method, a precursor (either liquid or vapour) burns with flame and is fed into through a small hole in the furnace at high pressure (Kammler et al., 2001), and the gaseous by-product is characterized to get NPs (Majhi et al., 2018). This green and eco-friendly method for NPs synthesis is called biosynthesis and produces nontoxic and biodegradable NPs using bacteria, plant extracts, and fungi with the precursors (Kuppusamy et al., 2014). This method produces NPs without convention chemicals (Hasan, 2015). Liposomes, vesicles, and micelles are NPs synthesized by supramolecular self-assembly of lipids and surfactants (Vaishnav and Mukherjee, 2019). Basically, micelles are the colloidal aggregates of amphiphilic molecules synthesized using soaps and detergents (Romero and Moya, 2012). Sodium dodecyl sulfate (SDS) and cetyltrimethylammonium bromide (CTAB) are the typical surfactants that form micelles (Hoque et al., 2018). Some lipids and proteins, like lipoxygenase-3, can also aggregate in micelles (26 nm) by heat induction (Brault et al., 2002).
Vesicles/liposomes/lipid vesicles are hollow spheres that are enclosed by amphiphilic molecules (Davies et al., 2006). The vesicles are classified into two types: Unilamellar vesicles (UVs) and Mutilamellar vesicles (MLVs). UVs are defined as having one amphiphile bilayer in the hollow sphere and MLVs are defined as having more than one amphiphile bilayer (Bangham et al., 1965). On the basis of compositions, vesicles are of two types; one is composed of natural or synthetic glycolipids and the other is composed of phospholipids (Romero and Moya, 2012). The properties of having a vesicle-like size and surface potential, polydispersity, degree of ionization, permeability, physical stability, and phase behaviour depend on the methodology used in preparation and the nature of the constituent (da Silva Santos et al., 2019). There are two methods that have been reported for vesicle synthesis spontaneous formation (Jung et al., 2001; Rolland et al., 2004; Segota and Težak, 2006) and vesicle fabrication (Courbin and Panizza, 2004; Segota and Težak, 2006). Spontaneous formation is applied with stress to homogenize the structure without using external energy whereas vesicle fabrication is an induced method to form vesicles via. extrusion, sonication, and other methods using external energy. Nowadays, liposomes and vesicles play a significant role in the research field for model systems and permeable biological membranes (Courbin and Panizza, 2004). Some monodispersed branched polymers, such as dendrimers, were found to be different from other linear polymer molecules which can be synthesized through divergent and convergent methods (Hodge, 1993). In divergent methods, two dormant groups and one reactive group-containing a monomer react with a first-generation dendrimer (core-forming) and then successively follows the reaction of several monomers to form large macromolecules. The main drawback of the divergent method is purified form of macromolecule synthesis. Convergent methods rely on the inward synthesis of dendrimers and are easy to purify (Hawker and Fréchet, 1990). Therefore, the development and improvement of novel technology for the synthesis of NPs with their vast applications showed their importance, particularly in the environmental systems and sustainable agricultural (Cheng et al., 2016; Shang et al., 2019; Zehra et al., 2020).
Nanomaterials as Delivery System
It has been found that NPs play a very significant role as delivery systems in agricultural research for the improvement of crops (Singh et al., 2015). The delivery process of chemicals through NPs in plants is similar to the delivery of nano drugs in humans (Jahangirian et al., 2017; Barupal et al., 2020c). In agriculture, these smart delivery systems should have time-controlled, targeted specific, well-controlled, multifunctional characteristics, and should be self-regulated to evade biological barriers. Plants and their extracts have been used to synthesize several NPs and were found to be more ecofriendly with specific well-defined size and shapes (Agarwal et al., 2017; Ahmed et al., 2017; Meena and Zehra, 2019). NPs as delivery systems have been applied in agricultural applications for the improvement of crops by studying their effect on plant growth, metabolic functions, and genetic transformation. Nano-encapsulated chemicals for agricultural purposes should be planned in a manner to show less ecotoxicity, effective concentration, high stability, solublility, time-control, and to enhance their targeted activity when certain stimuli occur (Mathur, 2016). Perez-de-Luque and Rubiales (2009) reported that nanocapsulated herbicides reduce the phytotoxicity caused by herbicides under parasitic weed control.
These nanocapsules have the ability to penetrate cuticles and release active ingredients to control target weeds. The diameter of NPs should be less than the diameter of a plant’s cell wall (5–20 nm) to penetrate and reach the plasma membrane (Schwab et al., 2016). NPs can enter into the plant cell through stomatal openings or bases of trichomes, and are then translocated to tissues (Nair et al., 2010). Lipophilic nanosilica get easily absorbed into the cuticular lipids (effective barrier made of several lipids and fatty acids) of insects through physiosorption process and destroy the protective wax layer for use as pest control in agriculture (Jampílek and Kráľová, 2017). Ag-NPs (1–5 nm) have been successfully used to control phytopathogens (Surega et al., 2019). Currently, nanotechnology applications have been employed to study biological systems in medical research and animal science.
The use of nanotechnology and their versatility can also be demonstrated in plant science research to study genomics and the function of genes for the improvement of crop species. It has been shown that silica NPs can be used to deliver drugs (Giri et al., 2005) and DNA material (Bharali et al., 2005; Meena and Samal, 2019) into animal cells and tissues but their delivery into plant cells is limited due to the presence of a cell wall. 3 nm pores containing mesoporous silica nanoparticles (MSN) can transport chemicals and DNA into plant cells due to having exclusive structural features, such as their thermally and chemically stable mesoporous structures. Mesoporous structures have well-defined surface properties with pore sizes (2–10 nm in diameter) and surface areas more than 800 m2 g−1, and are preferred as an ideal host for the various properties containing guest molecules. In most of the non-porous Au or Ag coated particle-based (such as in gene gun process) DNA or chemical delivery limitations were shown to the nucleic acid. The microinjection process can also be used for DNA delivery to the plant cell for genetic modification but they were found to be inefficient (Ahmad and Mukhtar, 2017; Meena et al., 2017g; Meena et al., 2017h). The specific feature of MSN is to prevent the leaching out of loaded molecules or drugs due to covalent bonding with the pore. The molecules are released by some chemicals (uncapping triggers), such as dithiothreitol (DTT), or disulphide-reducing antioxidant inside the cells (Torney et al., 2007). The interactions of these capped MSN systems have been studied in plant cells (without cell wall) compared with animal cells. In animal cells, endocytosis is a very efficient process as compared to plant cells due to membrane impermeability (Jat et al., 2020). Torney et al. (2007) reported that endocytotic vesicles size ranged between 0.2 and 3 mm and showed no toxicity to plants cells.
The mesoporous structure of the MSNs enables the delivery of those chemicals which are incompatible with growth media and impermeable to the membrane along with DNA material to the targeted cells. Further, developments like enlargement of pore size and more functionality of these MSNs will offer new potential and possibilities in the delivery of target specific proteins, chemicals, and nucleotides in plant biotechnology. Overall, the MSN system appeared as a new and versatile tool to study cell biology and plant endocytosis. Each plant has a specific defense mechanism to protect itself against phytopathogens and herbivorous insects. This defense mechanism is further translated into a suitable adaptive response to defend against pathogen attack (Dangl and Jones, 2001; Barupal et al., 2019). This mechanism either can be triggered or activated after a pathogen attack or be pre-existing (Koornneef and Pieterse, 2008). Under pathogen attack, plants showed resistance against pathogens which is referred to as induced systemic resistance (ISR). ISR is the alternative natural and clean biological process of an integrated pest management strategy to control plant diseases (Sticher et al., 1997; Van Loon, 1997). In cucumber plants, SiO2 NPs reduced the infection of papaya ring spot virus (PRSV) by inducing certain defense-related gene expressions to activate phenylalanine ammonia-lyase (PAL) genes peroxidases (POX). Most of the important food crops are affected by bacterial wilt which is a serious problem. Recent studies have indicated the resistance of plants to bacterial infection can be increased by treatment with biotic or abiotic stress factors. MgO-NPs showed disease resistance in tomato plants against Ralstonia solanacearum. MgO NPs significantly reduced the bacterial (R. solanacearum) infection in the root of tomato seedlings by inducing rapid synthesis of reactive oxygen species (ROS) such as oxygen-free radicals.
It has been clearly investigated that NPs induce defense-related mechanisms against pathogens through ISR. NPs such as chitosan biopolymer are biocompatible, biodegradable, and non-toxic in character and therefore can be used as delivery systems for micronutrients and immune elicitors to suppress disease in plants (Kumaraswamy et al., 2018; Meena et al., 2017c; Meena et al., 2017d). Cu and Zn CS-NPs (chitosan NPs) were synthesized by entrapping metal in chitosan. These CS-NPs were useful for controlling plant diseases like Curvularia leaf spot (CLS) of maize (Choudhary et al., 2017) and blast disease of finger millet (Sathiyabama and Manikandan, 2018) by inhibiting mycelial growth of pathogens and activating the plant growth. CLS controlled by 0.04–0.16% CS-NPs about 24.6–22.6% in the pot while in 44.0% of water condition (Choudhary et al., 2017). Cu-CS-NPs-treated plants showed 11.6% enhancement in grain weight as compared to Bavistin treated wheat plant similar to the case of Zn-CNPs. Higher concentrations of Cu-CNPs and Zn-CNPs negatively affect plant growth (Fu et al., 2020). These CS-NPs release their metals Cu2+ and Zn2+ to interact with the cellular system of plants and facilitate other metabolic processes of plants based on Cu and Zn nutrition (Rajasekaran and Santra, 2015; Saharan and Pal, 2016). Therefore, Cu-CNPs and Zn-CS-NPs improve the plant growth as well as protect from phytopathogens as plant immune elicitors by showing multimodal action. CS-NPs have emerged as better immune elicitors as compared to salicylic acid (SA) and harpin (Thakur and Sohal, 2013). The harpinPss-loaded CS-NPs (H-CS-NPs) improved the damping-off in tomato caused by a phytopathogen Rhizoctonia solani (Nadendla et al., 2018). SA functionalized chitosan nanoparticles (CS-NPs) control the Fusarium verticillioides causing post-flowering stalk rot (Kumaraswamy et al., 2019) and showed strong antifungal activity by growth inhibition of mycelia 62.2–100% at 0.08–0.16% of CS-NPs. Therefore, present studies showed strong evidence regarding how NPs act as an efficient delivery system for the continued release of bioactive compounds that trigger the plant immune system to enhance the long-lasting effect of disease suppression efficacy. NPs also work as biostimulants at a specific concentration and play a very important role in disease suppression in plants.
A Brief Discussion of Engineered Nanomaterials
For the specific physical and chemical properties, nanomaterials are engineered at 1–100 nm in particle size (Wilson et al., 2002). These engineered nanomaterials are nanoscale metals and contain oxides (e.g., iron oxides, aluminum oxides, and titanium dioxide), polymeric nanocomposite materials and polymers. Engineered nanomaterials have also been used in drug delivery, immunology, and photovoltaic cells. (Bhatia, 2016). Engineered nanomaterials play a very important role in energy generation, production of food (Morris, 2011), and remediation of water to remove toxic substances or pollutants (Hochella et al., 2019). Currently, due to having a large surface area and small size, engineered nanomaterials are discussed to improve plant growth and health with good soil quality for sustainable agriculture. These engineered nanomaterials are highly potent for soil feasibility in soils due to being highly reactive and containing distinct properties such as high cation exchange capacity, longlasting release of nutrients, and delivery of nutrients to solve the problem of soil restoration (Bastioli, 2020). Metallic oxide-based NPs such as Mn, Fe, Cu, and Ag have been widely used in biological processes (Amde et al., 2017).
In another aspect, the synthesis and use of massively engineered nanoproducts released into the environment interact with several components of the environment and are followed by dynamic transformation processes (Abbas et al., 2020). These transformations of engineered NPs are interrelated to several environmental aspects. Several environmental processes, such as physical, chemical and biological changes the mobility and availability of these engineered NPs. Physico-chemical features of engineered NPs and environmental factors (pH, temperature, ionic strength, organic, inorganic colloids, etc.) are very important conditions to transform engineered NPs (Goswami et al., 2017). Therefore, it is of high importance to study the activities of transformed engineered NPs to recognize their environmental fortune, bioavailability, and form of toxicity. Some toxicological studies revealed that some freely circulating engineered NPs can be toxic for living systems. They affect the capability and behaviour of the plants (Aslani et al., 2014).
Interaction of Nanoparticles With Plants
Nanoparticles (NPs) due to their various properties are being used in the fields of biotechnology and agriculture (Pérez-de-Luque, 2017). Different factors such as the nature of the NPs, plant physiology and interaction of the NPs govern the uptake of NPs by the plants (Khan M. R. et al., 2019; Figure 7A-C). Chemical entities, stability, and functionalization of NPs influence the uptake, translocation, and accumulation; properties are also found to be variably affected by plant type, species, and site facilitating internalization of NPs (Santana et al., 2020). Different studies have reported both the positive and negative effects of the NPs on the plants (Yang et al., 2017; Goswami et al., 2019; Kumar et al., 2019). Zinc oxide NPs showed a positive effect on soybean by increasing its root length whereas negative effects (shrunken root tip and broken root caps) were found in ryegrass (Lin and Xing, 2007; López-Moreno et al., 2010; Meena et al., 2017e; Meena et al., 2017f). Similarly, Cañas et al. (2008) also reported both positive and negative impacts of single-walled carbon nanotubes (SWCNTs) in root length of onion and cucumber, respectively.
Absorption, Uptake, and Translocation of Nanoparticles by the Plants
It is well demonstrated that the properties of NPs are the main factor in absorption by the plants (Khan I. et al., 2019; Santana et al., 2020). Among the different properties of NPs, size of the NPs is one of the main factors affecting the penetration, translocation, and accumulation of NPs to the plant cells (Lv et al., 2019). NPs with a size larger than 40–50 nm are restricted by the plant cells for absorption (Avellan et al., 2017; Pérez-de-Luque, 2017). Chemical composition, morphology, and the type of NPs are other factors affecting the uptake and translocation (Elemike et al., 2019; Sanzari et al., 2019). Additionally, Judy et al. (2012), studied another factor responsible for absorption and accumulation i.e. functionalization and coating of the nanomaterial surface. The absorption and accumulation of NPs by the plants are greatly affected by the functionalization and coating. Different researchers revealed that the physiology of plants is also an important factor influencing the uptake and translocation of NPs (Pérez-de-Luque, 2017; Khan M. R. et al., 2019). Some NPs exposed to different plant species belonging to different families showed different absorption and accumulation patterns in the plants (Balafrej et al., 2020). The effectiveness of penetration to the plant cells is greatly determined by the application method of NPs because roots and leaves are both specialized in different processes (Schwab et al., 2016).
The interactions of NPs with the environment also affect the properties of NPs, and in turn influence the uptake of NPs by the plants. Navarro et al. (2008) studied the effect of organic matter and salt ion on the absorption of NPs and found that the stability of organic matter provided better availability of the NPs to the plants whereas salt ions showed opposite results. Microbes present in the soil also influence the uptake of NPs to the plants especially mycorrhizal fungi. Mycorrhiza forms a symbiotic association with the roots of plants and hence provides a better platform for the NPs to get easily absorbed by the plants (Ingle et al., 2017; Cao et al., 2020). Once the NPs are absorbed by the plants, the translocation of the NPs is achieved in two different ways: the symplast and the apoplast (Meena et al., 2017i; Lv et al., 2019). The transport of NPs via. apoplastic pathways occur through extracellular spaces and cell walls of neighbouring cells and xylem vessels (Sanzari et al., 2019) whereas symplastic transport takes place through plasmodesmata between the two adjacent cells (Ruttkay-Nedecky et al., 2017) and sieve plates. The importance of apoplastic pathways is very crucial for the movement of the NPs within the plants (Schwab et al., 2016). NPs reach the central cylinder and vascular tissue of the roots via. this pathway and further move to the aerial parts of the plants through the xylem with the help of transpiration stream (Cifuentes et al., 2010; Banerjee et al., 2019).
Still, there is a barrier to reaching the xylem of NPs through the root via. the apoplastic pathway called the Casparian strip which can be overcome by the endodermal cells following the symplastic pathways. Different studies have reported the accumulation of some NPs at the Casparian strip (Schwab et al., 2016; Rossi et al., 2017). Translocation of NPs via. the symplastic pathway through the sieve tube elements of the phloem allows the distribution of NPs toward non-photosynthetic tissues and organs (Shukla et al., 2016; Banerjee et al., 2019). Foliar application of NPs involves the crossing of the cuticle which acts as a barrier for the NPs following the lipophilic or hydrophilic pathways (Avellan et al., 2019). Hydrophilic and lipophilic pathways involve diffusion through cuticular waxes and through the polar aqueous pores present in the cuticle and/or stomata (Fernández and Eichert, 2009; Fernández et al., 2017). In the case of foliar application, the stomatal pathway is the main route for the interaction of NPs above 10 nm. The tiny size of the cuticular pore (around 2 nm) makes it less efficient for the translocation of NPs (Eichert and Goldbach, 2008).
The information about the accumulation of NPs inside the plants mainly depends on the route of translocation (Singh J. et al., 2018). For example, if a kind of NP shows a good translocation through the xylem, application should be done to the roots, whereas if the main route of any NPs is the phloem, not xylem so they should be applied by foliar spray for the even distribution of the NPs. If the route of the translocation of the NPs is known, the accumulation of NPs in plant parts can be found. For example, if any NP is translocated through the phloem, it must be accumulated in fruits and grains. However, it is not necessary that translocation will takes place with a specific cell. Lateral movement of NPs between the xylem and phloem can also occur (Pérez-de-Luque, 2017). Translocation and accumulation of the NPs are greatly influenced by the characteristics and nature of the NPs, in addition to the physiology of the plant species (Remédios et al., 2012; Lv et al., 2019). Different studies have reported the differences in the mechanism of translocation and accumulation of the same kind of NPs for different plant species (Shang et al., 2019; Yan and Chen, 2019; Hossain et al., 2020). On the contrary, similar NPs with few differences showed different results within the same plant species (Zhang P. et al., 2019). In pea plants, faster translocation and large accumulation of carbon-coated iron NPs were found in the roots whereas slow translocation and less accumulation of the same NPs were reported in sunflower and wheat (Cifuentes et al., 2010). Further, a large amount of positively charged gold NPs were accumulated by radish and ryegrass than rice and pumpkin (Zhu et al., 2012). Negatively charged Au-NPs were not taken up faster by the roots of the plants because plant cell walls contain negative charges resulting in the accumulation of positively charged Au-NPs.
NPs generally accumulate to different plant parts, such as fruits (McClements and Xiao, 2017), grains (Mahakham et al., 2017), flowers, and young leaves (Padalia et al., 2015; Javed et al., 2019) after the translocation through the vascular system. The location of the accumulation of NPs within the plants can be crucial to avoid human and animal consumption of NPs after treatment. Different studies have demonstrated the storage of NPs in the plant parts which are not used for consumption and degradation or transformation of some NPs by the plant after some time (Kalpana and Devi Rajeswari, 2018; Khan I. et al., 2019; Salem and Fouda, 2020). Higher concentrations of NPs affects human health. Human exposure to NPs takes place via. three different routes-gastrointestinal, skin and lungs and is then distributed to the blood and brain after absorption and subsequently, to heart and kidney (Korani et al., 2015).
Interaction of Nanomaterials With Plant Cells
If the NPs are to be translocated by the symplastic pathway, they must be taken by the plant cell and cross the plasma membrane (Karny et al., 2018). There are different ways for the internalization of the NPs to take place. Nanoparticles can be taken by the plant cell through the process of endocytosis and can cross the plasma membrane (Etxeberria et al., 2016). Some NPs instead of being invaginated by the plasma membrane are taken up by the cell by the formation of pores on the plasma membrane which directly reaches the cytoplasm (Behzadi et al., 2017; Zhao and Stenzel, 2018). NPs can also bind to carrier proteins of the plasma membrane that internalize the NPs inside the plant cell (Lesniak et al., 2013). Several researchers have acknowledged aquaporins as the carrier protein for internalization of the NPs to the plant cells, however the tiny pore size creates a hinderance for NP penetration (Banerjee et al., 2019), without reorganisation and enhancement of pore size. Plasmodesmata are very important structures of plant cells for the translocation of NPs through the phloem (Fincheira et al., 2020). Additionally, ion channels are also used by the NPs for entry into the plant cells but the tiny size of the channels makes it not suitable for the NPs penetration without specific modifications (Chichiricco and Poma, 2015; Pérez-de-Luque, 2017). Endocytosis appeared to be the most suitable way for the delivery of chemicals inside specific cell organelles (Iversen et al., 2011). On the other hand, pore formation is the best way for the delivery of chemicals into the cytosol.
Molecular Approaches of Nanoparticles
Gene Carriers
It has been observed that an effortless DNA conveyance strategy would encourage investigations of plant functional genomics (Rai et al., 2015). Nonetheless, the effect of NPs on plants is limited by the plant cell wall (Torney et al., 2007). There are different relevant properties of NPs with the ability to cross biological membranes, carry out intracellular multifaceted target delivery, and perform controlled release having enabled NPs to revolutionize the genetic engineering method (Cunningham et al., 2018). However, plant cell walls act as a barrier for efficient nanocarrier delivery which is generally conquered by chemical or mechanical methods (Demirer et al., 2017). DNA and chemicals were first delivered by Torney et al. (2007) to tobacco plants through biolistic delivery of 100–200 nm gold-capped MSNs. In this method, Gold NPs were capped by the MSN pores which were loaded with the chemical expression inducer. The coating of green fluorescent protein (GFP) plasmids was done to the capped MSNs and delivered to the tobacco cotyledons by gene gun. Thereafter, unsealing and release of the chemical expression inducer caused the expression of GFP. This study demonstrated the proof role of NPs as a gene carrier into the plant cells. In addition to this, Martin-Ortigosa et al. (2014) reported the delivery of Cre recombinase proteins into the Zea mays cells using the gold plated MSNs by the biolistic method. Different strategies comprising of gene gun, electromagnetic field, and protoplast polyethylene glycol transfection are still mandatory for the efficient delivery of biomolecules into the plant cells by NPs, as NPs cannot passively bypass the plant cell wall (Cunningham et al., 2018; Lu, 2018; Rastogi et al., 2019). Even after the requirement of mechanical and chemical aid for internalization of NPs, nanocarriers still show superior performance over traditional methods because of their small size and high surface area (Shang et al., 2019). Several studies have demonstrated the successful mediated delivery of NPs to the plants in vivo (Raliya et al., 2016; Zhao et al., 2016; Lee et al., 2017) and in vitro (Pasupathy et al., 2008; Naqvi et al., 2012; Burlaka et al., 2015). Chang et al. (2013) performed fluorescence and antibody labelling techniques for the detection of gene expression in the epidermal and endodermal layer of Arabidopsis thaliana roots by using MSNs as a gene carrier to deliver foreign DNA into the plants.
Moreover, Demirer et al. (2018) studied the efficient delivery of plasmid DNA and siRNA into Eruca sativa and Nicotiana benthamiana plants using functionalized carbon nanotubes (CNT) NPs. In the leaves of E. sativa, the green fluorescent protein (GFP) was expressed whereas expressed GFP was silenced in transgenic Nicotiana benthamiana leaves. Further examinations are expected to advance NP properties and functionalization, since early outcomes are promising for additional investigation of NPs as a plant biomolecule delivery vehicle that tends to the drawbacks of the traditional strategies. This could work alongside with the appearance of nuclease-based gene-altering advancements. It is of incredible interest to researchers to improve the delivery of these progressive genome designing tools by investigating NP-based delivery techniques for assorted biomolecular cargoes.
Genetic Modification
The genetic modification of plants has been broadly investigated for the production of new varieties of crop plants with several desirable characters such as high yield, improved quality, and resistance against abiotic and biotic stress (Kumar et al., 2020). Practically, tissue culture is the main technique used in almost all of the current strategies of genetic engineering, although they are very tedious, long, and relentless procedures (Zhang et al., 2020). It is very difficult for some of the agriculturally important crop plants, such as cotton to produce transgenic plants from the tissue culture with conventional plant breeding methods. So, there should be an alternative method to overcome the constraints of traditional tissue culture methods and its associated problems. Pollen-based plant transformations are viewed as promising alternatives over traditional methods of transformation (Zhang R. et al., 2019). During pollination and fertilization, foreign DNA is directly released to the ovary by pollen grains. There is a direct production of transgenic seeds with foreign DNA transformed pollen by the process of pollination. Different physical methods such as electroporation, bombardment, sonication, and Agrobacterium infection have been used for the transformation of pollen, however its success rate is restricted. Although, this technique is promising, they are also unfavorable to pollen viability. An ideal and highly efficient method of pollen transformation is magnetofection in which a foreign DNA associated with magnetic NPs is adroitly taken up by the target cells of pollen in the presence of a magnetic field (Zhao P. et al., 2017). One of the molecular approaches of NPs is genetic modification. Pollen magnetofection is the genetic modification of pollen using NPs.
In this technique, pollens are genetically transformed with the help of magnetic NPs which are loaded with pure plasmid DNA carrying functional genes. Pure plasmid DNA is delivered into the pollen through a pollen aperture in the presence of a magnetic field. Genetically modified pollen (magnetofected pollens) produces transformed seeds through pollination (Bisen et al., 2015; Zhang R. et al., 2019). One of the main advantages of this technique is that foreign DNA can stably express in successive generations. Pollen magnetofection is an effective stage for genetic modification of cotton and other crops with high-throughput and proficient potential infield activity (Altindal and Altindal, 2020). The wall of pollen is reduced at the surface apertures with a diameter of about 5–10 μm in most of the crop pollens. Zhao P. et al. (2017) reported the presence of such aperture in cotton pollen where the wall of pollen was thin with high permeability. The thin pollen wall made the delivery of foreign DNA possible inside the pollen. MNPs were used by Zhao P. et al. (2017) as DNA carriers that could easily pass through the apertures under the influence of a magnetic field. In pollen magnetofection, an MNP-DNA complex was formed by binding and condensing the negatively charged DNA with the positively charged polyethyleneimine-coated Fe3O4 MNPs which inconsequentially acts as a DNA carrier. Then, pollen was mixed with MNP–DNA complexes. Subsequent mixing of the MNP-DNA complexes were directed into the pollen through pollen aperture under the influence of the magnetic field before pollination. After the formation of the transformed seeds, transgenic plants were obtained by kanamycin screening.
RNA Interference
The RNAi pathway has risen as an amazing asset to battle plant pathogenic microbes by genetic engineering (Robinson et al., 2014; Majumdar et al., 2017). Effective use of dsRNA has developed as a profoundly engaging alternative. Up till now, nanocarriers of RNAi-inducing molecules have been used against viruses, aphids, and mosquitoes (Das et al., 2015; Mitter et al., 2017; Thairu et al., 2017). Silva et al. (2010) reported the knockdown of a target gene in tobacco protoplasts through encapsulation of siRNAs into conjugated polymer NPs. Draz et al. (2014) reviewed the use of different NPs such as metal and metal oxides NPs, silica and silicon-based NPs, carbon nanotubes, dendrimers, graphene, polymers, cyclodextrins, lipids, semiconductor nanocrystals, and hydrogels as a carrier for dsRNA. In the seedlings of Arabidopsis, fluorescent NPs loaded with dsRNA induced the gene silencing of two endogenous genes (Jiang et al., 2014). Mitter and colleagues sprayed the plants with Bioclay, a layered double hydroxide (LDH) NP loaded with dsRNA against the two viruses viz. pepper mild mottle virus (PMmoV)and cucumber mosaic virus (CMV) (Mitter et al., 2017). Further, Worrall et al. (2019), synthesized BCMVCP-BioClay by the encapsulation of BCMVCP-dsRNA (which targets the coat protein (CP) coding region of bean common mosaic virus) into LDH-NPs and reported the enhanced protection of Nicotiana benthamiana and Vigna unguiculata against aphid-mediated virus transmission as compared to the naked dsRNA. However, even though exogenous use of RNAi-inducing molecules for crop improvement still has advantages over pesticides, because of its decreased toxicity, effective use of RNAi still faces its own obstacles.
Application of Nanoparticles
Applications of Nanotechnology to Increase the Production Rate and Crop Yield
Different methods such as plant breeding, fertilizers, and plant protection products have been used for increasing the crop yield (Usman et al., 2020). The decline in agricultural productivity has been reported since the green revolution which needs another revolution in agricultural technology (Ghidan and Al Antary, 2019). Nanotechnology is a quickly emerging field with the possibility to advance forward the agriculture and food industry with new devices and tools which guarantee to increase food production in a sustainable manner and to protect crops from various diseases (Moulick et al., 2020). The management of the primary production of crops highly depends on two main fundamental aspects: increased crop production and nutrient use efficiency (Usman et al., 2020). Nanofertilizers and nanobionics both meet these two aspects and play important roles in agriculture by increasing the production rate and crop yield (Shang et al., 2019).
Nanofertilizers
The consistently growing human population is creating pressure for the agriculture sector to fulfill their continuously increasing demands (Zulfiqar et al., 2019). Chemical fertilizers that are generally used for improving crop productivity have major adverse environmental and ecological effects (Pirzadah et al., 2020). Nanotechnology which utilizes the small size of NPs (less than 100 nm) with unique properties such as higher absorption rate, utilization efficacy, and minimum losses may offer an exceptional opportunity to create a concentrated source of plant supplements (Iqbal, 2019). Nanofertilizers are being synthesized by encapsulating the plant nutrients into nanomaterials and delivering them in the form of nano-sized emulsions (Kah, 2015). The uptake and deep penetration of nanomaterials are facilitated by the nanopores and stomatal openings in plant leaves leading to higher nutrient use efficiency. Plasmodesmata which are nanosized channels between cells facilitate higher transport and delivery of nanofertilizers (Pirzadah et al., 2020). The increased efficiency of utilization causes significantly less nutrient losses of nanofertilizers which ultimately leads to higher productivity and nutritional quality of various crops.
Different approaches, such as top-down, bottom-up and biological methods (especially endophytic), are generally used for the synthesis of NPs as nanofertilizers (Shang et al., 2019; Messaoudi and Bendahou, 2020). Nanofertilizers are generally of two types, macronutrient nanofertilizers and micronutrient nanofertilizers. Different macronutrients such as nitrogen, phosphorus, potassium, magnesium, sulphur and calcium encapsulated with NPs reduce their overall requirements and deliver precise amount of nutrients to the crops (Zulfiqar et al., 2019). Nanofertilizers consist of one or more macronutrients with specific NPs. Nanofertilizers such as zeolites, hydroxyapatite and mesoporous silica NPs containing nitrogen macronutrient have been reported to show promising results by increasing the production and yield in different food crops (Fatima et al., 2020). Nanofertilizers are also synthesized by encapsulating the micronutrients to meet the requirements of different crop plants. Zinc (Zn) plays a very important role in plant growth by acting as a regulatory cofactor for various enzymes (Umair Hassan et al., 2020). Zinc has also been reported to provide protection to the plants against different pathogens (Cabot et al., 2019). Boron is also very important for the growth and development of plants as it is involved in the biosynthesis of the cell wall and its lignifications (Wimmer et al., 2019). Hence, it is crucial to apply the appropriate amount of Zn and B to different food crops for higher yield and good quality. Davarpanah et al. (2016) studied the effect of three different concentrations of nanofertilizers of Zn and B on the yield and quality of pomegranate and observed that the maximum fruit yield along with good quality was improved by the application of low amounts of B and Zn. In another study, the fruit yield and growth of shoots was increased in cucumber seedlings grown in nutrient solution containing rubber type NPs as Zn source as compared to commercial Zn sulphate fertilizer (Moghaddasi et al., 2013).
Further, Tarafdar et al. (2014) developed zinc nanofertilizers for the enhancement of crop production in pearl millet (Pennisetum glaucum L.) and found that the growth and yield of the crop were significantly enhanced by the use of zinc nanofertilizers. Several studies have reported the effect of different nanofertilizers on increased crop production in many cereals (Jyothi and Hebsur, 2017). Maghemite NPs improve crop production and stress tolerance by reducing the hydrogen peroxide content as well as lipid peroxidation in Brassica napus plants (Palmqvist et al., 2017). Fe is also a very important micronutrient for the growth and development of plants. Hu et al. (2017) studied the effect of different concentrations of iron oxide NPs and ferric ions on the physiological changes in Citrus maxima plants and demonstrated that the effect of nanofertilizers on plants was different at different concentrations. At very low concentrations there was no effect on the plants whereas at very high concentrations, plants were negatively influenced. This suggests that the effect of iron oxide NPs was concentration-dependent. Manganese (Mn) also plays an important role in various physiological processes by acting as a cofactor of various enzymes. Stabilized NPs of copper, zinc, manganese, and iron oxide NPs showed different effects on lettuce seedlings. Mn and Fe NPs enhanced plant growth whereas CuO NPs were more toxic than the Cu ions. The toxicity of ZnO NPs was similar to Zn ions (Liu et al., 2016).
Nanobionics
Plant nanobionics is a combination of plant biology and nanotechnology and it deals with the enhancement of plant productivity by improving plant growth development and photosynthetic efficiency (Sharma and Kar, 2019; Ansari et al., 2020). Nanobionics use nanomaterials for the enhancement of plant productivity (Lew et al., 2020). Photosynthetic efficiency can be improved by widening the range of solar light absorption near-infrared spectra. Nanomaterials with unique properties and higher stability can form chloroplast based photocatalytic complexes with enhanced and improved functional properties (Marchiol, 2018). Different studies have reported on the positive effects of nanomaterials on photosynthesis (Qi et al., 2013; Giraldo et al., 2014). The high photocatalytic activity of titanium oxide nanoparticles (nTiO2) play a role in the enhancement of absorption of light by the leaves and increase photosynthesis. nTiO2 enhances the photosynthetic rate by influencing the electron chain transport, photophosphorylation activity, Rubisco carboxylation, and protection of chloroplast from ageing (Linglan et al., 2008; Qi et al., 2013). It also positively influences water conductance and transpiration. Giraldo et al. (2014) studied the effect of single-walled carbon nanotubes (SWCNTs) on the photosynthesis process in leaves of Arabidopsis thaliana and isolated chloroplasts of Spinacia oleracea. The authors observed that the shelf life of isolated chloroplast and electron transport rate was highly increased in the treated leaves and chloroplast. The advantage of semiconductor SWCNTs over chloroplasts was having high electrical conductance and the ability to capture solar energy in wavelengths that were weakly absorbed by chloroplasts. Three times higher photosynthetic activity and enhanced electron transport rate were promoted by the SWCNT-chloroplast assemblies than control (Giraldo et al., 2014). From one perspective, there is no uncertainty that further comprehensive research would be expected to assess the impacts of plant nanobionics on enhanced production of sugars as well as crop yield. Then again, the upgrade of a fundamental plant function because of the consolidation of nanomaterials was shown as confirmation of the concept.
Role of Nanotechnology in Crop Protection
Antimicrobial Agents
Nanoparticles are one of the most promising agents to prevent the emergence of antimicrobial resistance against pathogenic microbes such as Fusarium oxysporum, Alternaria solani, Aspergillus niger, Ralstonia solanacearum, and Erwinia amylovora (Wang Y. et al., 2017; El-Batal et al., 2020). Acording to Chavan and Nadanathangam (2019), the use of higher concentrations of Ag and ZnO NPs (3,000 μg/ml) affect the three groups of agriculturally relevant beneficial microorganisms. The exclusive physiochemical properties of NPs and growth inhibition of pathogens make it a potential candidate for antimicrobials (Karaman et al., 2017). Different metals such as silver and copper have long been used for treatment against pathogenic microbes. It is very obvious that some of the metallic compounds have antimicrobial properties. Lately, some of the metals in the form of NPs have been used as promising antimicrobial agents. Various kinds of metallic NPs viz. aluminium, copper, gold, magnesium, silver, titanium, and zinc NPs are found to have antimicrobial properties (Sánchez-López et al., 2020; Table 1). Different NPs inhibit microbial growth through different mechanisms (Figure 8).
Antibacterial Activity of Different Nanoparticles
Silver Nanoparticles (Ag-NPs)
Different salts of silver and their derivatives are potential antimicrobial agents (Zorraquín-Peña et al., 2020). The antimicrobial properties of nanosilver particles are reported on by several researchers (Silva et al., 2017; Loo et al., 2018; Sánchez-López et al., 2020). Different mechanisms have been put forward to clarify the inhibitory impact of silver nanoparticles (Ag-NPs) on microscopic organisms (Le Ouay and Stellacci, 2015; Liao et al., 2019; Qais et al., 2019). One of the most important reasons for the antimicrobial properties of silver is high affinity towards sulphur and phosphorus. Ag-NPs react with the sulphur-containing amino acids found in the protein of bacterial cell membranes and affect the viability of bacterial cells (Roy et al., 2019). NPs react with the phosphorus moiety of the DNA and sulphur of the proteins and inhibit the DNA replication and enzymatic processes of the bacterial cell (Liao et al., 2019). Greater permeability of the cell occurs through the attachment of Ag-NPs (with a size less than 20 nm) to the sulphur-containing amino acids of the cell membrane which causes the death of the bacterial cell (Slavin et al., 2017; Guilger-Casagrande and Lima, 2019). Various studies have reported on the dose dependent-effect of Ag-NPs with the size range of 10–15 nm on the Gram-positive and Gram-negative bacteria (Pazos-Ortiz et al., 2017; Slavin et al., 2017; Chittora et al., 2020). At both high and low concentrations, silver NPs were found to inhibit the growth of bacterial cells (Wang Y. et al., 2017). In different mechanisms of inhibition of bacterial cells such as uncoupling of respiratory electron transport, blocking of respiratory chain enzymes and interference with the membrane permeability are shown by silver ions at low concentrations. Additionally, at higher concentrations, nucleic acids, and cytoplasmic contents of bacterial cells are found to be affected by silver ions (Dakal et al., 2016).
Different techniques such as TEM, SEM, and X-ray microanalyses were used to show the effect of Ag-NPs on the cell structures of Gram-positive and Gram-negative bacteria (Jung et al., 2008). Silver ion-induced almost similar morphological and physiological changes in both E. coli, and Staphylococcus aureus bacteria. But the effect of silver ion was higher in Gram-negative bacteria. It may be because of the presence of a thick layer of peptidoglycan in the Gram-positive bacteria which can prevent the inhibitory effect of silver ions up to some extent (Jung et al., 2008). The general mechanism of the death of bacterial cells by the Ag-NPs may be the interaction of silver ions to the nucleic acids. This leads to the impairment of DNA replication. Further, Smetana et al. (2008) studied the antimicrobial effect of Ag-NPs (with a size range of 2–5 nm) using green fluorescent protein (GFP)-expressing recombinant E. coli. Ag-NPs with a size of less than 10 nm causes perforation of the cell wall and by attaching to the bacterial cell, leads to death. Silver ions were also reported to induce reactive oxygen species in bacteria which leads to the destruction of the bacterial cell (Meena et al., 2013; Shaikh et al., 2019). It has been reported that the antimicrobial activity of Ag-NPs was enhanced by the combination of polymer even at low concentrations (Chen et al., 2016; Abbas et al., 2018; Batista et al., 2018). Chitosan, a cationic polysaccharide was used along with Ag-NPs to improve the antimicrobial properties of NPs (Abdelgawad et al., 2014). Cationic chitosan decreased the osmotic stability of the cell as well as leakage of intracellular constituents by binding with the negatively charged cell membranes.
The antimicrobial effect of chitosan Ag-NPs is much higher than its individual constituents i.e. chitosan and silver. Both chitosan and silver work together in chitosan Ag-NPs to destruct the bacterial cell (Regiel-Futyra et al., 2017). Chitosan attaches to the negatively charged plasma membrane of the bacteria whereas silver ions produce pores on the bacterial wall, thereby causing rapid destruction of the bacteria. Ag induced the expression of stress-related proteins such as envelope proteins and heat shock proteins on the cell membrane of the bacterial cell which has been confirmed by the proteomic approach (Zienkiewicz-Strzałka et al., 2020). Further, Tormena et al. (2020) evaluated the antimicrobial activity of Ag-NPs which were synthesized by the biological method using Handroanthus impetiginosus underbark extract.
Gold Nanoparticles (Au-NPs)
In recent decades, various investigations on the antibacterial activity of Au-NPs have been reported (Shamaila et al., 2016; Katas et al., 2019). The enhanced antimicrobial effect of Au-NPs have been demonstrated when it is used in combination with antibiotics, drugs, vaccines, and antibodies (Tao, 2018). Gu et al. (2003) reported the enhanced antibacterial effect of vancomycin antibiotic against enterococci after coating with Au-NPs. The improved efficacy of cefaclor and Au-NPs were reported against Staphylococcus aureus and E. coli when both were used together. Both cefaclor and Au-NPs show various mechanisms for inhibition of the growth of bacterial cells. Binding of Au-NPs with the DNA of the bacterial cell played an important role in the death of the bacterial cell. Cefaclor and Au-NPs both inhibited the synthesis of cell walls by creating holes which caused leakage of the contents of the bacterial cell (Rai et al., 2010). Antibacterial effects of Au-NPs were enhanced against the Gram-positive Staphylococcus epidermidis and the Gram-negative Enterobacter aerogenes when used in combination with antibiotic kanamycin. The antibacterial effect of both kanamycin and Au-NPs when used together was much higher than the individual use (Payne et al., 2016). Additionally, Rattanata et al. (2016) studied the combined effect of gallic and Au-NPs against the food borne pathogens Plesiomonas shigelloides and S. flexneri B. and demonstrated by the use of Fourier-transform infrared spectroscopy that the biomolecules of the bacterial cell were destructed by the Au-NPs–gallic acid. Further, Bagga et al. (2017) evaluated the antibacterial effect of Au-NPs along with levofloxacin antibiotic against Staphylococcus aureus, E. coli and P. aeruginosa. Analysis of the underlying mechanism revealed that the effect of gold nanoparticles-levofloxacin conjugate was much pronounced than when used alone (Bagga et al., 2017). A study conducted by Wongyai et al. (2020) on the antibacterial effect of greenly synthesized Au-NPs showed effective antibacterial activity against Staphylococcus aureus, methicillin-resistant Staphylococcus aureus, and Acinetobacter baumannii.
Magnesium Oxide Nanoparticles
MgO-NPs have great potential as an antimicrobial agent (Cai et al., 2018). MgO-NPs have been reported as a potential antimicrobial agent either used individually or in combination with other antimicrobial agents (Imani and Safaei, 2019). In one study, Cai et al. (2018) demonstrated the superior antibacterial properties of MgO-NPs against R. solanacearum at a very low concentration (250 μg/ml). Disruption of nascent biofilms and death of bacterial cell by the production of ROS, increased calcium ion concentrations and quorum sensing was reported as different antimicrobial mechanisms of the MgO-NPs against planktonic bacteria (Nguyen et al., 2018). In another study, He et al. (2016) studied the mechanism of action of MgO-NPs against some bacteria and used scanning electron microscopy technique to show the cell damage in Campylobacter jejuni, E. coli, and Salmonella enteritidis bacteria after treatment with MgO-NPs. The antibacterial effect of MgO-NPs was shown against Streptococcus mutans and Streptococcus sobrinus bacteria which was evident by the formation of a zone of inhibition using agar disk diffusion technique (Noori and Kareem, 2019). Similarly, Ibrahem et al. (2017) studied the role of MgO-NPs which were synthesized by the A. niger method as an effective antimicrobial agent against Staphylococcus aureus and P. aeruginosa. NPs synthesized by the green method proved to be effective antibacterial agents against various bacteria. MgO-NPs which were greenly synthesized by using the Dalbergia sissoo extract showed excellent antibacterial activity against E. coli and Ralstonia solanacearum (Khan et al., 2020).
Zinc Oxide Nanoparticles
ZnO-NPs are considered to be highly toxic amongst the different metallic NPs (Xie et al., 2011). Use of ZnO-NPs in agricultural and food industries is highly recommended because of selective toxicity against bacteria and negligible toxic effects on human cells (Espitia et al., 2012). Different studies have reported the antimicrobial activity of ZnO-NPs against different food-borne pathogens (Sirelkhatim et al., 2015; Khatami et al., 2018). A study conducted by Jin et al. (2009) on the antimicrobial effect of ZnO-NPs in culture media showed promising results against Listeria monocytogenes, Salmonella enteritidis, and E. coli. ZnO-NPs completely lysed some food borne pathogens such as S. typhimurium and Staphylococcus aureus and showed strong antimicrobial activity (Souza et al., 2019). Tiwari et al. (2018) reported the antibacterial mechanism of ZnO-NPs against Acinetobacter baumannii which is a multi-drug resistant pathogen. In another study, Naseer et al. (2020) used leaf extracts of Cassia fistula and Melia azedarach plants and synthesized ZnO-NPs which revealed improved antibacterial activity against E. coli and Staphylococcus aureus. Various mechanisms have been put forward to explain the antibacterial activity of the ZnO-NPs. The production of reactive oxygen species is one of the important mechanisms of the ZnO-NPs which causes lipid peroxidation and leakage of the cellular contents (Tiwari et al., 2018). ZnO-NPs also caused disruption of the cell membrane of the bacterial cell which leads to cell death (Qiu et al., 2020). Zn ions which were released from the Zn NPs were also reported to interact with the cell membrane and cellular contents of the bacterial cell (El-Nahhal et al., 2017).
Copper Oxide Nanoparticles (CuO-NPs)
The unusual crystal structure and high surface area make CuO-NPs an effective antimicrobial agent (Mahmoodi et al., 2018). Generally, the high concentrations of CuO-NPs are required for their better antibacterial activity (Concha-Guerrero et al., 2014). CuO-NPs were reported to possess antimicrobial activity against different bacterial pathogens such as E. coli, E. faecalis, S. flexneri, and S. typhimurium (Ahamed et al., 2014). In one study, Amiri et al. (2017) employed an agar diffusion test to assess the antibacterial properties of CuO-NPs against Streptococcus mutans and Lactobacilli. CuO-NPs exhibited effective results against both bacteria. CuO-NPs biosynthesized by using the leaf extracts of papaya, were found to have excellent antibacterial activity against a soil-borne pathogen R. solanacearum (Chen et al., 2019b). CuO-NPs caused damage to the cell membrane of the pathogenic bacterium and ultimately leakage of the cellular contents. It also generates toxic hydroxyl radicals which ultimately causes the death of the bacterial cell (Taran et al., 2017). CuO-NPs were biosynthesized by the actinomycetes and enhanced antimicrobial activity was reported by Nabila and Kannabiran (2018) against some bacterial pathogens. Similarly, Qamar et al. (2020) synthesized CuO-NPs from Momordica charantia plants with improved antibacterial activity against different bacterial pathogens such as Bacillus cereus, Corynebacterium xerosis, and Streptococcus viridians.
Aluminium Oxide Nanoparticles (Al2O3-NPs)
Aluminum oxide NPs show comprehensive applications as antimicrobial agents (Aderibigbe, 2017). Different studies have reported on the antimicrobial applications of Al2O3-NPs (Prashanth et al., 2015; Sánchez-López et al., 2020). Bala et al. (2011) prepared the alumina silver composite NPs and demonstrated the enhanced antimicrobial activity of NPs against E. coli and Staphylococcus epidermidis. Al2O3-NPs cause cell death by attaching to the cell surface of bacteria (Aderibigbe, 2017). In another study, Ansari et al. (2015) showed improved antibacterial activity of Al2O3-NPs against P. aeruginosa which were synthesized by biological methods using leaf extracts of lemongrass. Al2O3-NPs were synthesized by combustion methods and their effects were investigated against some Gram-positive and Gram-negative bacteria. The synthesized aluminium oxide NPs showed considerable effect against all the tested strains of bacteria (Prashanth et al., 2015). Further, Brintha and Ajitha (2016) prepared aluminium doped NPs and examined their antibacterial activity against some pathogenic bacteria. Similarly, Manyasree et al. (2018) studied the antibacterial activity of Al2O3-NPs against different bacteria such as Staphylococcus aureus, Streptococcus mutans, E. coli, and P. vulgaris. Green synthesized Al2O3-NPs showed enhanced antibacterial activity against Gram-positive and Gram-negative bacteria (Manikandan et al., 2019).
Titanium Dioxide Nanoparticles (TiO2-NPs)
Synthesis of metal oxide NPs via. chemical methods cause serious problems and are also harmful to the environment (Nayantara and Kaur, 2018). NPs synthesized by biological methods are safe, cost-effective, and environmentally friendly (Singh J. et al., 2018). Different studies have reported the synthesis of TiO2-NPs by biological methods called green synthesis. Green synthesis of TiO2-NPs has shown that the NPs synthesized by biological methods are much more effective against microbes (de Dicastillo et al., 2020). Rajakumar et al. (2012) synthesized the TiO2-NPs by using A. flavus fungus and showed enhanced antibacterial activity of TiO2-NPs against E. coli. Increased antibacterial activity of TiO2-NPs synthesized by using Aeromonas hydrophila bacterium was shown against different bacteria such as E. coli, P. aeruginosa, Staphylococcus aureus, Streptococcus pyogenes, and E. faecalis (Jayaseelan et al., 2013a). Subhapriya and Gomathipriya (2018) prepared the Trigonella foenum-graecum extract mediated TiO2-NPs with enhanced antibacterial activity against Staphylococcus aureus, K. pneumoniae, E. faecalis, Streptococcus faecalis, E. coli, P. eruginosa, P. vulgaris, B. subtilis and Yersinia enterocolitica.Bavanilatha et al. (2019) reported on the synthesis of TiO2-NPs by the root extracts of Glycyrrhiza glabra commonly known as Licorice with the help of a precursor, titanium oxysulfate. The general mechanism behind the antibacterial activity of TiO2-NPs is the generation of ROS. Generated ROS disrupts the cellular mechanisms of the bacteria and ultimately causes cell death. TiO2-NPs also interfere with the cell signaling pathways and cause changes in gene expression of the bacterial cell by affecting the transcription factors. A study conducted by Soo et al. (2020) on enhancing the antibacterial performance of TiO2-NPs reported the superior activity of titanium dioxide nanofibres coated with Ag-NPs as compared to intrinsic titanium dioxide nanofibres.
Antiviral Activities of Nanoparticles
There are several studies based on the antibacterial property of metal NPs, yet the antiviral properties of metal NPs have limited reports. Some researchers have reported on the antiviral properties of different NPs (Haggag et al., 2019; Meléndez-Villanueva et al., 2019). The diseases caused by viruses present testing issues with overall social and monetary ramifications. Synthesizing antiviral drugs that can focus on the virus and maintain host cell viability is challenging (Baram-Pinto et al., 2009). Metal NPs have been proposed as antiviral systems exploiting the core material and additionally the ligands shell (Di Gianvincenzo et al., 2010). Haggag et al. (2019) studied the antiviral properties of Ag-NPs biosynthesized by Lampranthus coccineus and Malephora lutea. Green synthesized Ag-NPs showed remarkable antiviral activity against HSV-1, HAV-10, and CoxB4 virus. Khandelwal et al. (2014) reviewed the application of Ag-NPs as potential antiviral agents for different viruses. Ag-NPs have been reported to show antiviral activity against HIV-1 viruses through inhibition of CD4 dependent virion binding as well as prevention of the post-entry phase of the HIV-1 life cycle (Lara et al., 2010). Au-NPs have also been demonstrated for their role as an antiviral agent. Au-NPs biosynthesized by using garlic extracts showed potent virucidal effects against the measles virus (Meléndez-Villanueva et al., 2019).
Antifungal Activities of Nanoparticles
Unlike the antibacterial properties of metal NPs, there are limited investigations on the antifungal activity of metal NPs. Some studies have reported on the antifungal activity of different metal NPs. Colloid Ag-NPs were reported to show antifungal activity against A. niger and Penicillium citrinum (Zhang et al., 2008). Haghighi et al. (2011) investigated the antifungal activity of TiO2/ZnO nanostructures against C. albicans and found that the TiO2/ZnO nanowires showed improved antifungal activity as compared to both individual NPs. A significant improvement in inhibition of growth of A. niger fungus was shown by the use of ZnO nanoneedles which were synthesized through the co-precipitation method (Singh J. et al., 2018). In one study, El-Nahhal et al. (2020) synthesized ZnO-NPs by the deposition onto cotton fibers and showed improved antifungal activity against Microsporum cannis. Further, Khatoon et al. (2017) evaluated the antifungal activity of Ag-NPs, synthesized by the tri-sodium citrate assisted chemical approach. Authors found that the Ag-NPs showed significant antifungal activity against Saccharomyces cerevisiae and C. albicans fungi. Antifungal activity of Ag-NPs prepared from the extract of a bacterial strain was demonstrated against C. albicans fungus (Oves et al., 2016). Jayaseelan et al. (2013b) showed improved antifungal activity of green synthesized Au-NPs by the seed extract of Abelmoschus esculentus plants against Puccinia graminis, C. albicans, A. niger, and A. flavus. The growth of two different species of Candida fungus viz. Candida tropical and C. albicans were found to be inhibited by the graphene oxide-based silver nanocomposites (Li et al., 2013). Cu-NPshave also been reported to show antifungal activity against C. albicans fungus (Usman et al., 2013).
Advantages and Challenges of Nanotechnology-Based Antimicrobial Analysis
One of the promising approaches for the smart delivery of antibacterial compounds is the use of nanocarriers (Din et al., 2017). Several studies have demonstrated the advantage of antimicrobial NPs over free antimicrobial compounds (Beyth et al., 2015; Wang Y. et al., 2017; Varier et al., 2019). Stability, solubility, and side effects are the important issues of pesticide use which are reduced by nanocarriers. Nanocarriers have enabled the use of a combination of more than one antimicrobial compound into the carrier matrix (Karaman et al., 2017). The surface alterations can be completed by focusing on ligands on the nanocarriers that are not known by the immune system and instead are explicitly focused on unique microbes. The organization of antimicrobial agents utilizing NPs can enhance the general pharmacokinetics by advancing the therapeutic index, broadening drug circulation, and maintaining controlled drug discharge. Many pathogenic bacteria develop antibacterial resistance which is prevented by the use of antibacterial NPs (Baptista et al., 2018). Bacteria finds it very difficult to develop resistance against antibacterial NPs because of the modularity in their design. Antibacterial NPs are composed of an antibacterial core material (e.g. metal or metal oxide) surrounded with an antibacterial polymeric shell or coating, in which antibiotic drugs could be loaded (Lam et al., 2016). Wu et al. (2016) reported the destruction of bacterial cell walls through a nano-piercing process after the dissolution of the polymeric shells by the core material of zinc dopped copper oxide antibacterial NPs. Differing opportunities for combination therapy along with existing antimicrobials to arrive at synergistic impacts are clear. In spite of the fact that NP-based antibacterial treatments guarantee huge advantages and advances in tending to the key obstacles in treating infectious diseases, there are difficulties in interpreting this energizing innovation for clinical use (Karthikeyan et al., 2016). These incorporate assessing the collaborations of NPs with cells, tissues, and organs, which as needs be recalibrates dosages and distinguishes legitimate organization courses to acquire therapeutic impacts. Henceforth, to give a clinical interpretation of nanomaterials, normalized in vitro experimentations that will give in vivo applicable information ought to be built up (Huh and Kwon, 2011).
Biostimulants
Biostimulants are natural or artificial substances, generally used for the improvement of the quality of the plants. They promote plant growth, increase tolerance against biotic and abiotic stresses, and enhance the yield and quality of crop plants. The need for fertilizers has also reduced because of use of biostimulants (Rouphael and Colla, 2020). NPs can also be used as biostimulants as they enhance the quality of crops. Several studies have reported on the biostimulant properties of different NPs (Byczyńska, 2017; Juárez-Maldonado et al., 2019; Kumaraswamy et al., 2019). Van et al. (2013) demonstrated the increase in the chlorophyll content, net photosynthetic rate, and nutrient uptake in coffee plants after treatment with CS-NPs. Further, Kumaraswamy et al. (2019) reported the biostimulant properties of the salicylic acid chitosan nanoparticles (SA-CS NPs) for promoting plant growth and defense mechanisms in maize. Different mechanisms such as elevation of antioxidant-defense enzyme activities, balancing of reactive oxygen species (ROS), and cell wall reinforcement by lignin deposition were used by SA-CS NPs to enhance the growth and defense system of the maize plants. Selenium nanoparticles (Se-NPs) biosynthesized by Trichoderma spp. showed growth-promoting characters in Vigna radiata plants (Keswani et al., 2014; Keswani et al., 2016; Bărbieru et al., 2019). Venkatachalam et al. (2017) studied the plant growth-promoting role of phycomolecules coated ZnO-NPs with phosphorus (P) supplementation in cotton and observed that the combination of bioengineered ZnO-NPs with P supplementation resulted into an increase in biomass, photosynthetic pigments, total soluble proteins, and antioxidant enzyme activities. Nano-silver also possesses the plant growth-promoting characteristics which can be used as a potential plant biostimulant (Byczyńska, 2017).
Pesticide Carriers
It has been estimated that almost 90% of applied pesticides are lost due to leaching, evaporation, and degradation (Lushchak et al., 2018). The loss of pesticides causes environmental pollution and increases the cost of pest management. The use of NPs as pesticide carriers have many advantages viz., enhanced bioavailability, improved specificity, ease and safety in handling, minimum ecological damage, and lower application rates (Worrall et al., 2018). Different NPs as pesticide carriers are listed in Table 2. The increased cost and toxicity of low water-soluble insecticides can be minimised by the use of NPs as carriers which can increase the solubility (Campos et al., 2018). Several studies have reported the use of NPs for the smart delivery of various insecticides (Lu et al., 2013; Zhang et al., 2013; Wang Y. et al., 2014; Campos et al., 2018). Lu et al. (2013) demonstrated the role of CS-NPs as a carrier for azadirachtin for the sustained release of insecticide. An increase in uptake and higher toxicity of thiamethoxam insecticide was reported against Helicoverpa armigera larvae when intercalated with dendrimer NPs (Liu X. et al., 2015). In another study, Nguyen et al. (2014) showed an increase in toxicity of layered double hydroxides (LDH) NPs encapsulated with anacardic acid against Spodoptera litura. Anacardic acid alone did not show higher mortality against S. litura but after loading with LDH NPs, an improvement in toxicity was observed. Evaporation of the active molecules of the pesticides after an application is a common problem associated with the loss of pesticides. Essential oils show insecticidal properties but such properties rapidly evaporate due to their chemical instability in the presence of air, light, moisture, and high temperatures. A decrease in evaporation of Artemisia arborescens L. essential oil was reported when encapsulated with solid lipid NPs (Lai et al., 2006). Further, Yang et al. (2009) reported the increase in mortality rate from 11 to 80% of essential oil of garlic intercalated with polyethylene glycol NPs against red flour beetles (Tribolium castaneum) in rice plants. In another study, α- pinene and linalool were loaded into silica NPs and applied to castor leaves and then infested with S. litura and castor semi looper. It was found that both insects showed lower feeding activity on treated castor leaves and ultimately led to death due to starvation (Rani et al., 2014). The stability of the active molecules of the insecticides is also an important concern because it decreases the use of insecticides which is essential for environmental health.
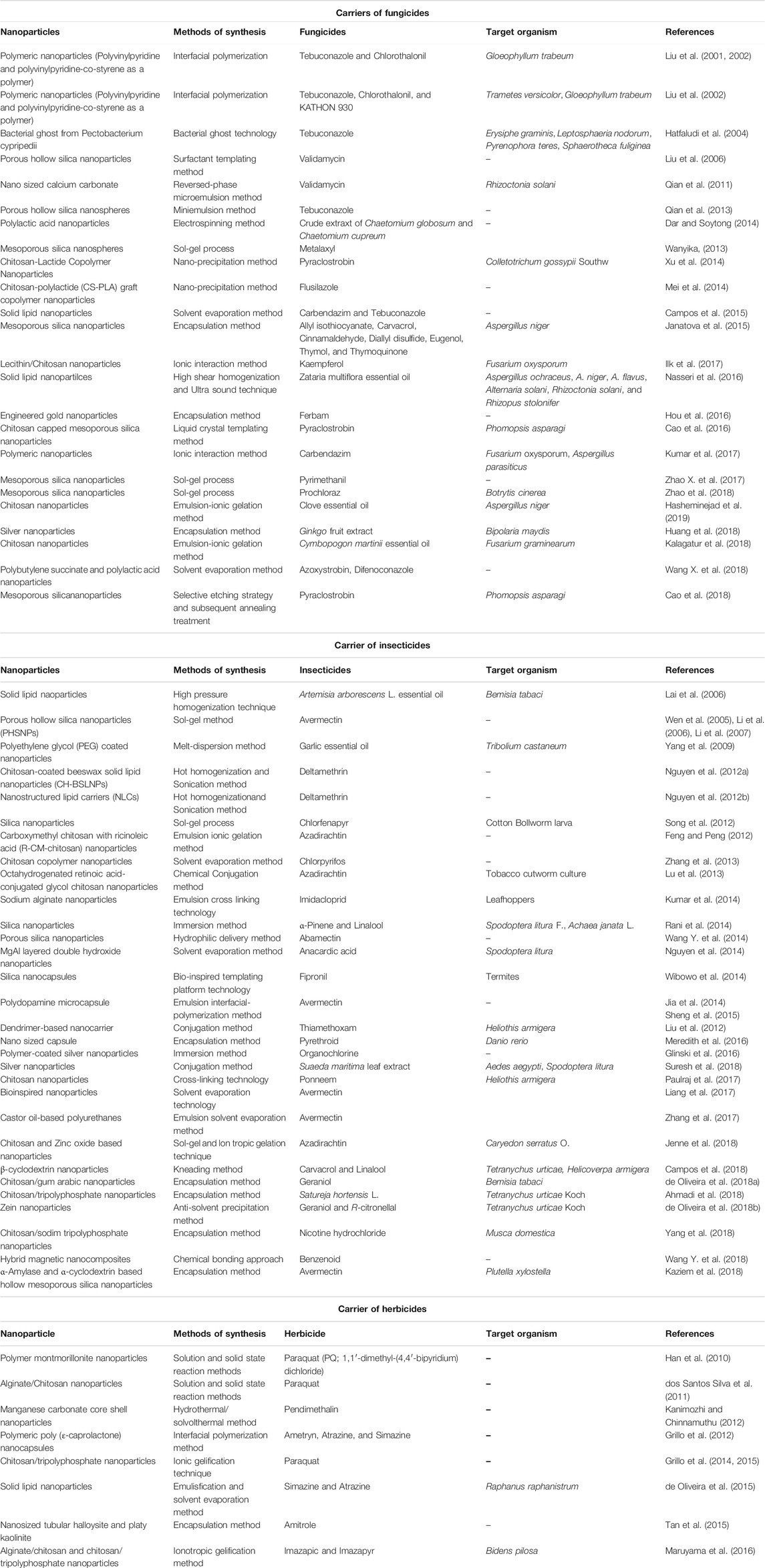
TABLE 2. Different nanoparticles as carriers of various pesticides (fungicides, insecticides and herbicides).
Several studies have reported the incorporation of different fungicides with NPs and their enhanced activity against different fungi. Various problems associated with fungicides such as low-water-solubility, volatilization, and stability were resolved by the loading of fungicides into NPs. Hatfaludi et al. (2004) reported increased toxicity by the use of fluorescence-labeled Pectobacterium cypripedii ghosts as a carrier of fungicide tebuconazole against different fungal pathogens such as Erysiphe graminis, Leptosphaeria nodorum, Pyrenophora teres, and Sphaerotheca fuliginea. An increase in inhibition against Colletotrichum gossypii Southw was also seen when pyraclostrobin was intercalated into chitosan-lactide copolymer NPs (Xu et al., 2014). Pyraclostrobin loaded NPs showed improved fungicidal activity against Colletotrichum gossypii Southw after long post-treatment which further presented controlled release properties. In another study, Ilk et al. (2017) used lecithin/CS-NPs to improve the inhibition efficacy of kaempferol fungicide against Fusarium oxysporum. Several studies have reported the improvement of the low solubility of tebuconazole and chlorothalonil fungicides by loading into various kinds of NPs. Liu et al. (2001) successfully incorporated tebuconazole and chlorothalonil into polymeric NPs and treated southern pine sapwood samples. It was found that the treated samples indicated enhanced resistance against the wood decay fungus Gloeophyllum trabeum. Further, Liu et al. (2002) created the smaller and more stable surfactant free NPs after loading with chlorothalonil and tebuconazole which ultimately increased the uptake into the wood. It also showed high inhibition activity against G. trabeum and Trametes versicolor.
Volatilization of essential oils with fungicidal activity is an important issue regarding the use of fungicides. Some essential oil components with antifungal activity, such as allyl isothiocyanate, carvacrol, cinnamaldehyde, diallyl disulfide, eugenol, thymol, and thymoquinone were encapsulated into mesoporous silica NPs. Encapsulated compounds showed enhanced activity against A. niger and also showed long-term effects by controlled release and ease of application as compared to fungicides alone (Janatova et al., 2015). Further, Nasseri et al. (2016) loaded Zataria multiflora essential oil into solid lipid NPs and showed enhanced efficacy against different fungal pathogens such as Aspergillus ochraceus, A. niger, A. flavus, Alternaria solani, R. solani, and Rhizopus stolonifer. Another major pesticide issue is the movement of water and chemicals through soil called leaching which affects the usage of pesticides. Metalaxyl fungicide encapsulated with MSN was investigated for the reduced loss and changed release profile. MSN entrapped fungicides showed controlled release behaviour as about only 11.5% of the free metalaxyl was released into the soil over a time period of 30 days as compared to the free fungicides in which 76% was released within the same time period (Wanyika, 2013). Campos et al. (2015) prepared two different types of NPs, polymeric, and solid lipid NPs loaded with a combination of tebuconazole and carbendazim and investigated their controlled release behavior and storage properties. After the loading of fungicides with NPs, their release profile and toxicity was changed. Slow controlled release, enhanced stability and fungicidal activity against R. solani were seen in validamycin loaded nanosized calcium carbonate as compared to fungicide alone (Qian et al., 2011). Further, Kumar et al. (2017) studied the bio-efficacy of polymeric NPs loaded with carbendazim against F. oxysporum and Aspergillus parasiticus and found that after incorporation of fungicide with NPs, the inhibition activity was enhanced. Moreover, Zhao X. et al. (2017) studied the uptake and distribution of the pyrimethanil loaded mesoporous silica NPs in cucumber plants and reported lower accumulation of fungicide loaded NPs in the edible parts of the plants.
Herbicides play a vital role in integrated weed management programs. The major concern of the herbicides is their non-target toxicity. Encapsulation of herbicides with NPs provides a better solution for the non-target toxicity of the herbicides. The development of NP-based herbicides has also included a wider variety of NPs. Maruyama et al. (2016) reported the improved mode of action and reduced toxicity of Imazapic and Imazapyr herbicides after loading with CS-NPs. Authors also studied the effect of herbicide loaded NPs on the soil microbiota and found no changes in the number of soil bacteria. An increase in physicochemical stability and high encapsulation efficiencies were reported in solid lipid NPs loaded with simazine and atrazine herbicides (de Oliveira et al., 2015). Herbicide loaded NPs showed enhanced toxicity and no activity against target Raphanus raphanistrum and non-target plants (Zea mays), respectively, as compared to herbicides alone. Further, de Oliveira et al. (2016) compared the effects of the clomazone herbicide in both its free form and associated with chitosan-alginate NPs. Loading of herbicides with NPs also reduces the problem of leaching.
Additionally, Chidambaram (2016) used rice husk nanosorbents encapsulated with 2, 4-dichlorophenoxyacetic acid herbicide and showed the controlled release profile, reduced leaching activity and enhanced toxicity against the target plant (Brassica sps.) as compared to herbicides alone. The release profile, stability, and storage were seen to be improved in the alginate/CS-NPs intercalated with paraquat herbicide (dos Santos Silva et al., 2011). The behaviour of herbicides in terms of chemical stability, solubility, bioavailability, photodecomposition, and soil sorption was changed by the incorporation of herbicides into the poly (ɛ-caprolactone) nanocapsules (Grillo et al., 2012). Further, Grillo et al. (2014) prepared herbicide atrazine loaded with poly (ɛ-caprolactone) nanocapsules and showed the increased physico-chemical stability and herbicide release profile. Herbicide paraquat was also encapsulated into CS-NPs by Grillo et al. (2015) and they reported the increased stability and reduced non-target toxicity of the herbicides.
Internet of Nano Things (IoNT)
It has been discussed in the above sections that nanotechnology is considered as an upfront technology to design and develop nanometers scale devices. The Internet of Things (IoT) is the intelligent interaction of different sensors and the main application of IoT has been discussed in the field of nanotechnology to offers effective solutions and opportunities in the area of pharmaceutical industries, agriculture, military and computing systems (Atlam et al., 2018). The IoNT (internet of nano things) is the replacement of sensors by nanosensors, which established a new aspect of IoT in the field of nanotechnology. Therefore, the interconnection of nanodevices and nanosensors with the internet contains a light-emitting diode referred to as IoNT (Internet of Nano Things). IoNT is introduced by Akyildiz and Jornet (Akyildiz and Jornet, 2010) where it is operated by terahertz frequencies using graphene-based nano-antennas. The dimension of this nanomachine ranges between 1 and 100 nm (Chaudhry et al., 2017; Miraz et al., 2018). According to the (UN DESA report, 2015) (UN DESA report ‘World Population Prospects: the 2015 revision), the world population is estimated to reach 9.7 billion by 2050 which will cause severe food scarcity. Fortunately, IoNT will open-up the domain in the agricultural field with more confidence to produce more adequate food supplies by enhancing crop production. IoNT reduces the harmful influences on the environment significantly (Patil et al., 2012; Nida et al., 2015; Maksimović and Omanović-Mikličanin, 2017). The use of IoNT enhances the utilization of inputs in agriculture such as water, soil, pesticides, fertilizers, etc., reduces production costs, creates high profitability, and ensures sustainability and environmental protection. Even with more future adaptability aspects, the IoNT faces several challenges due to privacy and security concerns. IoNT uses two systems of communication: Electromagnetic Nano-Communication and Molecular Communication (Lakshmi, 2018). Therefore, through the development of nanomachines, IoNT has a wonderful impact and significantly adds to revolutionizing agriculture practices to make the food industry more efficient, sustainable, and safe (Maksimović and Omanović-Mikličanin, 2017). There is a wide range of IoNT applications that have been reported. IoNT can be applied in a body sensor network (BSN) in which it plays a crucial role in the collection of data on the biological activity of patients. It also can be applied for environmental monitoring, such as temperature or air pollution. (Nayyar et al., 2017). In agriculture, it develops various exactitude agricultural practices which leads to the growth of crops and monitoring of animal, grass or pesticide and insecticide (Balasubramaniam and Kangasharju, 2013; Jarmakiewicz and Parobczak, 2016; Nayyar and Puri, 2016; Nayyar et al., 2017).
Future Perspectives
In this review, we have discussed detailed information about NPs such as their definition, types, synthesis, characterizations, properties, and applications. Several studies have shown that nanotechnology plays a very important role in commercial development. It is improving the everyday lives of human beings by increasing their performance and competence with daily objects. This technology has been used to provide a safe environment by improving air and water quality and also provides renewable energy sources for a sustainable future. We need to find more breakthroughs and novel prospects for advances in nanotechnology to develop the world economy. NPs are used in several fields such as agriculture, electronics, food, medical diagnostics, and pharmaceutical industries. This review discussed the roles of nanomaterials to show their great promise in agricultural fields. The interaction of plants with NPs results in various changes in morphological parameters, physiological parameters, and at the genotoxic level. It helps in the growth of plants through changes in their metabolic processes. It is being focused on to enhance the targeted delivery of fertilizers and pesticides and is used to minimize waste production through nano-based approaches. Currently, engineered NPs have been used widely to enhance crop production. Through nanotechnology, crop disease suppression can be explored adequately to enhance crop production. As we have discussed, apart from crop production NPs can also be used for medical diagnosis due to their surface chemistry, biocompatibility, stability, and regulating toxicity in biological systems. Therefore, nanotechnology needs to be studied intensively to analyse its long-term toxicity. The overall studies have stated that the application of NPs need more optimization for synthesis, mechanisms, and biofunctionalization of NPs. These NPs as nanobiosensors may improve plant development by detecting phytoregulators and secondary metabolites. Even with more studies, genome editing still remains an immense challenge, therefore with the help of NPs, CRISPR-Cas9 technology will provide great innovations to plant genetics. Significant research should be dedicated to this field; it will result in great benefit to plants as well as humans, and create more efficient and environmentally friendly approaches. Apart from that, most of endophytic microorganisms are unexplored and uncultivated, therefore it’s very important to focus research on developing innovative processes for the identification and isolation of the endophytic microorganisms in the green synthesis of metal NPs.
Author Contributions
MM: Provided the general concept, conceived, and drafted part of the manuscript; Writing - Original draft preparation; Prepared the figures and tables; Conceptualization; Investigation; Resources; Supervision; Validation; Visualization; Writing - Review & editing, AZ: Provided the general concept; Original draft preparation; Validation; Visualization; Prepared the figures and tables; Writing - Review & editing, PS: Provided the general concept; Original draft preparation; Conceptualization; Investigation; Validation; Visualization; Prepared the figures and tables; Writing - Review & editing, H: Writing - Original draft, review & editing; Validation; Visualization, AM: Writing - Original draft, review & editing; Validation; Visualization, GY: Writing - Original draft preparation; Investigation, PRS: Writing - Original draft preparation; Investigation, All authors read and approved it for publication.
Conflict of Interest
The authors declare that the research was conducted in the absence of any commercial or financial relationships that could be construed as a potential conflict of interest.
Acknowledgments
The author, MM is thankful to Mohanlal Sukhadia University, Udaipur for providing the necessary facilities during the course of study. The author, PS is thankful to Principal and Head, Department of Botany, Acharya Narendra Dev College, University of Delhi, New Delhi, India for providing necessary facilities during this study.
Glossary
Ag Silver
Ag2O Silver oxide
Ag-NPs Silver nanoparticles
Al Aluminium
Al2O3 Aluminium oxide
Al2O3-NPs Aluminium oxide nanoparticles
Au Gold
Au-NPs Gold nanoparticles
Bi Bismuth
Bi2O3 Bismuth sesquioxide
CaO Calcium oxide
Cd Cadmium
CLS Curvularia leaf spot
CNT Carbon nanotubes
Co Cobalt
CS-NPs Chitosan nanoparticles
CTAB Cetyl trimethylammonium bromide
Cu Copper
Cu-NPs Copper nanoparticles
CuO Copper oxide
CuO-NPs Copper oxide nanoparticles
CVD Chemical vapour deposition
DTT Dithiothreitol
Fe Iron
FeO Ferrous oxide
GFP Green fluorescent protein
GNPs β-d-Glucan nanoparticles
IgA Immunoglobulin A
IoNT Internet of nano things
ISR Induced systemic resistance
LDL Low density lipoprotein
Mg Magnesium
MnO2 Manganese dioxide
MgO Magnesium oxide
MgO-NPs Magnesium oxide nanoparticles
MLVs Mutilamellar vesicles
MSCs Mesenchymal stem cells
MSN Mesoporous silica nanoparticle
Ni Nickel
NNI National nanotechnology initiative
NPs Nanoparticles
PAL Phenylalanine ammonia lyase
Pb Lead
Pd Palladiumn
POX Peroxidases
PRSV papaya ring spot virus
Pt Platinum
ROS Reactive oxygen species
Sb Antimony
SDR Spinning disc reactor
SDS Sodium dodecyl sulphate
Ta Tantalum
Ti Titanium
TiO2 Titanium dioxide
TiO2-NPs Titanium dioxide nanoparticles
UVs Unilamellar vesicles
Zn Zinc
ZnO Zinc oxide
ZnO-NPs Zinc oxide nanoparticles
References
Abbas, M., Naeem, N., Iftikhar, H., and Latif, U. (2018). Synthesis, characterization and antimicrobial properties of silver nanocomposites. London, United Kingdom: IntechOpen. doi:10.5772/intechopen.74623
Abbas, Q., Yousaf, B., Amina, M. U., Ali, M. U., Munir, M. A. M., El-Naggar, A., et al. (2020). Transformation pathways and fate of engineered nanoparticles (ENPs) in distinct interactive environmental compartments: A review. Environ. Int. 138, 105646. doi:10.1016/j.envint.2020.105646
Abdel-Azeem, A., A. Nada, A., O’Donovan, A., Kumar Thakur, V., and Elkelish, A. (2020). Mycogenic silver nanoparticles from endophytic Trichoderma atroviride with antimicrobial activity. J. Renew. Mater. 8, 171–185. doi:10.32604/jrm.2020.08960
Abdelgawad, A. M., Hudson, S. M., and Rojas, O. J. (2014). Antimicrobial wound dressing nanofiber mats from multicomponent (chitosan/silver-NPs/polyvinyl alcohol) systems. Carbohydr. Polym. 100, 166–178. doi:10.1016/j.carbpol.2012.12.043
Abdelhakim, H. K., El‐Sayed, E. R., and Rashidi, F. B. (2020). Biosynthesis of zinc oxide nanoparticles with antimicrobial, anticancer, antioxidant and photocatalytic activities by the endophytic. Alternaria tenuissima J. Appl. Microbiol. 128, 1634–1646. doi:10.1111/jam.14581
Aderibigbe, B. (2017). Metal-based nanoparticles for the treatment of infectious diseases. Molecules 22 (8), 1370. doi:10.3390/molecules22081370
Agarwal, H., Venkat Kumar, S., and Rajeshkumar, S. (2017). A review on green synthesis of zinc oxide nanoparticles - An eco-friendly approach. Resource-Efficient Tech. 3 (4), 406–413. doi:10.1016/j.reffit.2017.03.002
Agrawal, S., Patidar, D., and Saxena, N. S. (2011). Glass transition temperature and thermal stability of ZnS/PMMA nanocomposites. Phase Transit. 84 (11–12), 888–900. doi:10.1080/01411594.2011.563152
Ahamed, M., Alhadlaq, H. A., Khan, M. A., Karuppiah, P., and Al-Dhabi, N. A. (2014). Synthesis, characterization, and antimicrobial activity of copper oxide nanoparticles. J. Nanomat. 2014, 17–21. doi:10.1155/2014/637858
Ahmad, N., and Mukhtar, Z. (2017). Genetic manipulations in crops: challenges and opportunities. Genomics 109 (5–6), 494–505. doi:10.1016/j.ygeno.2017.07.007
Ahmadi, Z., Saber, M., Akbari, A., and Mahdavinia, G. R. (2018). Encapsulation of Satureja hortensis L. (Lamiaceae) in chitosan/TPP nanoparticles with enhanced acaricide activity against Tetranychus urticae Koch (Acari: Tetranychidae). Ecotoxicology Environ. Saf. 161, 111–119. doi:10.1016/j.ecoenv.2018.05.051
Ahmed, S., Annu, S. A., Chaudhry, S. A., and Ikram, S. (2017). A review on biogenic synthesis of ZnO nanoparticles using plant extracts and microbes: a prospect towards green chemistry. J. Photochem. Photobiol. B: Biol. 166, 272–284. doi:10.1016/j.jphotobiol.2016.12.011
Ajibade, P. A., and Mbese, J. Z. (2014). Synthesis and characterization of metal sulfides nanoparticles/poly (methyl methacrylate) nanocomposites. Int. J. Polym. Sci. 2014, 752394. doi:10.1155/2014/752394
Akyildiz, I., and Jornet, J. (2010). The internet of nano-things. IEEE Wireless Commun. 17, 58–63. doi:10.1109/mwc.2010.5675779
Al-Sheddi, E. S., Farshori, N. N., Al-Oqail, M. M., Al-Massarani, S. M., Saquib, Q., Wahab, R., et al. (2018). Anticancer potential of green synthesized silver nanoparticles using extract of Nepeta deflersiana against human cervical cancer cells (HeLA). Bioinorganic Chem. Appl. 2018, 1–12. doi:10.1155/2018/9390784
Alshehri, A. A., and Malik, M. A. (2019). Biogenic fabrication of ZnO nanoparticles using Trigonella foenum-graecum (Fenugreek) for proficient photocatalytic degradation of methylene blue under UV irradiation. J. Mater. Sci. Mater. Electron. 30, 16156–16173. doi:10.1007/s10854-019-01985-8
Altindal, N., and Altindal, D. (2020). “Agriculture and nanoparticles,” in Biogenic Nano-Particles And Their Use In Agro-Ecosystems. Editors M. Ghorbanpour, P. Bhargava, A. Varma, and D. Choudhary (Singapore: Springer), 59–66. doi:10.1007/978-981-15-2985-6_4
Amde, M., Liu, J.-f., Tan, Z.-Q., and Bekana, D. (2017). Transformation and bioavailability of metal oxide nanoparticles in aquatic and terrestrial environments. A review. Environ. Pollut. 230, 250–267. doi:10.1016/j.envpol.2017.06.064
Amendola, V., and Meneghetti, M. (2009). Laser ablation synthesis in solution and size manipulation of noble metal nanoparticles. Phys. Chem. Chem. Phys. 11 (20), 3805–3821. doi:10.1039/b900654k
Amiri, M., Etemadifar, Z., Daneshkazemi, A., and Nateghi, M. (2017). Antimicrobial effect of copper oxide nanoparticles on some oral bacteria and Candida species. J. Dent. Biomater. 4 (1), 347–352.
Andrade, F. A. C., Vercik, L. C. d. O., Monteiro, F. J., and Rigo, E. C. d. S. (2016). Preparation, characterization and antibacterial properties of silver nanoparticles-hydroxyapatite composites by a simple and eco-friendly method. Ceramics Int. 42 (2), 2271–2280. doi:10.1016/j.ceramint.2015.10.021
Ansari, M. A., Khan, H. M., Alzohairy, M. A., Jalal, M., Ali, S. G., Pal, R., et al. (2015). Green synthesis of Al2O3 nanoparticles and their bactericidal potential against clinical isolates of multi-drug resistant Pseudomonas aeruginosa. World J. Microbiol. Biotechnol. 31 (1), 153–164. doi:10.1007/s11274-014-1757-2
Ansari, M. H. D., Lavhale, S., Kalunke, R. M., Srivastava, P. L., Pandit, V., Gade, S., et al. (2020). Recent advances in plant nanobionics and nanobiosensors for toxicology applications. Curr. Nanosci. 16 (1), 27–41. doi:10.2174/1573413715666190409101305
Arakawa, F. S., Shimabuku-Biadola, Q. L., Fernandes Silva, M., and Bergamasco, R. (2019). Development of a new vacuum impregnation method at room atmosphere to produce silver-copper oxide nanoparticles on activated carbon for antibacterial applications. Environ. Technol. 41, 2400–2411. doi:10.1080/09593330.2019.1567607
Araújo, A., Mendes, M. J., Mateus, T., Costa, J., Nunes, D., Fortunato, E., et al. (2018). Ultra-fast plasmonic back reflectors production for light trapping in thin Si solar cells. J. Sol. Energy 174, 786–792. doi:10.1016/j.solener.2018.08.068
Argueta-Figueroa, L., Martínez-Alvarez, O., Santos-Cruz, J., Garcia-Contreras, R., Acosta-Torres, L. S., de la Fuente-Hernández, J., et al. (2017). Nanomaterials made of non-toxic metallic sulfides: a systematic review of their potential biomedical applications. Mater. Sci. Eng. C. 76, 1305–1315. doi:10.1016/j.msec.2017.02.120
Aslani, F., Bagheri, S., Muhd Julkapli, N., Juraimi, A. S., Hashemi, F. S., and Baghdadi, A. (2014). Effects of engineered nanomaterials on plants growth: an overview. Sci. World J. 2014, 641759. doi:10.1155/2014/641759
Atlam, H. F., Walters, R. J., and Wills, G. B. (2018). “Internet of Nano Things: Security Issues and Applications,” in Proceedings of the 2018 2nd International Conference on Cloud and Big Data Computing, New York, NY, August, 2018, 71–77. doi:10.1145/3264560.3264570
Attarde, S. S., and Pandit, S. V. (2020). Anticancer potential of nanogold conjugated toxin GNP-NN-32 from Naja naja venom. J. Venom. Anim. Toxins Incl. Trop. Dis. 26, 1–13. doi:10.1590/1678-9199-jvatitd-2019-0047
Avellan, A., Schwab, F., Masion, A., Chaurand, P., Borschneck, D., Vidal, V., et al. (2017). Nanoparticle uptake in plants: gold nanomaterial localized in roots of Arabidopsis thaliana by X-ray computed nanotomography and hyperspectral imaging. Environ. Sci. Technol. 51 (15), 8682–8691. doi:10.1021/acs.est.7b01133
Avellan, A., Yun, J., Zhang, Y., Spielman-Sun, E., Unrine, J. M., Thieme, J., et al. (2019). Nanoparticle size and coating chemistry control foliar uptake pathways, translocation, and leaf-to-rhizosphere transport in wheat. ACS Nano 13 (5), 5291–5305. doi:10.1021/acsnano.8b09781
Azam, A., Ahmed, A. S., Oves, M., Khan, M. S., Habib, S. S., and Memic, A. (2012b). Antimicrobial activity of metal oxide nanoparticles against Gram-positive and Gram-negative bacteria: a comparative study. Ijn 7, 6003–6009. doi:10.2147/IJN.S35347
Azam, A., Ahmed, A. S., Oves, M., Khan, M. S., and Memic, A. (2012a). Size-dependent antimicrobial properties of CuO nanoparticles against Gram-positive and -negative bacterial strains. Ijn 7, 3527–3535. doi:10.2147/IJN.S29020
Bagga, P., Siddiqui, H. H., Akhtar, J., Mahmood, T., Zahera, M., and Khan, M. S. (2017). Gold nanoparticles conjugated levofloxacin: for improved antibacterial activity over levofloxacin alone. Cdd 14 (8), 1114–1119. doi:10.2174/1567201814666170316113432
Bagur, H., Poojari, C. C., Melappa, G., Rangappa, R., Chandrasekhar, N., and Somu, P. (2019). Biogenically synthesized silver nanoparticles using endophyte fungal extract of Ocimum tenuiflorum and evaluation of biomedical properties. J. Clust. Sci. 31, 1241–1255. doi:10.1007/s10876-019-01731-4
Bala, T., Armstrong, G., Laffir, F., and Thornton, R. (2011). Titania-silver and alumina-silver composite nanoparticles: Novel, versatile synthesis, reaction mechanism and potential antimicrobial application. J. Colloid Interf. Sci. 356 (2), 395–403. doi:10.1016/j.jcis.2011.01.044
Balafrej, H., Bogusz, D., Triqui, Z.-E. A., Guedira, A., Bendaou, N., Smouni, A., et al. (2020). Zinc hyperaccumulation in plants: A review. Plants 9 (5), 562. doi:10.3390/plants9050562
Balasubramaniam, S., and Kangasharju, J. (2013). Realizing the internet of nano things: challenges, solutions, and applications. Computer 46 (2), 62–68. doi:10.1109/mc.2012.389
Banerjee, K., Pramanik, P., Maity, A., Joshi, D. C., Wani, S. H., and Krishnan, P. (2019). “Methods of using nanomaterials to plant systems and their delivery to plants (mode of entry, uptake, translocation, accumulation, biotransformation and barriers,” in Advances In Phytonanotechnology: From Synthesis To Application. Editors M. Ghorbanpour, and S. H. Wani (Cambridge, MA: Academic Press), 123–152. doi:10.1016/B978-0-12-815322-2.00005-5
Bangham, A. D., Standish, M. M., and Watkins, J. C. (1965). Diffusion of univalent ions across the lamellae of swollen phospholipids. J. Mol. Biol. 13 (1), 238–252. doi:10.1016/s0022-2836(65)80093-6
Baptista, P. V., McCusker, M. P., Carvalho, A., Ferreira, D. A., Mohan, N. M., Martins, M., et al. (2018). Nano-strategies to fight multidrug resistant bacteria-"A Battle of the Titans". Front. Microbiol. 9, 1441. doi:10.3389/fmicb.2018.01441
Baram-Pinto, D., Shukla, S., Perkas, N., Gedanken, A., and Sarid, R. (2009). Inhibition of herpes simplex virus type 1 infection by silver nanoparticles capped with mercaptoethane sulfonate. Bioconjug. Chem. 20 (8), 1497–1502. doi:10.1021/bc900215b
Bareket, L., Inzelberg, L., Rand, D., David-Pur, M., Rabinovich, D., Brandes, B., et al. (2016). Temporary-tattoo for long-term high fidelity biopotential recordings. Sci. Rep. 6, 25727. doi:10.1038/srep25727
Barupal, T., Meena, M., and Sharma, K. (2020c). A study on preventive effects of Lawsonia inermis L. bioformulations against leaf spot disease of maize. Biocatal. Agric. Biotechnol. 23, 101473. doi:10.1016/j.bcab.2019.101473
Barupal, T., Meena, M., and Sharma, K. (2020b). Effect of different physical factors on Lawsonia inermis leaf extracts and their herbal formulations efficacy. Am. J. Agric. Sci. 7 (1), 1–7.
Barupal, T., Meena, M., and Sharma, K. (2020a). In vitro assay of antifungal activity of various elicitors and binders against Curvularia lunata. Food Sci. Nutr. Technol. 5 (1), 000206. doi:10.23880/fsnt-16000206
Barupal, T., Meena, M., and Sharma, K. (2019). Inhibitory effects of leaf extract of Lawsonia inermis on Curvularia lunata and characterization of novel inhibitory compounds by GC-MS analysis. Biotechnol. Rep. 23, e00335. doi:10.1016/j.btre.2019.e00335
Bastioli, C. (2020). Handbook of Biodegradable Polymers. (Berlin, Germany: Walter de Gruyter GmbH & Co KG).
Batista, C. C. S., Albuquerque, L. J. C., de Araujo, I., Albuquerque, B. L., da Silva, F. D., and Giacomelli, F. C. (2018). Antimicrobial activity of nano-sized silver colloids stabilized by nitrogen-containing polymers: the key influence of the polymer capping. RSC Adv. 8 (20), 10873–10882. doi:10.1039/c7ra13597a
Bavanilatha, M., Yoshitha, L., Nivedhitha, S., and Sahithya, S. (2019). Bioactive studies of TiO2 nanoparticles synthesized using Glycyrrhiza glabra. Biocatal. Agric. Biotechnol. 19 (8), 101131. doi:10.1016/j.bcab.2019.101131
Bayda, S., Adeel, M., Tuccinardi, T., Cordani, M., and Rizzolio, F. (2020). The History of nanoscience and nanotechnology: from chemical-physical applications to nanomedicine. Molecules 25 (1), 112. doi:10.3390/molecules25010112
Behzadi, S., Serpooshan, V., Tao, W., Hamaly, M. A., Alkawareek, M. Y., Dreaden, E. C., Brown, D., Alkilany, A. M., Farokhzad, O. C., and Mahmoudi, M. (2017). Cellular uptake of nanoparticles: journey inside the cell. Chem. Soc. Rev. 46 (14), 4218–4244. doi:10.1039/c6cs00636a
Berry, T. D., Filley, T. R., and Blanchette, R. A. (2014). Oxidative enzymatic response of white-rot fungi to single-walled carbon nanotubes. Environ. Pollut. 193, 197–204. doi:10.1016/j.envpol.2014.06.013
Beyth, N., Houri-Haddad, Y., Domb, A., Khan, W., and Hazan, R. (2015). Alternative antimicrobial approach: nano-antimicrobial materials. Evid. Based Complement. Alternat. Med. 2015, 246012. doi:10.1155/2015/246012
Bharali, D. J., Klejbor, I., Stachowiak, E. K., Dutta, P., Roy, I., Kaur, N., et al. (2005). Organically modified silica nanoparticles: a nonviral vector for in vivo gene delivery and expression in the brain. Proc. Natl. Acad. Sci. 102 (32), 11539–11544. doi:10.1073/pnas.0504926102
Bhaviripudi, S., Mile, E., Steiner, S. A., Zare, A. T., Dresselhaus, M. S., Belcher, A. M., et al. (2007). CVD Synthesis of single-walled carbon nanotubes from gold nanoparticle catalysts. J. Am. Chem. Soc. 129 (6), 1516–1517. doi:10.1021/ja0673332
Bisen, K., Keswani, C., Mishra, S., Saxena, A., Rakshit, A., and Singh, H. B. (2015). “Unrealized potential of seed biopriming for versatile agriculture,” in Nutrient Use Efficiency: From Basics To Advances. Editors A. Rakshit, H. B. Singh, and A. Sen (Chennai, TN: Springer), 193–206.
Biswas, A., Bayer, I. S., Biris, A. S., Wang, T., Dervishi, E., and Faupel, F. (2012). Advances in top–down and bottom–up surface nanofabrication: Techniques, applications & future prospects. Adv. Colloid Interf. Sci. 170 (1–2), 2–27. doi:10.1016/j.cis.2011.11.001
Brault, P.-A., Kariapper, M. S. T., Pham, C. V., Flowers, R. A., Gunning, W. T., Shah, P., et al. (2002). Protein micelles from lipoxygenase 3. Biomacromolecules 3 (4), 649–654. doi:10.1021/bm010149i
Brintha, S., and Ajitha, M. (2016). Synthesis, structural and antibacterial activity of aluminium and nickel doped ZnO nanoparticles by sol-gel method. Ajocs 1 (1), 1–9. doi:10.9734/ajocs/2016/29704
Burlaka, O. M., Pirko, Y. V., Yemets, A. I., and Blume, Y. B. (2015). Plant genetic transformation using carbon nanotubes for DNA delivery. Cytol. Genet. 49, 349–357. doi:10.3103/S009545271506002X
Byczyńska, A. (2017). Nano-silver as a potential biostimulant for plant–A review. World Sci. News 86 (3), 180–192.
Bărbieru, O. G., Dimitriu, L., Călin, M., Răut, I., Constantinescu-Aruxandei, D., and Oancea, F. (2019). Plant biostimulants based on selenium nanoparticles biosynthesized by Trichoderma strains. Multidis. Dig. Pub. Inst. Proc. 29 (1), 95. doi:10.3390/proceedings2019029095
Cabot, C., Martos, S., Llugany, M., Gallego, B., Tolrà, R., and Poschenrieder, C. (2019). A role for zinc in plant defense against pathogens and herbivores. Front. Plant Sci. 10, 1171. doi:10.3389/fpls.2019.01171
Cai, L., Chen, J., Liu, Z., Wang, H., Yang, H., and Ding, W. (2018). Magnesium oxide nanoparticles: effective agricultural antibacterial agent against Ralstonia solanacearum. Front. Microbiol. 9, 790. doi:10.3389/fmicb.2018.00790
Camelo, S., Lajavardi, L., Bochot, A., Goldenberg, B., Naud, M.-C., Brunel, N., et al. (2009). Protective effect of intravitreal injection of vasoactive intestinal peptide-loaded liposomes on experimental autoimmune uveoretinitis. J. Ocul. Pharmacol. Ther. 25 (1), 9–22. doi:10.1089/jop.2008.0074
Campos, E. V., Proença, P. L., Oliveira, J. L., Melville, C. C., Della Vechia, J. F., De Andrade, D. J., et al. (2018). Chitosan nanoparticles functionalized with β-cyclodextrin: a promising carrier for botanical pesticides. Sci. Rep. 8 (1), 2067. doi:10.1038/s41598-018-25618-y
Campos, E. V. R., Oliveira, J. L. d., Da Silva, C. M. G., Pascoli, M., Pasquoto, T., Lima, R., et al. (2015). Polymeric and solid lipid nanoparticles for sustained release of carbendazim and tebuconazole in agricultural applications. Sci. Rep. 5 (1), 13809. doi:10.1038/srep13809
Cañas, J. E., Long, M., Nations, S., Vadan, R., Dai, L., Luo, M., et al. (2008). Effects of functionalized and nonfunctionalized single-walled carbon nanotubes on root elongation of select crop species. Environ. Toxicol. Chem. 27 (9), 1922–1931. doi:10.1897/08-117.1
Cao, J., Feng, Y., Lin, X., and Wang, J. (2020). A beneficial role of arbuscular mycorrhizal fungi in influencing the effects of silver nanoparticles on plant-microbe systems in a soil matrix. Environ. Sci. Pollut. Res. 27, 11782–11796. doi:10.1007/s11356-020-07781-w
Cao, L., Zhang, H., Cao, C., Zhang, J., Li, F., and Huang, Q. (2016). Quaternized chitosan-capped mesoporous silica nanoparticles as nanocarriers for controlled pesticide release. Nanomaterials 6 (7), 126. doi:10.3390/nano6070126
Cao, L., Zhang, H., Zhou, Z., Xu, C., Shan, Y., Lin, Y., et al. (2018). Fluorophore-free luminescent double-shelled hollow mesoporous silica nanoparticles as pesticide delivery vehicles. Nanoscale 10 (43), 20354–20365. doi:10.1039/c8nr04626c
Chandran, H., Meena, M., Barupal, T., and Sharma, K. (2020b). Plant tissue culture as a perpetual source for production of industrially important bioactive compounds. Biotechnol. Rep. 26, e00450. doi:10.1016/j.btre.2020.e00450
Chandran, H., Meena, M., and Sharma, K. (2020a). Microbial biodiversity and bioremediation assessment through omics approaches. Front. Environ. Chem. 1, 570326. doi:10.3389/fenvc.2020.570326
Chang, F.-P., Kuang, L.-Y., Huang, C.-A., Jane, W.-N., Hung, Y., Hsing, Y.-I. C., et al. (2013). A simple plant gene delivery system using mesoporous silica nanoparticles as carriers. J. Mater. Chem. B. 1, 5279–5287. doi:10.1039/c3tb20529k
Chaudhry, J., Qidwai, U., Miraz, M. H., Ibrahim, A., and Valli, C. (2017). Data security among ISO/IEEE 11073 compliant personal healthcare devices through statistical fingerprinting. Proceedings of the 9th IEEE-GCC Conference and Exhibition 2017. Bahrain: Manama, 8–11 May 2017, 319–324.
Chavan, S., and Nadanathangam, V. (2019). Effects of nanoparticles on plant growth-promoting bacteria in Indian agricultural soil. Agronomy 9 (3), 140. doi:10.3390/agronomy9030140
Chawengkijwanich, C., and Hayata, Y. (2008). Development of TiO2 powder-coated food packaging film and its ability to inactivate Escherichia coli in vitro and in actual tests. Int. J. Food Microbiol. 123 (3), 288–292. doi:10.1016/j.ijfoodmicro.2007.12.017
Chen, J., Wang, X., and Han, H. (2013). A new function of graphene oxide emerges: inactivating phytopathogenic bacterium, Xanthomonas oryzae pv. Oryzae. J. Nanopart. Res. 15 (5), 1658. doi:10.1007/s11051-013-1658-6
Chen, J., Doumanidis, H., Lyons, K., Murday, J., and Roco, M. C. (2007). Manufacturing at the Nanoscale. Texas, Arlington: Report of the National Nanotechnology Initiative Workshops.
Chen, J., Li, S., Luo, J., Wang, R., and Ding, W. (2016). Enhancement of the antibacterial activity of silver nanoparticles against phytopathogenic bacterium Ralstonia solanacearum by stabilization. J. Nanomat. 2016, 7135852. doi:10.1155/2016/7135852
Chen, J., Mao, S., Xu, Z., and Ding, W. (2019a). Various antibacterial mechanisms of biosynthesized copper oxide nanoparticles against soilborne Ralstonia solanacearum. RSC Adv. 9 (7), 3788–3799. doi:10.1039/c8ra09186b
Chen, J., Meng, H., Tian, Y., Yang, R., Du, D., Li, Z., et al. (2019b). Recent advances in functionalized MnO2 nanosheets for biosensing and biomedicine applications. Nanoscale Horiz. 4, 321–338. doi:10.1039/C8NH00274F
Cheng, H. N., Klasson, K. T., Asakura, T., and Wu, Q. (2016). “Nanotechnology in agriculture,” in Nanotechnology: Delivering on the Promise. Editors H. N. Cheng, L. Doemeny, C. L. Geraci, and D. G. Schmidt (Washington, DC: ACS), 2, 233–242.
Chichiriccò, G., and Poma, A. (2015). Penetration and toxicity of nanomaterials in higher plants. Nanomaterials 5 (2), 851–873. doi:10.3390/nano5020851
Chidambaram, R. (2016). Application of rice husk nanosorbents containing 2, 4-dichlorophenoxyacetic acid herbicide to control weeds and reduce leaching from soil. J. Taiwan Inst. Chem. Eng. 63, 318–326. doi:10.1016/j.jtice.2016.03.024
Chittora, D., Meena, M., Barupal, T., Swapnil, P., and Sharma, K. (2020). Cyanobacteria as a source of biofertilizers for sustainable agriculture. Biochem. Biophys. Rep. 22, 100737. doi:10.1016/j.bbrep.2020.100737
Chokkareddy, R., and Redhi, G. G. (2018). “Green synthesis of metal nanoparticles and its reaction mechanisms,” in Green Metal Nanoparticles: Synthesis, Characterization And Their Application Editors S. Kanchi, and S. Ahmed (Beverly, MA: Scrivener Publishing LLC), 113–139. doi:10.1002/9781119418900.ch4
Choudhary, R. C., Kumaraswamy, R. V., Kumari, S., Sharma, S. S., Pal, A., Raliya, R., et al. (2017). Cu-chitosan nanoparticle boost defense responses and plant growth in maize (Zea mays L.). Sci. Rep. 7, 9754. doi:10.1038/s41598-017-08571-0
Chrissafis, K., and Bikiaris, D. (2011). Can nanoparticles really enhance thermal stability of polymers? Part I: An overview on thermal decomposition of addition polymers. Thermochim. Acta 523 (1–2), 1–24. doi:10.1016/j.tca.2011.06.010
Christian, D. A., Cai, S., Bowen, D. M., Kim, Y., Pajerowski, J. D., and Discher, D. E. (2009). Polymersome carriers: from self-assembly to siRNA and protein therapeutics. Eur. J. Pharmaceutics Biopharmaceutics 71 (3), 463–474. doi:10.1016/j.ejpb.2008.09.025
Cifuentes, Z., Custardoy, L., de la Fuente, J. M., Marquina, C., Ibarra, M. R., Rubiales, D., et al. (2010). Absorption and translocation to the aerial part of magnetic carbon-coated nanoparticles through the root of different crop plants. J. Nanobiotechnology 8 (1), 26. doi:10.1186/1477-3155-8-26
Clarance, P., Luvankar, B., Sales, J., Khusro, A., Agastian, P., Tack, J.-C., et al. (2020). Green synthesis and characterization of gold nanoparticles using endophytic fungi Fusarium solani and its in-vitro anticancer and biomedical applications. Saudi J. Biol. Sci. 27, 706–712. doi:10.1016/j.sjbs.2019.12.026
Concha-Guerrero, S. I., Brito, E. M. S., Piñón-Castillo, H. A., Tarango-Rivero, S. H., Caretta, C. A., Luna-Velasco, A., et al. (2014). Effect of CuO nanoparticles over isolated bacterial strains from agricultural soil. J. Nanomat. 2014, 148743. doi:10.1155/2014/148743
Conf, I. O. P. (2017). A review on the classification, characterisation, synthesis of nanoparticles and their application. Ser. Mater. Sci. Eng. 263, 032019. doi:10.1088/1757-899X/263/3/032019
Courbin, L., and Panizza, P. (2004). Shear-induced formation of vesicles in membrane phases: Kinetics and size selection mechanisms, elasticity versus surface tension. Phys. Rev. E. 69 (2), 21504. doi:10.1103/PhysRevE.69.021504
Cunningham, F. J., Goh, N. S., Demirer, G. S., Matos, J. L., and Landry, M. P. (2018). Nanoparticle-mediated delivery towards advancing plant genetic engineering. Trends Biotechnol. 36 (9), 882–897. doi:10.1016/j.tibtech.2018.03.009
da Silva Santos, V., Badan Ribeiro, A. P., and Andrade Santana, M. H. (2019). Solid lipid nanoparticles as carriers for lipophilic compounds for applications in foods. Food Res. Int. 122, 610–626. doi:10.1016/j.foodres.2019.01.032
Dahoumane, S. A., Wujcik, E. K., and Jeffryes, C. (2016). Noble metal, oxide and chalcogenide-based nanomaterials from scalable phototrophic culture systems. Enzyme Microb. Technology 95, 13–27. doi:10.1016/j.enzmictec.2016.06.008
Dahoumane, S., Jeffryes, C., Mechouet, M., and Agathos, S. (2017). Biosynthesis of inorganic nanoparticles: A fresh look at the control of shape, size and composition. Bioengineering 4, 14. doi:10.3390/bioengineering4010014
Dakal, T. C., Kumar, A., Majumdar, R. S., and Yadav, V. (2016). Mechanistic basis of antimicrobial actions of silver nanoparticles. Front. Microbiol. 7, 1831. doi:10.3389/fmicb.2016.01831
Dangl, J. L., and Jones, J. D. G. (2001). Plant pathogens and integrated defence responses to infection. Nature 411 (6839), 826–833. doi:10.1038/35081161
Dar, J., and Soytong, K. (2014). Construction and characterization of copolymer nanomaterials loaded with bioactive compounds from Chaetomium species. Int. J. Agric. Technol. 10 (4), 823–831.
Das, S., Debnath, N., Cui, Y., Unrine, J., and Palli, S. R. (2015). Chitosan, carbon quantum dot, and silica nanoparticle mediated dsRNA delivery for gene silencing in Aedes aegypti: a comparative analysis. ACS Appl. Mater. Inter. 7 (35), 19530–19535. doi:10.1021/acsami.5b05232
Davarpanah, S., Tehranifar, A., Davarynejad, G., Abadía, J., and Khorasani, R. (2016). Effects of foliar applications of zinc and boron nano-fertilizers on pomegranate (Punica granatum cv. Ardestani) fruit yield and quality. Sci. Hortic. 210, 57–64. doi:10.1016/j.scienta.2016.07.003
Davies, T. S., Ketner, A. M., and Raghavan, S. R. (2006). Self-assembly of surfactant vesicles that transform into viscoelastic wormlike micelles upon heating. J. Am. Chem. Soc. 128 (20), 6669–6675. doi:10.1021/ja060021e
de Dicastillo, C. L., Correa, M. G., Martínez, F. B., Streitt, C., and Galotto, M. J. (2020). “Antimicrobial effect of titanium dioxide nanoparticles,” in Titanium Dioxide. London, United Kingdom: IntechOpen. doi:10.5772/intechopen.90891
de Oliveira, C. R., Fraceto, L. F., Rizzi, G. M., Salla, R. F., Abdalla, F. C., Costa, M. J., et al. (2016). Hepatic effects of the clomazone herbicide in both its free form and associated with chitosan-alginate nanoparticles in bullfrog tadpoles. Chemosphere 149, 304–313. doi:10.1016/j.chemosphere.2016.01.076
de Oliveira, J. L., Campos, E. V. R., Gonçalves da Silva, C. M., Pasquoto, T., Lima, R., and Fraceto, L. F. (2015). Solid lipid nanoparticles co-loaded with simazine and atrazine: preparation, characterization, and evaluation of herbicidal activity. J. Agric. Food Chem. 63 (2), 422–432. doi:10.1021/jf5059045
de Oliveira, J. L., Campos, E. V. R., Pereira, A. E. S., Nunes, L. E. S., Da Silva, C. C. L., Pasquoto, T., et al. (2018a). Geraniol encapsulated in chitosan/gum arabic nanoparticles: A promising system for pest management in sustainable agriculture. J. Agric. Food Chem. 66 (21), 5325–5334. doi:10.1021/acs.jafc.8b00331
de Oliveira, J. L., Campos, E. V. R., Pereira, A. E. S., Pasquoto, T., Lima, R., Grillo, R., et al. (2018b). Zein nanoparticles as eco-friendly carrier systems for botanical repellents aiming sustainable agriculture. J. Agric. Food Chem. 66 (6), 1330–1340. doi:10.1021/acs.jafc.7b05552
Delcea, M., Yashchenok, A., Videnova, K., Kreft, O., Möhwald, H., and Skirtach, A. G. (2010). Multicompartmental micro- and nanocapsules: hierarchy and applications in biosciences. Macromol. Biosci. 10 (5), 465–474. doi:10.1002/mabi.200900359
Demirer, G. S., Chang, R., Zhang, H., Chio, L., and Landry, M. P. (2017). Nanoparticle-guided biomolecule delivery for transgene expression and gene silencing in mature plants. BioRxiv. 2017, 179549 doi:10.1101/179549
Demirer, G. S., Zhang, H., Matos, J. L., Goh, N. S., Cunningham, F. J., Sung, Y., et al. (2019). High aspect ratio nanomaterials enable delivery of functional genetic material without DNA integration in mature plants. Nat. Nanotechnol. 14 (5), 456–464. doi:10.1038/s41565-019-0382-5
Di Gianvincenzo, P., Marradi, M., Martínez-Ávila, O. M., Bedoya, L. M., Alcamí, J., and Penadés, S. (2010). Gold nanoparticles capped with sulfate-ended ligands as anti-HIV agents. Bioorg. Med. Chem. Lett. 20 (9), 2718–2721. doi:10.1016/j.bmcl.2010.03.079
Din, F. U., Aman, W., Ullah, I., Qureshi, O. S., Mustapha, O., Shafique, S., et al. (2017). Effective use of nanocarriers as drug delivery systems for the treatment of selected tumors. Ijn 12, 7291–7309. doi:10.2147/IJN.S146315
Ding, C., Yan, Y., Xiang, D., Zhang, C., and Xian, Y. (2016). Magnetic Fe3S4 nanoparticles with peroxidase-like activity, and their use in a photometric enzymatic glucose assay. Microchim. Acta 183, 625–631. doi:10.1007/s00604-015-1690-6
dos Santos Silva, M., Cocenza, D. S., Grillo, R., de Melo, N. F. S., Tonello, P. S., de Oliveira, L. C., et al. (2011). Paraquat-loaded alginate/chitosan nanoparticles: preparation, characterization and soil sorption studies. J. Hazard. Mater. 190 (1–3), 366–374. doi:10.1016/j.jhazmat.2011.03.057
Singh, D. P., Singh, H. B., and Prabha, R. (2016). Microbial inoculants in sustainable agricultural productivity (New Delhi, India: Springer), 118.
Draz, M. S., Fang, B. A., Zhang, P., Hu, Z., Gu, S., Weng, K. C., et al. (2014). Nanoparticle-mediated systemic delivery of siRNA for treatment of cancers and viral infections. Theranostics 4 (9), 872–892. doi:10.7150/thno.9404
Dreaden, E. C., Alkilany, A. M., Huang, X., Murphy, C. J., and El-Sayed, M. A. (2012). The golden age: gold nanoparticles for biomedicine. Chem. Soc. Rev. 41, 2740–2779. doi:10.1039/c1cs15237h
Ealias, A. M., and Saravanakumar, M. P. (2017). A review on the classification, characterisation, synthesis of nanoparticles and their application. IOP Conf. Ser. Mater. Sci. Eng. 263 (3), 032019. doi:10.1088/1757-899X/263/3/032019
Eichert, T., and Goldbach, H. E. (2008). Equivalent pore radii of hydrophilic foliar uptake routes in stomatous and astomatous leaf surfaces–further evidence for a stomatal pathway. Physiol. Plant 132 (4), 491–502. doi:10.1111/j.1399-3054.2007.01023.x
El-Batal, A. I., Balabel, N. M., Attia, M. S., and El-Sayyad, G. S. (2020). Antibacterial and antibiofilm potential of mono-dispersed stable copper oxide nanoparticles-streptomycin nano-drug: implications for some potato plant bacterial pathogen treatment. J. Clust. Sci. 31, 1021–1040. doi:10.1007/s10876-019-01707-4
El-Gamal, M. S., Salah, S. S., Salem, S., and Abdullah, A. (2018). Biosynthesis, characterization, and antimicrobial activity of silver nanoparticles synthesized by endophytic Streptomyces sp. Egypt. J. Biotechnol. 56, 69–85. doi:10.1007/s00449-014-1205-6
El-Moslamy, S. H. (2018). Bioprocessing strategies for cost-effective large-scale biogenic synthesis of nano-MgO from endophytic Streptomyces coelicolor strain E72 as an anti-multidrugresistant pathogens agent. Sci. Rep. 8 (1), 3820. doi:10.1038/s41598-018-22134-x
El-Nahhal, I. M., Elmanama, A. A., El Ashgar, N. M., Amara, N., Selmane, M., and Chehimi, M. M. (2017). Stabilization of nano-structured ZnO particles onto the surface of cotton fibers using different surfactants and their antimicrobial activity. Ultrason. Sonochem. 38, 478–487. doi:10.1016/j.ultsonch.2017.03.050
El-Nahhal, I. M., Salem, J., Anbar, R., Kodeh, F. S., and Elmanama, A. (2020). Preparation and antimicrobial activity of ZnO-NPs coated cotton/starch and their functionalized ZnO-Ag/cotton and Zn (II) curcumin/cotton materials. Sci. Rep. 10 (1), 5410. doi:10.1038/s41598-020-61306-6
El-Sayed, A., and Kamel, M. (2020). Advanced applications of nanotechnology in veterinary medicine. Environ. Sci. Pollut. Res. 27 (16), 19073–19086. doi:10.1007/s11356-018-3913-y
Elemike, E. E., Uzoh, I. M., Onwudiwe, D. C., and Babalola, O. O. (2019). The role of nanotechnology in the fortification of plant nutrients and improvement of crop production. Appl. Sci. 9 (3), 499. doi:10.3390/app9030499
Elmer, W., and White, J. C. (2018). The future of nanotechnology in plant pathology. Annu. Rev. Phytopathol. 56, 111–133. doi:10.1146/annurev-phyto-080417-050108
Espitia, P. J. P., Soares, N. D. F. F., dos Reis Coimbra, J. S., de Andrade, N. J., Cruz, R. S., and Medeiros, E. A. A. (2012). Zinc oxide nanoparticles: synthesis, antimicrobial activity and food packaging applications. Food Bioproc. Technol. 5 (5), 1447–1464. doi:10.1007/s11947-012-0797-6
Etxeberria, E., Gonzalez, P., Bhattacharya, P., Sharma, P., and Ke, P. C. (2016). Determining the size exclusion for nanoparticles in citrus leaves. Hortscience 51 (6), 732–737. doi:10.21273/HORTSCI.51.6.732
Ewald, A., Hösel, D., Patel, S., Grover, L. M., Barralet, J. E., and Gbureck, U. (2011). Silver-doped calcium phosphate cements with antimicrobial activity. Acta Biomater. 7, 4064–4070. doi:10.1016/j.actbio.2011.06.049
Ezhov, A. A., Shandryuk, G. A., Bondarenko, G. N., Merekalov, A. S., Abramchuk, S. S., Shatalova, A. M., et al. (2011). Liquid-crystalline polymer composites with CdS nanorods: structure and optical properties. Langmuir 27 (21), 13353–13360. doi:10.1021/la203258r
Fan, G., Dundas, C. M., Zhang, C., Lynd, N. A., and Keitz, B. K. (2018). Sequence-dependent peptide surface functionalization of metal–organic frameworks. ACS Appl. Mater. Inter. 10, 18601–18609. doi:10.1021/acsami.8b05148
Farsi, M., and Farokhi, S. (2018). Biosynthesis of antibacterial silver nanoparticles by endophytic fungus Nemania sp. isolated from Taxus baccata L. (Iranian yew). Zahedan J. Res. Med. Sci. 20 (6), e57916. doi:10.5812/zjrms.57916
Fatima, F., Hashim, A., and Anees, S. (2020). Efficacy of nanoparticles as nanofertilizer production: a review. Environ. Sci. Poll. Res. 28, 1292–1303. doi:10.1007/s11356-020-11218-9
Fellahi, O., Sarma, R. K., Das, M. R., Saikia, R., Marcon, L., Coffinier, Y., et al. (2013). The antimicrobial effect of silicon nanowires decorated with silver and copper nanoparticles. Nanotechnology 24 (49), 495101. doi:10.1088/0957-4484/24/49/495101
Feng, B. H., and Peng, L. F. (2012). Synthesis and characterization of carboxymethyl chitosan carrying ricinoleic functions as an emulsifier for azadirachtin. Carbohydr. Polym. 88 (2), 576–582. doi:10.1016/j.carbpol.2012.01.002
Fernández, V., Bahamonde, H. A., Javier Peguero-Pina, J., Gil-Pelegrín, E., Sancho-Knapik, D., Gil, L., et al. (2017). Physico-chemical properties of plant cuticles and their functional and ecological significance. J. Exp. Bot. 68 (19), 5293–5306. doi:10.1093/jxb/erx302
Fernández, V., and Eichert, T. (2009). Uptake of hydrophilic solutes through plant leaves: current state of knowledge and perspectives of foliar fertilization. Crit. Rev. Plant Sci. 28 (1–2), 36–68. doi:10.1080/07352680902743069
Filipponi, L., Sutherland, D., and Center, I. N. (2010). Introduction to nanoscience and nanotechnologies. Module 1: Fundamental concepts in nanoscience and nanotechnologies. NANOYOU Teach. Train. Kit Nanoscience Nanotechnologies 1–29.
Fincheira, P., Tortella, G., Duran, N., Seabra, A. B., and Rubilar, O. (2020). Current applications of nanotechnology to develop plant growth inducer agents as an innovation strategy. Crit. Rev. Biotechnol. 40 (1), 15–30. doi:10.1080/07388551.2019.1681931
Fu, L., Wang, Z., Dhankher, O. P., and Xing, B. (2020). Nanotechnology as a new sustainable approach for controlling crop diseases and increasing agricultural production. J. Exp. Bot. 71 (2), 507–519. doi:10.1093/jxb/erz314
Ganesh, M., Hemalatha, P., Peng, M. M., and Jang, H. T. (2017). One pot synthesized Li, Zr doped porous silica nanoparticle for low temperature CO2 adsorption. Arabian J. Chem. 10 (2), S1501–S1505. doi:10.1016/j.arabjc.2013.04.031
Gao, Q., Xie, W., Wang, Y., Wang, D., Guo, Z., Gao, F., et al. (2018). A theranostic nanocomposite system based on radial mesoporous silica hybridized with Fe3O4 nanoparticles for targeted magnetic field responsive chemotherapy of breast cancer. RSC Adv. 8, 4321–4328. doi:10.1039/C7RA12446E
Gao, X., and Lowry, G. V. (2018). Progress towards standardized and validated characterizations for measuring physicochemical properties of manufactured nanomaterials relevant to nano health and safety risks. NanoImpact 9, 14–30. doi:10.1016/j.impact.2017.09.002
Ghashghaei, S., and Emtiazi, G. (2015). The methods of nanoparticle synthesis using bacteria as biological nanofactories, their mechanisms and major applications. Curr. Bionanotechnol. 1, 3–17. doi:10.2174/2213529401999140310104655
Ghidan, A. Y., and Al Antary, T. M. (2019). “Applications of nanotechnology in agriculture,” in Applications Of Nanobiotechnology. Editors M. Stoytcheva, and R. Zlatev (London, United Kingdom: Intechopen). doi:10.5772/intechopen.88390
Ghormade, V., Deshpande, M. V., and Paknikar, K. M. (2011). Perspectives for nano-biotechnology enabled protection and nutrition of plants. Biotechnol. Adv. 29, 792–803. doi:10.1016/j.biotechadv.2011.06.007
Giraldo, J. P., Landry, M. P., Faltermeier, S. M., McNicholas, T. P., Iverson, N. M., Boghossian, A. A., et al. (2014). Plant nanobionics approach to augment photosynthesis and biochemical sensing. Nat. Mat. 13 (4), 400–408. doi:10.1038/nmat3890
Giri, S., Trewyn, B. G., Stellmaker, M. P., and Lin, V. S. Y. (2005). Stimuli‐responsive controlled‐release delivery system based on mesoporous silica nanorods capped with magnetic nanoparticles. Angew. Chem. Int. Ed. Engl. 44 (32), 5038–5044. doi:10.1002/anie.200501819
Glinski, A., Liebel, S., Pelletier, È., Voigt, C. L., Randi, M. A. F., Campos, S. X., et al. (2016). Toxicological interactions of silver nanoparticles and organochlorine pesticides in mouse peritoneal macrophages. Toxicol. Mech. Methods 26 (4), 251–259. doi:10.3109/15376516.2016.1159770
Goel, S., Chen, F., and Cai, W. (2014). Synthesis and biomedical applications of copper sulfide nanoparticles: from sensors to theranostics. Small 10, 631–645. doi:10.1002/smll.201301174
Goswami, L., Kim, K. H., Deep, A., Das, P., Bhattacharya, S. S., Kumar, S., et al. (2017). Engineered nano particles: nature, behavior, and effect on the environment. J. Enviro. Manag. 196, 297–315. doi:10.1016/j.jenvman.2017.01.011
Goswami, P., Yadav, S., and Mathur, J. (2019). Positive and negative effects of nanoparticles on plants and their applications in agriculture. Plant Sci. Today 6 (2), 232–242. doi:10.14719/pst.2019.6.2.502
Gour, A., and Jain, N. K. (2019). Advances in green synthesis of nanoparticles. Artif. Cell Nanomed. Biotechnol. 47, 844–851. doi:10.1080/21691401.2019.1577878
Grabnar, P. A., and Kristl, J. (2011). The manufacturing techniques of drug-loaded polymeric nanoparticles from preformed polymers. J. Microencapsul. 28 (4), 323–335. doi:10.3109/02652048.2011.569763
Grasso, G., Zane, D., and Dragone, R. (2020). Microbial nanotechnology: Challenges and prospects for green biocatalytic synthesis of nanoscale materials for sensoristic and biomedical applications. Nanomaterials 10, 11. doi:10.3390/nano10010011
Grillo, R., Clemente, Z., de Oliveira, J. L., Campos, E. V. R., Chalupe, V. C., Jonsson, C. M., et al. (2015). Chitosan nanoparticles loaded the herbicide paraquat: the influence of the aquatic humic substances on the colloidal stability and toxicity. J. Hazard. Mater. 286, 562–572. doi:10.1016/j.jhazmat.2014.12.021
Grillo, R., dos Santos, N. Z. P., Maruyama, C. R., Rosa, A. H., de Lima, R., and Fraceto, L. F. (2012). Poly (ɛ-caprolactone) nanocapsules as carrier systems for herbicides: Physico-chemical characterization and genotoxicity evaluation. J. Hazard. Mater. 231, 1–9. doi:10.1016/j.jhazmat.2012.06.019
Grillo, R., Rosa, A. H., and Fraceto, L. F. (2014). Poly (ε-caprolactone) nanocapsules carrying the herbicide atrazine: effect of chitosan-coating agent on physico-chemical stability and herbicide release profile. Int. J. Environ. Sci. Technol. 11 (6), 1691–1700. doi:10.1007/s13762-013-0358-1
Gross, S., Camozzo, D., Di Noto, V., Armelao, L., and Tondello, E. (2007). PMMA: A key macromolecular component for dielectric low-κ hybrid inorganic–organic polymer films. Euro. Polym. J. 43 (3), 673–696. doi:10.1016/j.eurpolymj.2006.12.012
Grover, G. N., and Maynard, H. D. (2010). Protein-polymer conjugates: synthetic approaches by controlled radical polymerizations and interesting applications. Curr. Opin. Chem. Biol. 14 (6), 818–817. doi:10.1016/j.cbpa.2010.10.008
Gu, H., Ho, P. L., Tong, E., Wang, L., and Xu, B. (2003). Presenting vancomycin on nanoparticles to enhance antimicrobial activities. Nano Lett. 3 (9), 1261–1263. doi:10.1021/nl034396z
Guilger-Casagrande, M., and Lima, R. (2019). Synthesis of silver nanoparticles mediated by fungi: A review. Front. Bioeng. Biotechnol. 22 (7), 287. doi:10.3389/fbioe.2019.00287
Gunalan, S., Sivaraj, R., and Rajendran, V. (2012). Green synthesized ZnO nanoparticles against bacterial and fungal pathogens. Prog. Nat. Sci. Mater. Int. 22 (6), 693–700. doi:10.1016/j.pnsc.2012.11.015
Guo, B. L., Han, P., Guo, L. C., Cao, Y. Q., Li, A. D., Kong, J. Z., et al. (2015). The antibacterial activity of Ta-doped ZnO nanoparticles. Nanoscale Res. Lett. 10, 1047. doi:10.1186/s11671-015-1047-4
Gurav, D. D., Jia, Y. A., Ye, J., and Qian, K. (2019). Design of plasmonic nanomaterials for diagnostic spectrometry. Nanoscale Adv. 1, 459–469. doi:10.1039/C8NA00319J
Hilton, A., Handiseni, M., Choi, W., Wang, X., Grauke, L. J., Yu, C., et al. (2017). Novel phytosanitary treatment of Xylella Fastidiosa–infected pecan scions using carbon nanotubes. San Antonio, TX: 109th Annual Meeting of the American Phytopathological Society.
Haggag, E. G., Elshamy, A. M., Rabeh, M. A., Gabr, N. M., Salem, M., Youssif, K. A., et al. (2019). Antiviral potential of green synthesized silver nanoparticles of Lampranthus coccineus and Malephora lutea. Int. J. Nanomedicine 14, 6217–6229. doi:10.2147/IJN.S214171
Haghighi, F., Roudbar Mohammadi, S., Mohammadi, P., Hosseinkhani, S., and Shipour, R. (2013). Antifungal activity of TiO2 nanoparticles and EDTA on Candida albicans biofilms. Infect. Epidemiol. Med. 1 (1), 33–38.
Haghighi, N., Abdi, Y., and Haghighi, F. (2011). Light-induced antifungal activity of TiO2 nanoparticles/ZnO nanowires. Appl. Surf. Sci. 257 (23), 10096–10100. doi:10.1016/j.apsusc.2011.06.145
Han, Y. S., Lee, S. Y., Yang, J. H., Hwang, H. S., and Park, I. (2010). Paraquat release control using intercalated montmorillonite compounds. J. Phys. Chem. Sol. 71 (4), 460–463. doi:10.1016/j.jpcs.2009.12.011
Hasan, S. (2015). A review on nanoparticles: Their synthesis and types. Res. J. Recent Sci. 4, 9–11.
Hasheminejad, N., Khodaiyan, F., and Safari, M. (2019). Improving the antifungal activity of clove essential oil encapsulated by chitosan nanoparticles. Food Chem. 275 (1), 113–122. doi:10.1016/j.foodchem.2018.09.085
Hashmi, S. A. (2012). Enhanced zinc ion transport in gel polymer electrolyte: effect of nano-sized ZnO dispersion. J. Solid State. Electrochem. 16, 3105–3114. doi:10.1007/s10008-012-1733-4
Hassan, S. E. L. D., Fouda, A., Radwan, A. A., Salem, S. S., Barghoth, M. G., Awad, M. A., et al. (2019). Endophytic actinomycetes Streptomyces spp mediated biosynthesis of copper oxide nanoparticles as a promising tool for biotechnological applications. J. Biol. Inorg. Chem. 24 (3), 377–393. doi:10.1007/s00775-019-01654-5
Hassan, S. E. L. D., Salem, S. S., Fouda, A., Awad, M. A., El-Gamal, M. S., and Abdo, A. M. (2018). New approach for antimicrobial activity and bio-control of various pathogens by biosynthesized copper nanoparticles using endophytic actinomycetes. J. Radiat. Res. Appl. Sci. 11 (3), 262–270. doi:10.1016/j.jrras.2018.05.003
Hatfaludi, T., Liska, M., Zellinger, D., Ousman, J. P., Szostak, M., Jalava, K., et al. (2004). Bacterial ghost technology for pesticide delivery. J. Agric. Food Chem. 52 (18), 5627–5634. doi:10.1021/jf049489w
Hawker, C. J., and Fréchet, J. M. J. (1990). Preparation of polymers with controlled molecular architecture. A new convergent approach to dendritic macromolecules. J. Am. Chem. Soc. 112 (21), 7638–7647. doi:10.1021/ja00177a027
Singh, H. B., Keswani, C., Reddy, M. S., Royano, E. S., and García-Estrada, C. (2019). Secondary Metabolites of Plant Growth Promoting Rhizomicroorganisms: Discovery and Applications. (Singapore: Springer-Nature), 392.
He, Y., Ingudam, S., Reed, S., Gehring, A., Strobaugh, T. P., and Irwin, P. (2016). Study on the mechanism of antibacterial action of magnesium oxide nanoparticles against foodborne pathogens. J. Nanobiotechnol. 14 (54), 1–9. doi:10.1186/s12951-016-0202-0
Heinz, H., Pramanik, C., Heinz, O., Ding, Y., Mishra, R. K., Marchon, D., et al. (2017). Nanoparticle decoration with surfactants: molecular interactions, assembly, and applications. Surf. Sci. Rep. 72 (1), 1–58. doi:10.1016/j.surfrep.2017.02.001
Hochella, M. F., Mogk, D. W., Ranville, J., Allen, I. C., Luther, G. W., Marr, L. C., et al. (2019). Natural, incidental, and engineered nanomaterials and their impacts on the Earth system. Science 363 (6434), eaau8299. doi:10.1126/science.aau8299
Hoque, M. A., Mahbub, S., Rub, M. A., Rana, S., and Khan, M. A. (2018). Experimental and theoretical investigation of micellization behavior of sodium dodecyl sulfate with cetyltrimethylammonium bromide in aqueous/urea solution at various temperatures. Korean J. Chem. Eng. 35, 2269–2282. doi:10.1007/s11814-018-0120-y
Hossain, Z., Yasmeen, F., and Komatsu, S. (2020). Nanoparticles: Synthesis, morphophysiological effects, and proteomic responses of crop plants. Int. J. Mol. Sci. 21 (9), 3056. doi:10.3390/ijms21093056
Hou, R., Zhang, Z., Pang, S., Yang, T., Clark, J. M., and He, L. (2016). Alteration of the nonsystemic behavior of the pesticide ferbam on tea leaves by engineered gold nanoparticles. Environm. Sci. Technol. 50 (12), 6216–6223. doi:10.1021/acs.est.6b01336
Hu, C., Lan, Y., Qu, J., Hu, X., and Wang, A. (2006). Ag/AgBr/TiO2 visible light photocatalyst for destruction of azodyes and bacteria. J. Phys. Chem. B. 110 (9), 4066–4072. doi:10.1021/jp0564400
Hu, J., Guo, H., Li, J., Wang, Y., Xiao, L., and Xing, B. (2017). Interaction of γ-Fe2O3 nanoparticles with Citrus maxima leaves and the corresponding physiological effects via foliar application. J. Nanobiotechnol. 15 (1), 51. doi:10.1186/s12951-017-0286-1
Hu, X., Zhang, Y., Ding, T., Liu, J., and Zhao, H. (2020). Multifunctional gold nanoparticles: A novel nanomaterial for various medical applications and biological activities. Front. Bioeng. Biotech. 8, 990. doi:10.3389/fbioe.2020.00990
Huang, W., Wang, C., Duan, H., Bi, Y., Wu, D., Du, J., et al. (2018). Synergistic antifungal effect of biosynthesized silver nanoparticles combined with fungicides. Int. J. Agric. Biol. 20 (5), 1225–1229. doi:10.17957/IJAB/15.0595
Huh, A. J., and Kwon, Y. J. (2011). “Nanoantibiotics”: a new paradigm for treating infectious diseases using nanomaterials in the antibiotics resistant era. J. Control. Release 156 (2), 128–145. doi:10.1016/j.jconrel.2011.07.002
Hulteen, J. C., Treichel, D. A., Smith, M. T., Duval, M. L., Jensen, T. R., and Van Duyne, R. P. (1999). Nanosphere lithography: size-tunable silver nanoparticle and surface cluster arrays. J. Phys. Chem. B. 103 (19), 3854–3863. doi:10.1021/jp9904771
Ibrahem, E. J., Thalij, K. M., and Badawy, A. S. (2017). Antibacterial potential of magnesium oxide nanoparticles synthesized by Aspergillus niger. Biotechnol. J. Int. 18 (1), 1–7. doi:10.9734/BJI/2017/29534
Ibrahim, E., Fouad, H., Zhang, M., Zhang, Y., Qiu, W., Yan, C., et al. (2019). Biosynthesis of silver nanoparticles using endophytic bacteria and their role in inhibition of rice pathogenic bacteria and plant growth promotion. RSC Adv. 9, 29293–29299. doi:10.1039/C9RA04246F
Ibrahim, E., Zhang, M., Zhang, Y., Hossain, A., Qiu, W., Chen, Y., et al. (2020). Green synthesization of silver nanoparticles using endophytic bacteria isolated from garlic and its antifungal activity against wheat Fusarium head blight pathogen Fusarium graminearum. Nanomaterials 10, 219. doi:10.3390/nano10020219
Ilk, S., Saglam, N., and Özgen, M. (2017). Kaempferol loaded lecithin/chitosan nanoparticles: Preparation, characterization, and their potential applications as a sustainable antifungal agent. Artif. Cell Nanomed. Biotechnol. 45 (5), 907–916. doi:10.1080/21691401.2016.1192040
Imani, M. M., and Safaei, M. (2019). Optimized synthesis of magnesium oxide nanoparticles as bactericidal agents. J. Nanotechnol. 2019, 6063832. doi:10.1155/2019/6063832
Ingle, A., Rathod, D., Varma, A., and Rai, M. (2017). “Understanding the mycorrhiza-nanoparticles interaction,” in Mycorrhiza-Eco-Physiology, Secondary Metabolites, Nanomaterials. Editors V. Ajit, P. Ram, and T. Narendra (Cham: Springer), 311–324. doi:10.1007/978-3-319-57849-1_18
Iqbal, M. A. (2019). “Nano-Fertilizers for sustainable crop production under changing climate: A global perspective,” in Sustainable Crop Production. Editors M. Fujita, and T. A. R. Nogueira (London, United Kingdom: Intechopen). doi:10.5772/intechopen.89089
Iravani, S. (2011). Green synthesis of metal nanoparticles using plants. Green. Chem. 13 (10), 2638–2650. doi:10.1039/C1GC15386B
Iravani, S., Korbekandi, H., Mirmohammadi, S. V., and Zolfaghari, B. (2014). Synthesis of silver nanoparticles: Chemical, physical and biological methods. Res. Pharm. Sci. 9 (6), 385–406.
Islam, M. T., Saenz-Arana, R., Wang, H., Bernal, R., and Noveron, J. C. (2018). Green synthesis of gold, silver, platinum, and palladium nanoparticles reduced and stabilized by sodium rhodizonate and their catalytic reduction of 4-nitrophenol and methyl orange. New J. Chem. 42 (8), 6472–6478. doi:10.1039/C8NJ01223G
Iversen, T. G., Skotland, T., and Sandvig, K. (2011). Endocytosis and intracellular transport of nanoparticles: present knowledge and need for future studies. Nano Today 6 (2), 176–185. doi:10.1016/j.nantod.2011.02.003
Jahangirian, H., Lemraski, E. G., Webster, T. J., Rafiee-Moghaddam, R., and Abdollahi, Y. (2017). A review of drug delivery systems based on nanotechnology and green chemistry: green nanomedicine. Int. J. Nanomedicine 12, 2957–2978. doi:10.2147/IJN.S127683
Jampílek, J., and Kráľová, K. (2017). “Nanopesticides: Preparation, targeting, and controlled release,” in New Pesticides And Soil Sensors. Editor A. M. Grumezescu (Cambridge, MA: Academic Press), 81–127. doi:10.1016/B978-0-12-804299-1.00004-7
Janatova, A., Bernardos, A., Smid, J., Frankova, A., Lhotka, M., Kourimská, L., et al. (2015). Long-term antifungal activity of volatile essential oil components released from mesoporous silica materials. Ind. Crop Prod. 67, 216–220. doi:10.1016/j.indcrop.2015.01.019
Jarmakiewicz, J., and Parobczak, K. (2016). “On the Internet of Nano Things in Healthcare Network,” in 2016 International Conference on Military Communications And Information Systems (ICMCIS), Brussels, Belgium, May 23–24, 2016 (IEEE), 1–6. doi:10.1109/ICMCIS.2016.7496572
Jat, S. K., Bhattacharya, J., and Sharma, M. K. (2020). Nanomaterial based gene delivery: a promising method for plant genome engineering. J. Mater. Chem. B. 8 (19), 4165–4175. doi:10.1039/D0TB00217H
Javed, Z., Dashora, K., Mishra, M., Fasake, V. D., and Srivastva, A. (2019). Effect of accumulation of nanoparticles in soil health-a concern on future. Front. Nanosci. Nanotechnol. 5, 1–9. doi:10.15761/FNN.1000181
Jayaseelan, C., Rahuman, A. A., Roopan, S. M., Kirthi, A. V., Venkatesan, J., Kim, S. K., et al. (2013a). Biological approach to synthesize TiO2 nanoparticles using Aeromonas hydrophila and its antibacterial activity. Spectrochim. Acta Part. A: Mol. Biomol. Spectro. 107, 82–89. doi:10.1016/j.saa.2012.12.083
Jayaseelan, C., Ramkumar, R., Rahuman, A. A., and Perumal, P. (2013b). Green synthesis of gold nanoparticles using seed aqueous extract of Abelmoschus esculentus and its antifungal activity. Indus. Crops Prod. 45, 423–429. doi:10.1016/j.indcrop.2012.12.019
Jenne, M., Kambham, M., Tollamadugu, N. P., Karanam, H. P., Tirupati, M. K., Balam, R. R., et al. (2018). The use of slow releasing nanoparticle encapsulated Azadirachtin formulations for the management of Caryedon serratus O. (groundnut bruchid). IET Nanobiotechnol. 12 (7), 963–967. doi:10.1049/iet-nbt.2017.0276
Jia, X., Sheng, W. B., Li, W., Tong, Y. B., Liu, Z. Y., and Zhou, F. (2014). Adhesive polydopamine coated avermectin microcapsules for prolonging foliar pesticide retention. ACS Appl. Mater. Inter. 6 (22), 19552–19558. doi:10.1021/am506458t
Jiang, L., Ding, L., He, B., Shen, J., Xu, Z., Yin, M., et al. (2014). Systemic gene silencing in plants triggered by fluorescent nanoparticle-delivered double-stranded RNA. Nanoscale 6 (17), 9965–9969. doi:10.1039/c4nr03481c
Jin, T., Sun, D., Su, J. Y., Zhang, H., and Sue, H. J. (2009). Antimicrobial efficacy of zinc oxide quantum dots against Listeria monocytogenes, Salmonella enteritidis, and Escherichia coli O157: H7. J. Food Sci. 74 (1), M46–M52. doi:10.1111/j.1750-3841.2008.01013.x
Joshi, C. G., Danagoudar, A., Poyya, J., Kudva, A. K., and Dhananjaya, B. (2017). Biogenic synthesis of gold nanoparticles by marine endophytic fungus- Cladosporium cladosporioides isolated from seaweed and evaluation of their antioxidant and antimicrobial properties. Process Biochem. 63, 137–144. doi:10.1016/j.procbio.2017.09.008
Juárez-Maldonado, A., Ortega-Ortíz, H., Morales-Díaz, A. B., González-Morales, S., Morelos-Moreno, Á., Cabrera-De la Fuente, M., et al. (2019). Nanoparticles and nanomaterials as plant biostimulants. Int. J. Mol. Sci. 20 (1), 162. doi:10.3390/ijms20010162
Judy, J. D., Unrine, J. M., Rao, W., Wirick, S., and Bertsch, P. M. (2012). Bioavailability of gold nanomaterials to plants: Importance of particle size and surface coating. Environ. Sci. Technol. 46 (15), 8467–8474. doi:10.1021/es3019397
Jung, H. T., Coldren, B., Zasadzinski, J. A., Iampietro, D. J., and Kaler, E. W. (2001). The origins of stability of spontaneous vesicles. Proc. Natl. Acad. Sci. (Pnas) USA 98 (4), 1353–1357. doi:10.1073/pnas.98.4.1353
Jung, W. K., Koo, H. C., Kim, K. W., Shin, S., Kim, S. H., and Park, Y. H. (2008). Antibacterial activity and mechanism of action of the silver ion in Staphylococcus aureus and Escherichia coli. Appl. Environ. Microbiol. 74 (7), 2171–2178. doi:10.1128/AEM.02001-07
Jyothi, T. V., and Hebsur, N. S. (2017). Effect of nanofertilizers on growth and yield of selected cereals-A review. Agric. Rev. 38 (2), 112–120. doi:10.18805/ag.v38i02.7942
Kah, M. (2015). Nanopesticides and nanofertilizers: emerging contaminants or opportunities for risk mitigation? Front. Chem. 3, 64. doi:10.3389/fchem.2015.00064
Kalagatur, N. K., Nirmal Ghosh, O. S., Sundararaj, N., and Mudili, V. (2018). Antifungal activity of chitosan nanoparticles encapsulated with Cymbopogon martinii essential oil on plant pathogenic fungi Fusarium graminearum. Front. Pharmacol. 9, 610. doi:10.3389/fphar.2018.00610
Kalpana, V. N., and Devi Rajeswari, V. (2018). A review on green synthesis, biomedical applications, and toxicity studies of ZnO NPs. Bioinorg. Chem. Appl. 2018, 3569758. doi:10.1155/2018/3569758
Kammler, B. H. K., Mädler, L., and Pratsinis, S. E. (2001). Flame Synthesis of Nanoparticles. 24(6), 583–596. doi:10.1002/1521-4125(200106)24:6<583::AID-CEAT583>3.0.CO;2-H
Kanimozhi, V., and Chinnamuthu, C. R. (2012). Engineering core/hallow shell nanomaterials to load herbicide active ingredient for controlled release. Res. J. Nanosci. Nanotechnol. 2 (2), 58–69. doi:10.3923/rjnn.2012.58.69
Kapakoglou, N. I., Giokas, D. L., Tsogas, G. Z., and Vlessidis, A. G. (2008). Coacervation of surface-functionalized polymerized vesicles derived from ammonium bromide surfactants. Applications to the selective speciation of chromium in environmental samples. Anal. Chem. 80 (24), 9787–9796. doi:10.1021/ac802018w
Karaman, D. Ş., Manner, S., Fallarero, A., and Rosenholm, J. M. (2017). Current approaches for exploration of nanoparticles as antibacterial agents. IntechOpen 61–86. doi:10.5772/68138
Karny, A., Zinger, A., Kajal, A., Shainsky-Roitman, J., and Schroeder, A. (2018). Therapeutic nanoparticles penetrate leaves and deliver nutrients to agricultural crops. Sci. Rep. 8 (1), 7589. doi:10.1038/s41598-018-25197-y
Karthikeyan, R., Kumar, P. V., and Koushik, O. S. (2016). Dendrimeric Biocides-a tool for effective antimicrobial therapy. J. Nanomed. Nanotechnol. 7 (359), 2. doi:10.4172/2157-7439.1000359
Katas, H., Lim, C. S., Azlan, A. Y. H. N., Buang, F., and Busra, M. F. M. (2019). Antibacterial activity of biosynthesized gold nanoparticles using biomolecules from Lignosus rhinocerotis and chitosan. Saudi Pharma. J. 27 (2), 283–292. doi:10.1016/j.jsps.2018.11.010
Kaziem, A. E., Gao, Y., Zhang, Y., Qin, X., Xiao, Y., Zhang, Y., et al. (2018). α-Amylase triggered carriers based on cyclodextrin anchored hollow mesoporous silica for enhancing insecticidal activity of avermectin against Plutella xylostella. J. Hazard. Mater. 359, 213–221. doi:10.1016/j.jhazmat.2018.07.059
Keat, C. L., Aziz, A., Eid, A. M., and Elmarzugi, N. A. (2015). Biosynthesis of nanoparticles and silver nanoparticles. Bioresour. Bioproc. 2, 47. doi:10.1186/s40643-015-0076-2
Keswani, C., Bisen, K., Singh, S. P., Sarma, B. K., and Singh, H. B. (2016). “A proteomic approach to understand the tripartite interactions between plant-Trichoderma-pathogen: investigating the potential for efficient biological control,” in Plant, Soil And Microbes: Mechanisms and Molecular Interactions. Editors K. R. Hakeem, and M. S. Akhtar (New York: Springer), 2, 79–93.
Keswani, C., Mishra, S., Sarma, B. K., Singh, S. P., and Singh, H. B. (2014). Unravelling the efficient applications of secondary metabolites of various Trichoderma spp. Appl. Microbiol. Biotechnol. 98, 533–544. doi:10.1007/s00253-013-5344-5
Khan, I., Saeed, K., and Khan, I. (2019a). Nanoparticles: Properties, applications and toxicities. Arab. J. Chem. 12 (7), 908–931. doi:10.1016/j.arabjc.2017.05.011
Khan, M. I., Akhtar, M. N., Ashraf, N., Najeeb, J., Munir, H., Awan, T. I., et al. (2020). Green synthesis of magnesium oxide nanoparticles using Dalbergia sissoo extract for photocatalytic activity and antibacterial efficacy. Appl. Nanosci. 10, 2351–2364. doi:10.1007/s13204-020-01414-x
Khan, M. R., Adam, V., Rizvi, T. F., Zhang, B., Ahamad, F., Jośko, I., et al. (2019b). Nanoparticle–Plant Interactions: Two‐Way Traffic. Small 15 (37), e1901794. doi:10.1002/smll.201901794
Khan, M. R., and Rizvi, T. F. (2014). Nanotechnology: scope and application in plant disease management. Plant Pathol. J. 13 (3), 214–231. doi:10.3923/ppj.2014.214.231
Khandel, P., Yadaw, R. K., Soni, D. K., Kanwar, L., and Shahi, S. K. (2018). Biogenesis of metal nanoparticles and their pharmacological applications: Present status and application prospects. J. Nanostructure Chem. 8, 217–254. doi:10.1007/s40097-018-0267-4
Khandelwal, N., Kaur, G., Kumar, N., and Tiwari, A. (2014). Application of silver nanoparticles in viral inhibition: a new hope for antivirals. Dig. J. Nanomat. Biostruct. 9 (1), 175–186.
Khatami, M., Alijani, H. Q., Heli, H., and Sharifi, I. (2018). Rectangular shaped zinc oxide nanoparticles: Green synthesis by Stevia and its biomedical efficiency. Ceram. Int. 44 (13), 15596–15602. doi:10.1016/j.ceramint.2018.05.224
Khatoon, U. T., Rao, G. N., Mohan, K. M., Ramanaviciene, A., and Ramanavicius, A. (2017). Antibacterial and antifungal activity of silver nanospheres synthesized by tri-sodium citrate assisted chemical approach. Vacuum 146, 259–265. doi:10.1016/j.vacuum.2017.10.003
Khodakovskaya, M., Dervishi, E., Mahmood, M., Xu, Y., Li, Z., Watanabe, F., et al. (2009). Carbon nanotubes are able to penetrate plant seed coat and dramatically affect seed germination and plant growth. ACS Nano 10, 3221–3227. doi:10.1021/nn900887m
Kim, D., Shin, K., Kwon, S. G., and Hyeon, T. (2018). Synthesis and biomedical applications of multifunctional nanoparticles. Adv. Mater. 30 (49), 1802309. doi:10.1002/adma.201802309
Kishen, S., Mehta, A., and Gupta, R. (2020). “Biosynthesis and applications of metal nanomaterials,” in Green Nanomaterials. Editors S. Ahmed, and W. Ali (Singapore: Springer), 126, 157–139. doi:10.1007/978-981-15-3560-4_6
Kitching, M., Ramani, M., and Marsili, E. (2015). Fungal biosynthesis of gold nanoparticles: Mechanism and scale up. Microb. Biotechnol. 8, 904–917. doi:10.1111/1751-7915.12151
Koornneef, A., and Pieterse, C. M. (2008). Cross talk in defense signaling. Plant Physiol. 146 (3), 839–844. doi:10.1104/pp.107.112029
Koper, O. B., Klabunde, J. S., Marchin, G. L., Klabunde, K. J., Stoimenov, P., and Bohra, L. (2002). Nanoscale powders and formulations with biocidal activity toward spores and vegetative cells of bacillus species, viruses, and toxins. Curr. Microbiol. 44 (1), 49–55. doi:10.1007/s00284-001-0073-x
Korani, M., Ghazizadeh, E., Korani, S., Hami, Z., and Mohammadi-Bardbori, A. (2015). Effects of silver nanoparticles on human health. Eur. J. Nanomed. 7 (1), 51–62. doi:10.1515/ejnm-2014-0032
Kumar, A., Gupta, K., Dixit, S., Mishra, K., and Srivastava, S. (2019). A review on positive and negative impacts of nanotechnology in agriculture. Int. J. Environ. Sci. Technol. 16 (4), 2175–2184. doi:10.1007/s13762-018-2119-7
Kumar, K., Gambhir, G., Dass, A., Tripathi, A. K., Singh, A., Jha, A. K., et al. (2020). Genetically modified crops: current status and future prospects. Planta 251, 1–27. doi:10.1007/s00425-020-03372-8
Kumar, N., and Kumbhat, S. (2016). Essentials in Nanoscience and Nanotechnology. Hoboken, NJ: John Wiley & Sons.
Kumar, R., and Lal, S. (2014). Synthesis of organic nanoparticles and their applications in drug delivery and food nanotechnology: A review. J. Nanomater. Mol. Nanotechnol. 3, 4. doi:10.4172/2324-8777.1000150
Kumar, S., Bhanjana, G., Sharma, A., Sidhu, M. C., and Dilbaghi, N. (2014). Synthesis, characterization and on field evaluation of pesticide loaded sodium alginate nanoparticles. Carbohydr. Polym. 101, 1061–1067. doi:10.1016/j.carbpol.2013.10.025
Kumar, S., Kumar, D., and Dilbaghi, N. (2017). Preparation, characterization, and bio-efficacy evaluation of controlled release carbendazim-loaded polymeric nanoparticles. Environ. Sci. Pollut. Res. 24 (1), 926–937. doi:10.1007/s11356-016-7774-y
Kumaraswamy, R. V., Kumari, S., Choudhary, R. C., Pal, A., Raliya, R., Biswas, P., et al. (2018). Engineered chitosan based nanomaterials: bioactivities, mechanisms and perspectives in plant protection and growth. Int. J. Biol. Macromol. 113, 494–506. doi:10.1016/j.ijbiomac.2018.02.130
Kumaraswamy, R. V., Kumari, S., Choudhary, R. C., Sharma, S. S., Pal, A., Raliya, R., et al. (2019). Salicylic acid functionalized chitosan nanoparticle: a sustainable biostimulant for plant. Int. J. Biol. Macromol. 123, 59–69. doi:10.1016/j.ijbiomac.2018.10.202
Kumari, P., Meena, M., Gupta, P., Dubey, M. K., Nath, G., and Upadhyay, R. S. (2018b). Plant growth promoting rhizobacteria and their biopriming for growth promotion in mung bean (Vigna radiata (L.) R. Wilczek). Biocatal. Agric. Biotechnol. 16, 163–171. doi:10.1016/j.bcab.2018.07.030
Kumari, P., Meena, M., and Upadhyay, R. S. (2018a). Characterization of plant growth promoting rhizobacteria (PGPR) isolated from the rhizosphere of Vigna radiata (mung bean). Biocatal. Agric. Biotechnol. 16, 155–162. doi:10.1016/j.bcab.2018.07.029
Kuppusamy, P., Yusoff, M. M., and Govindan, N. (2014). Biosynthesis of metallic nanoparticles using plant derivatives and their new avenues in pharmacological applications - An updated report. Saudi. Pharm. J. 24 (4), 473–484. doi:10.1016/j.jsps.2014.11.013
Lai, F., Wissing, S. A., Müller, R. H., and Fadda, A. M. (2006). Artemisia arborescens L essential oil-loaded solid lipid nanoparticles for potential agricultural application: preparation and characterization. AAPS PharmSciTech. 7 (1), E10. doi:10.1208/pt070102
Lakshmi, I. (2018). Internet of nano things (IoNT): Next organic process step in technology. Int. J. Res. Anal. Rev. (Ijrar) 5 (3), 1–10.
Lala, N. L., Ramaseshan, R., Bojun, L., Sundarrajan, S., Barhate, R. S., Ying‐jun, L., et al. (2007). Fabrication of nanofibers with antimicrobial functionality used as filters: protection against bacterial contaminants. Biotechnol. Bioeng. 97 (6), 1357–1365. doi:10.1002/bit.21351
Lam, S. J., O'Brien-Simpson, N. M., Pantarat, N., Sulistio, A., Wong, E. H., Chen, Y. Y., et al. (2016). Combating multidrug-resistant Gram-negative bacteria with structurally nanoengineered antimicrobial peptide polymers. Nat. Microbiol. 1 (11), 16162. doi:10.1038/nmicrobiol.2016.162
Lara, H. H., Ayala-Nuñez, N. V., Ixtepan-Turrent, L., and Rodriguez-Padilla, C. (2010). Mode of antiviral action of silver nanoparticles against HIV-1. J. Nanobiotechnol. 8 (1), 1–10. doi:10.1186/1477-3155-8-1
Le Ouay, B., and Stellacci, F. (2015). Antibacterial activity of silver nanoparticles: a surface science insight. Nano Today 10 (3), 339–354. doi:10.1016/j.nantod.2015.04.002
Lee, D. E., Koo, H., Sun, I. C., Ryu, J. H., Kim, K., and Kwon, I. C. (2012). Multifunctional nanoparticles for multimodal imaging and theragnosis. Chem. Soc. Rev. 41 (7), 2656–2672. doi:10.1039/c2cs15261d
Lee, K., Conboy, M., Park, H. M., Jiang, F., Kim, H. J., Dewitt, M. A., et al. (2017). Nanoparticle delivery of Cas9 ribonucleoprotein and donor DNA in vivo induces homology-directed DNA repair. Nat. Biomed. Eng. 1 (11), 889–901. doi:10.1038/s41551-017-0137-2
Lesniak, A., Salvati, A., Santos-Martinez, M. J., Radomski, M. W., Dawson, K. A., and Åberg, C. (2013). Nanoparticle adhesion to the cell membrane and its effect on nanoparticle uptake efficiency. J. Am. Chem. Soc. 135 (4), 1438–1444. doi:10.1021/ja309812z
Leung, Y. H., Xu, X., Ma, A. P., Liu, F., Ng, A. M., Shen, Z., et al. (2016). Toxicity of ZnO and TiO2 to Escherichia coli cells. Sci. Rep. 6, 35243–35249. doi:10.1038/srep35243
Lew, T. T. S., Koman, V. B., Gordiichuk, P., Park, M., and Strano, M. S. (2020). The emergence of plant nanobionics and living plants as technology. Adv. Mater. Technol. 5 (3), 1900657. doi:10.1002/admt.201900657
Li, B., and Logan, B. E. (2004). Bacterial adhesion to glass and metal-oxide surfaces. Colloids Surf. B: Biointerfaces 36 (2), 81–90. doi:10.1016/j.colsurfb.2004.05.006
Li, C., Wang, X., Chen, F., Zhang, C., Zhi, X., Wang, K., et al. (2013). The antifungal activity of graphene oxide–silver nanocomposites. Biomaterials 34 (15), 3882–3890. doi:10.1016/j.biomaterials.2013.02.001
Li, S., Zhang, L., Wang, T., Li, L., Wang, C., and Su, Z. (2015). The facile synthesis of hollow Au nanoflowers for synergistic chemo-photothermal cancer therapy. Chem. Commun. 51, 14338–14341. doi:10.1039/C5CC05676D
Li, Z., and Wong, S. L. (2017). Functionalization of 2D transition metal dichalcogenides for biomedical applications. Mater. Sci. Eng. C. 70, 1095–1106. doi:10.1016/j.msec.2016.03.039
Li, Z. Z., Chen, J. F., Liu, F., Liu, A. Q., Wang, Q., Sun, H. Y., et al. (2007). Study of UV‐shielding properties of novel porous hollow silica nanoparticle carriers for avermectin. Pest Manag. Sci. 63 (3), 241–246. doi:10.1002/ps.1301
Li, Z. Z., Xu, S. A., Wen, L. X., Liu, F., Liu, A. Q., Wang, Q., et al. (2006). Controlled release of avermectin from porous hollow silica nanoparticles: influence of shell thickness on loading efficiency, UV-shielding property and release. J. Control Release 111 (1-2), 81–88. doi:10.1016/j.jconrel.2005.10.020
Liang, J., Yu, M., Guo, L., Cui, B., Zhao, X., Sun, C., et al. (2017). Bioinspired development of P (St–MAA)–avermectin nanoparticles with high affinity for foliage to enhance folia retention. J. Agric. Food Chem. 66 (26), 6578–6584. doi:10.1021/acs.jafc.7b01998
Liao, S., Zhang, Y., Pan, X., Zhu, F., Jiang, C., Liu, Q., et al. (2019). Antibacterial activity and mechanism of silver nanoparticles against multidrug-resistant Pseudomonas aeruginosa. Int. J. Nanomedicine 14, 1469–1487. doi:10.2147/IJN.S191340
Lin, D., and Xing, B. (2007). Phytotoxicity of nanoparticles: inhibition of seed germination and root growth. Environ. Pollut. 150 (2), 243–250. doi:10.1016/j.envpol.2007.01.016
Linglan, M., Chao, L., Chunxiang, Q., Sitao, Y., Jie, L., Fengqing, G., et al. (2008). Rubisco activase mRNA expression in spinach: modulation by nanoanatase treatment. Biol. Trace Elem. Res. 122 (2), 168–178. doi:10.1007/s12011-007-8069-4
Liu, D., Li, C., Zhou, F., Zhang, T., Zhang, H., Li, X., et al. (2015a). Rapid synthesis of monodisperse Au nanospheres through a laser irradiation-induced shape conversion, self-assembly and their electromagnetic coupling SERS enhancement. Sci. Rep. 5 (1), 7686. doi:10.1038/srep07686
Liu, F., Wen, L. X., Li, Z. Z., Yu, W., Sun, H. Y., and Chen, J. F. (2006). Porous hollow silica nanoparticles as controlled delivery system for water-soluble pesticide. Mater. Res. Bull. 41 (12), 2268–2275. doi:10.1016/j.materresbull.2006.04.014
Liu, J., Gray, W. D., Davis, M. E., and Luo, Y. (2012). Peptide-and saccharide-conjugated dendrimers for targeted drug delivery: a concise review. Interf. Focus 2 (3), 307–324. doi:10.1098/rsfs.2012.0009
Liu, R., Zhang, H., and Lal, R. (2016). Effects of stabilized nanoparticles of copper, zinc, manganese, and iron oxides in low concentrations on lettuce (Lactuca sativa) seed germination: nanotoxicants or nanonutrients?. Water Air Soil Poll. 227 (1), 42. doi:10.1007/s11270-015-2738-2
Liu, S., Wei, L., Hao, L., Fang, N., Chang, M. W., Xu, R., et al. (2009). Sharper and faster “nano darts” kill more bacteria: a study of antibacterial activity of individually dispersed pristine single-walled carbon nanotube. ACS Nano 3 (12), 3891–3902. doi:10.1021/nn901252r
Liu, X., He, B., Xu, Z., Yin, M., Yang, W., Zhang, H., et al. (2015b). A functionalized fluorescent dendrimer as a pesticide nanocarrier: application in pest control. Nanoscale 7 (2), 445–449. doi:10.1039/c4nr05733c
Liu, Y., Laks, P., and Heiden, P. (2002). Controlled release of biocides in solid wood. II. Efficacy against Trametes versicolor and Gloeophyllum trabeum wood decay fungi. J. Appl. Polym. Sci. 86 (3), 608–614. doi:10.1002/app.10897
Liu, Y., Yan, L., Heiden, P., and Laks, P. (2001). Use of nanoparticles for controlled release of biocides in solid wood. J. Appl. Polym. Sci. 79 (3), 458–465. doi:10.1002/1097-4628(20010118)79:3<458::AID-APP80>3.0.CO;2-H
Liversidge, G. G., and Cundy, K. C. (1995). Particle size reduction for improvement of oral bioavailability of hydrophobic drugs: I. Absolute oral bioavailability of nanocrystalline danazol in beagle dogs. Int. J. Pharm. 125 (1), 91–97. doi:10.1016/0378-5173(95)00122-Y
Llorens, A., Lloret, E., Picouet, P. A., Trbojevich, R., and Fernandez, A. (2012). Metallic-based micro and nanocomposites in food contact materials and active food packaging. Trends Food Sci. Technol. 24, 19–29. doi:10.1016/j.tifs.2011.10.001
Loo, Y. Y., Rukayadi, Y., Nor-Khaizura, M. A. R., Kuan, C. H., Chieng, B. W., Nishibuchi, M., et al. (2018). In vitro antimicrobial activity of green synthesized silver nanoparticles against selected gram-negative foodborne pathogens. Front. Microbiol. 9, 1555. doi:10.3389/fmicb.2018.01555
López-Moreno, M. L., de la Rosa, G., Hernández-Viezcas, J. Á., Castillo-Michel, H., Botez, C. E., Peralta-Videa, J. R., et al. (2010). Evidence of the differential biotransformation and genotoxicity of ZnO and CeO2 nanoparticles on soybean (Glycine max) plants. Environ. Sci. Technol. 44 (19), 7315–7320. doi:10.1021/es903891g
Lu, L. Y., Lin, B. J., Liu, J. S., and Yu, C. Y. (2012). Ethics in nanotechnology: What’s being done? What’s missing?. J. Bus. Ethics 109 (4), 583–598. doi:10.1007/s10551-012-1432-1
Lu, W., Lu, M. L., Zhang, Q. P., Tian, Y. Q., Zhang, Z. X., and Xu, H. H. (2013). Octahydrogenated retinoic acid‐conjugated glycol chitosan nanoparticles as a novel carrier of azadirachtin: Synthesis, characterization, and in vitro evaluation. J. Polym. Sci. Part. A: Polym. Chem. 51 (18), 3932–3940. doi:10.1002/pola.26801
Lu, X. (2018). Mesoporous silica nanoparticle delivery of biomolecules into plants. Geelong, Australia: Deakin University.
Lushchak, V. I., Matviishyn, T. M., Husak, V. V., Storey, J. M., and Storey, K. B. (2018). Pesticide toxicity: A mechanistic approach. EXCLI J. 17, 1101–1136. doi:10.17179/excli2018-1710
Lv, J., Christie, P., and Zhang, S. (2019). Uptake, translocation, and transformation of metal-based nanoparticles in plants: recent advances and methodological challenges. Environ. Sci. Nano 6 (1), 41–59. doi:10.1039/C8EN00645H
Mahakham, W., Sarmah, A. K., Maensiri, S., and Theerakulpisut, P. (2017). Nanopriming technology for enhancing germination and starch metabolism of aged rice seeds using phytosynthesized silver nanoparticles. Sci. Rep. 7 (1), 8263. doi:10.1038/s41598-017-08669-5
Mahmoodi, S., Elmi, A., and Hallaj-Nezhadi, S. (2018). Copper nanoparticles as antibacterial agents. J. Mol. Pharm. Org. Process. Res. 6 (1), 140. doi:10.4172/2329-9053.1000140
Majhi, S. M., Naik, G. K., Lee, H. J., Song, H. G., Lee, C. R., Lee, I. H., et al. (2018). Au@NiO core-shell nanoparticles as a p-type gas sensor: Novel synthesis, characterization, and their gas sensing properties with sensing mechanism. Sens. Actuators B: Chem. 268, 223–231. doi:10.1016/j.snb.2018.04.119
Majumdar, R., Rajasekaran, K., and Cary, J. W. (2017). RNA interference (RNAi) as a potential tool for control of mycotoxin contamination in crop plants: concepts and considerations. Front. Plant Sci. 8, 200. doi:10.3389/fpls.2017.00200
Maksimović, M., and Omanović-Mikličanin, E. (2017). “Green internet of things and green nanotechnology role in realizing smart and sustainable agriculture,” in VIII International Scientific Agriculture Symposium “AGROSYM 2017”, October 2017, Jahorina, Bosnia and Herzegovina, 2290–2295.
Malka, E., Perelshtein, I., Lipovsky, A., Shalom, Y., Naparstek, L., Perkas, N., et al. (2013). Eradication of multi-drug resistant bacteria by a novel Zn-doped CuO nanocomposite. Small 9, 4069–4076. doi:10.1002/smll.201301081
Manikandan, V., Jayanthi, P., Priyadharsan, A., Vijayaprathap, E., Anbarasan, P. M., and Velmurugan, P. (2019). Green synthesis of pH-responsive Al2O3 nanoparticles: Application to rapid removal of nitrate ions with enhanced antibacterial activity. J. Photochem. Photobiol. A: Chem. 371, 205–215. doi:10.1016/j.jphotochem.2018.11.009
Mann, S., Burkett, S. L., Davis, S. A., Fowler, C. E., Mendelson, N. H., Sims, S. D., et al. (1997). Sol–gel synthesis of organized matter. Chem. Mater. 9 (11), 2300–2310. doi:10.1021/cm970274u
Mansha, M., Qurashi, A., Ullah, N., Bakare, F. O., Khan, I., and Yamani, Z. H. (2016). Synthesis of In2O3/graphene heterostructure and their hydrogen gas sensing properties. Ceram. Int. 42 (9), 11490–11495. doi:10.1016/j.ceramint.2016.04.035
Manyasree, D., Kiranmayi, P., and Kumar, R. (2018). Synthesis, characterization and antibacterial activity of aluminium oxide nanoparticles. Int. J. Pharm. Pharm. Sci. 10 (1), 32–35. doi:10.22159/ijpps.2018v10i1.20636
Marchiol, L. (2018). “Nanotechnology in agriculture: New opportunities and perspectives,” in New Visions In Plant Science London, UK: InTechOpen. doi:10.5772/intechopen.74425
Martin-Ortigosa, S., Peterson, D. J., Valenstein, J. S., Lin, V. S. Y., Trewyn, B. G., Lyznik, L. A., et al. (2014). Mesoporous silica nanoparticle-mediated intracellular Cre protein delivery for maize genome editing via loxP site excision. Plant Physiol. 164 (2), 537–547. doi:10.1104/pp.113.233650
Maruyama, C. R., Guilger, M., Pascoli, M., Bileshy-José, N., Abhilash, P. C., Fraceto, L. F., et al. (2016). Nanoparticles based on chitosan as carriers for the combined herbicides imazapic and imazapyr. Sci. Rep. 6, 19768. doi:10.1038/srep19768
Mathur, M. (2016). Achievements, constraints and gaps of nano-techniques pertains to augmenting herbal drug efficacy. Med. Plants-Int. J. Phytomedicines Rel. Indus. 8 (3), 171–198. doi:10.5958/0975-6892.2016.00031.9
McClements, D. J., and Xiao, H. (2017). Is nano safe in foods? Establishing the factors impacting the gastrointestinal fate and toxicity of organic and inorganic food-grade nanoparticles. Npj Sci. Food 1 (1), 1–13. doi:10.1038/s41538-017-0005-1
Meena, M., Aamir, M., Vikas, K., Swapnil, P., and Upadhyay, R. S. (2018). Evaluation of morpho-physiological growth parameters of tomato in response to Cd induced toxicity and characterization of metal sensitive NRAMP3 transporter protein. Environ. Exp. Bot. 148, 144–167. doi:10.1016/j.envexpbot.2018.01.007
Meena, M., Divyanshu, K., Kumar, S., Swapnil, P., Zehra, A., Shukla, V., et al. (2019a). Regulation of L-proline biosynthesis, signal transduction, transport, accumulation and its vital role in plants during variable environmental conditions. Heliyon 5 (12), e02951. doi:10.1016/j.heliyon.2019.e02952
Meena, M., Dubey, M. K., Swapnil, P., Zehra, A., Singh, S., Kumari, P., et al. (2017a). “The rhizosphere microbial community and methods of its analysis,” in Advances In PGPR Research. Editors H. B. Singh, B. K. Sarma, and C. Keswani (Wallingford, England: CAB International), 275–295.
Meena, M., Gupta, S. K., Swapnil, P., Zehra, A., Dubey, M. K., and Upadhyay, R. S. (2017b). Alternaria toxins: potential virulence factors and genes related to pathogenesis. Front. Microbiol. 8, 1451. doi:10.3389/fmicb.2017.01451
Meena, M., Prasad, V., and Upadhyay, R. S. (2016a). Assessment of the bioweedicidal effects of Alternaria alternata metabolites against Parthenium species. Bull. Environ. Sci. Res. 5 (1), 1–7.
Meena, M., Prasad, V., and Upadhyay, R. S. (2017d). Evaluation of Alternaria alternata isolates for metabolite production isolated from different sites of Varanasi, India. J. Agric. Res. 2 (1), 12. doi:10.23880/OAJAR-16000124
Meena, M., Prasad, V., and Upadhyay, R. S. (2017c). Evaluation of biochemical changes in leaves of tomato infected with Alternaria alternata and its metabolites. Vegetos 30, 2. doi:10.5958/2229-4473.2017.00020.9
Meena, M., Prasad, V., Zehra, A., Gupta, V. K., and Upadhyay, R. S. (2015). Mannitol metabolism during pathogenic fungal‒host interactions under stressed conditions. Front. Microbiol. 6, 1019–1026. doi:10.3389/fmicb.2015.01019
Meena, M., and Samal, S. (2019). Alternaria host-specific (HSTs) toxins: An overview of chemical characterization, target sites, regulation and their toxic effects. Toxicol. Rep. 6, 745–758. doi:10.1016/j.toxrep.2019.06.021
Meena, M., Sonigra, P., and Yadav, G. (2020a). Biological-based methods for the removal of volatile organic compounds (VOCs) and heavy metals. Environ. Sci. Pollut. Res. 28, 2485–2508. doi:10.1007/s11356-020-11112-4
Meena, M., Swapnil, P., Divyanshu, K., Kumar, S., Zehra, A., Tripathi, Y. N., et al. (2020b). PGPR-mediated induction of systemic resistance and physiochemical alterations in plants against the pathogens: current perspectives. J. Basic Microbiol. 60 (8), 1–34. doi:10.1002/jobm.202000370
Meena, M., and Swapnil, P. (2019). Regulation of WRKY genes in plant defense with beneficial fungus Trichoderma: Current perspectives and future prospects. Arch. Phytopathol. Plant Protect. 52 (1–2), 1–17. doi:10.1080/03235408.2019.1606490
Meena, M., Swapnil, P., and Upadhyay, R. S. (2017e). Isolation, characterization and toxicological potential of tenuazonic acid, alternariol and alternariol monomethyl ether produced by Alternaria species phytopathogenic on plants. Sci. Rep. 7, 8777. doi:10.1038/s41598-017-09138-9
Meena, M., Swapnil, P., Zehra, A., Aamir, M., Dubey, M. K., and Upadhyay, R. S. (2017f). “Beneficial microbes for disease suppression and plant growth promotion,” in Plant-Microbe Interactions In Agro-Ecological Perspectives. Editors D. Singh, H. Singh, and R. Prabha (Singapore: Springer), 395–432. doi:10.1007/978-981-10-6593-4_16
Meena, M., Swapnil, P., Zehra, A., Dubey, M. K., Aamir, M., Patel, C. B., et al. (2019b). “Virulence factors and their associated genes in microbes,” in New And Future Developments In Microbial Biotechnology And Bioengineering. Editors H. B. Singh, V. K. Gupta, and S. Jogaiah (Chennai, TN: Elsevier), 181–208. doi:10.1016/B978-0-444-63503-7.00011-5
Meena, M., Swapnil, P., Zehra, A., Dubey, M. K., and Upadhyay, R. S. (2017g). Antagonistic assessment of Trichoderma spp. by producing volatile and non-volatile compounds against different fungal pathogens. Arch. Phytopathology Plant Protect. 50 (13–14), 629–648. doi:10.1080/03235408.2017.1357360
Meena, M., Tiwari, A., Zehra, A., Prasad, V., and Upadhyay, R. S. (2013). Morphological and molecular identification of alternaria alternata from tomato. RGSC, Barkachha, BHU 1, 506–509.
Meena, M., Zehra, A., Dubey, M. K., Aamir, M., Gupta, V. K., and Upadhyay, R. S. (2016b). Comparative evaluation of biochemical changes in tomato (Lycopersicon esculentum Mill.) infected by Alternaria alternata and its toxic metabolites (TeA, AOH, and AME). Front. Plant Sci. 7, 1408. doi:10.3389/fpls.2016.01408
Meena, M., Zehra, A., Dubey, M. K., Aamir, M., and Upadhyay, R. S. (2017h). “Penicillum enzymes for the food industries,” in New And Future Developments In Microbial Biotechnology And Bioengineering. Editors V. K. Gupta, and S. Rodriguez-Couto (Chennai, TN: Elsevier), 167–186. doi:10.1016/B978-0-444-63501-3.00014-4
Meena, M., Zehra, A., Dubey, M. K., and Upadhyay, R. S. (2016c). Mannitol and proline accumulation in Lycopersicum esculentum during infection of Alternaria alternata and its toxins. Int. J. Biomed. Sci. Bioinformat. 3 (2), 64–68.
Meena, M., Zehra, A., Swapnil, P., Dubey, M. K., Patel, C. B., and Upadhyay, R. S. (2017i). Effect on lycopene, β-carotene, ascorbic acid and phenolic content in tomato fruits infected by Alternaria alternata and its toxins (TeA, AOH and AME). Arch. Phytopathol. Plant Protect. 50 (7–8), 317–329. doi:10.1080/03235408.2017.1312769
Meena, M., and Zehra, A. (2019). Tomato: A model plant to study plant-pathogen interactions. Food Sci. Nutr. Technol. 4 (1), 000171. doi:10.23880/fsnt-16000171
Mei, X. D., Liang, Y. H., Zhang, T., Ning, J., and Wang, Z. Y. (2014). “An amphiphilic chitosan-polylactide graft copolymer and its nanoparticles as fungicide carriers,” in Advanced Materials Research 1051. Switzerland: Trans Tech Publications Ltd., 21–28.
Meléndez-Villanueva, M. A., Morán-Santibañez, K., Martínez-Sanmiguel, J. J., Rangel-López, R., Garza-Navarro, M. A., Rodríguez-Padilla, C., et al. (2019). Virucidal activity of gold nanoparticles synthesized by green chemistry using garlic extract. Viruses 11 (12), 1111. doi:10.3390/v11121111
Meredith, A. N., Harper, B., and Harper, S. L. (2016). The influence of size on the toxicity of an encapsulated pesticide: a comparison of micron-and nano-sized capsules. Environ. Int. 86, 68–74. doi:10.1016/j.envint.2015.10.012
Merisko-Liversidge, E., Liversidge, G. G., and Cooper, E. R. (2003). Nanosizing: a formulation approach for poorly-water-soluble compounds. Eur. J. Pharm. Sci. 18 (2), 113–120. doi:10.1016/S0928-0987(02)00251-8
Messaoudi, O., Bendahou, M., Benamar, I., and Abdelwouhid, D. E. (2015). Identification and preliminary characterization of non-polyene antibiotics secreted by new strain of actinomycete isolated from sebkha of Kenadsa, Algeria. Asian Pac. J. Trop. Biomed. 5, 438–445. doi:10.1016/j.apjtb.2015.04.002
Messaoudi, O., and Bendahou, M. (2020). “Biological synthesis of nanoparticles using endophytic microorganisms: Current development,” in Nanotechnology And the Environment. London, UK: Intechopen. doi:10.5772/intechopen.93734
Miraz, M. H., Ali, M., Excell, P. S., and Picking, R. (2018). Internet of nano-things, things and everything: future growth trends. Future Internet 10 (8), 68. doi:10.3390/fi10080068
Mitter, N., Worrall, E. A., Robinson, K. E., Li, P., Jain, R. G., Taochy, C., et al. (2017). Clay nanosheets for topical delivery of RNAi for sustained protection against plant viruses. Nat. Plants 3 (2), 16207. doi:10.1038/nplants.2016.207
Moghaddam, A. B., Namvar, F., Moniri, M., Tahir, P. M., Azizi, S., and Mohamad, R. (2015). Nanoparticles biosynthesized by fungi and yeast: A review of their preparation, properties, and medical applications. Molecules 20 (9), 16540–16565. doi:10.3390/molecules200916540
Moghaddasi, S., Khoshgoftarmanesh, A. H., Karimzadeh, F., and Chaney, R. L. (2013). Preparation of nano-particles from waste tire rubber and evaluation of their effectiveness as zinc source for cucumber in nutrient solution culture. Sci. Horticult. 160, 398–403. doi:10.1016/j.scienta.2013.06.028
Mogilevsky, G., Hartman, O., Emmons, E. D., Balboa, A., DeCoste, J. B., Schindler, B. J., et al. (2014). Bottom-up synthesis of anatase nanoparticles with graphene domains. ACS Appl. Mater. Inter. 6 (13), 10638–10648. doi:10.1021/am502322y
Mohammadi, S., Harvey, A., and Boodhoo, K. V. K. (2014). Synthesis of TiO2 nanoparticles in a spinning disc reactor. Chem. Eng. J. 258, 171–184. doi:10.1016/j.cej.2014.07.042
Monych, N. K., Gugala, N., and Turner, R. J. (2019). “Metal-based antimicrobials,” in Antimicrobial Materials For Biomedical Applications. Editors A. J. Domb, K. R. Kunduru, and S. Farah (Burlington House, UK: Royal Society of Chemistry), 5, 252–276. doi:10.1039/9781788012638-00252
Moraes, C. M., de Matos, A. P., Grillo, R., de Melo, N. F., de Paula, E., Dias, F. N., et al. (2011). Screening of formulation variables for the preparation of poly (epsilon-caprolactone) nanocapsules containing the local anesthetic benzocaine. J. Nanosci. Nanotechnol. 11 (3), 2450–2457. doi:10.1166/jnn.2011.3547
Morris, V. J. (2011). Emerging roles of engineered nanomaterials in the food industry. Trends Biotechnol. 29 (10), 509–516. doi:10.1016/j.tibtech.2011.04.010
Moulick, R. G., Das, S., Debnath, N., and Bandyopadhyay, K. (2020). Potential use of nanotechnology in sustainable and ‘smart’agriculture: advancements made in the last decade. Plant Biotechnol. Rep. 14, 505–513. doi:10.1007/s11816-020-00636-3
Mthethwa, T. P., Moloto, M. J., De Vries, A., and Matabola, K. P. (2011). Properties of electrospun CdS and CdSe filled poly (methyl methacrylate) (PMMA) nanofibres. Mater. Res. Bull. 46 (4), 569–575. doi:10.1016/j.materresbull.2010.12.022
Nabila, M. I., and Kannabiran, K. (2018). Biosynthesis, characterization and antibacterial activity of copper oxide nanoparticles (CuO NPs) from actinomycetes. Biocat. Agric. Biotechnol. 15, 56–62. doi:10.1016/j.bcab.2018.05.011
Nadendla, S. R., Rani, T. S., Vaikuntapu, P. R., Maddu, R. R., and Podile, A. R. (2018). HarpinPss encapsulation in chitosan nanoparticles for improved bioavailability and disease resistance in tomato. Carbohydr. Polym. 199, 11–19. doi:10.1016/j.carbpol.2018.06.094
Nair, R., Varghese, S. H., Nair, B. G., Maekawa, T., Yoshida, Y., and Kumar, D. S. (2010). Nanoparticulate material delivery to plants. Plant Sci. 179 (3), 154–163. doi:10.1016/j.plantsci.2010.04.012
Naqvi, S., Maitra, A. N., Abdin, M. Z., Akmal, M. D., Arora, I., and Samim, M. D. (2012). Calcium phosphate nanoparticle mediated genetic transformation in plants. J. Mat. Chem. 22 (8), 3500–3507. doi:10.1039/C2JM11739H
Naseer, M., Aslam, U., Khalid, B., and Chen, B. (2020). Green route to synthesize Zinc Oxide Nanoparticles using leaf extracts of Cassia fistula and Melia azedarach and their antibacterial potential. Sci. Rep. 10 (1), 9055. doi:10.1038/s41598-020-65949-3
Nasseri, M., Golmohammadzadeh, S., Arouiee, H., Jaafari, M. R., and Neamati, H. (2016). Antifungal activity of Zataria multiflora essential oil-loaded solid lipid nanoparticles in-vitro condition. Iranj. Basic Med. Sci. 19 (11), 1231–1237.
Navarro, E., Piccapietra, F., Wagner, B., Marconi, F., Kaegi, R., Odzak, N., et al. (2008). Toxicity of silver nanoparticles to Chlamydomonas reinhardtii. Environ. Sci. Technol. 42 (23), 8959–8964. doi:10.1021/es801785m
Nayantara , , and Kaur, P. (2018). Biosynthesis of nanoparticles using eco-friendly factories and their role in plant pathogenicity: a review. Biotechnol. Res. Inno. 2 (1), 63–73. doi:10.1016/j.biori.2018.09.003
Nayyar, A., and Puri, V. (2016). Data glove: internet of things (IoT) based smart wearable gadget. Br. J. Math. Comput. Sci. 15 (5), 1–12. doi:10.9734/BJMCS/2016/24854
Nayyar, A., Puri, V., and Le, D. N. (2017). Internet of nano things (IoNT): Next evolutionary step in nanotechnology. Nanosci. Nanotechnol. 7 (1), 4–8. doi:10.5923/j.nn.20170701.02
Needham, D., Arslanagic, A., Glud, K., Hervella, P., Karimi, L., Høeilund-Carlsen, P. F., et al. (2016). Bottom up design of nanoparticles for anti-cancer diapeutics: “Put the drug in the cancer’s food”. J. Drug Target. 24 (9), 836–856. doi:10.1080/1061186X.2016.1238092
Nganga, S., Travan, A., Marsich, E., Donati, I., Söderling, E., Moritz, N., et al. (2013). In vitro antimicrobial properties of silver–polysaccharide coatings on porous fiber-reinforced composites for bone implants. J. Mater. Sci. Mater. Med. 24, 2775–2785. doi:10.1007/s10856-013-5022-2
Nguyen, H. M., Hwang, I. C., Park, J. W., and Park, H. J. (2012a). Enhanced payload and photo-protection for pesticides using nanostructured lipid carriers with corn oil as liquid lipid. J. Microencapsul. 29 (6), 596–604. doi:10.3109/02652048.2012.668960
Nguyen, H. M., Hwang, I. C., Park, J. W., and Park, H. J. (2012b). Photoprotection for deltamethrin using chitosan‐coated beeswax solid lipid nanoparticles. Pest Manag. Sci. 68 (7), 1062–1068. doi:10.1002/ps.3268
Nguyen, N. Y. T., Grelling, N., Wetteland, C. L., Rosario, R., and Liu, H. (2018). Antimicrobial activities and mechanisms of magnesium oxide nanoparticles (nMgO) against pathogenic bacteria, yeasts, and biofilms. Sci. Rep. 8 (1), 16260. doi:10.1038/s41598-018-34567-5
Nguyen, T. N. Q., Hua, Q. C., and Nguyen, T. T. (2014). Enhancing insecticide activity of anacardic acid by intercalating it into MgAl layered double hydroxides nanoparticles. J. Viet. Environ. 6 (3), 208–211. doi:10.13141/jve.vol6.no3.pp208-211
Nida, M., Khan, I. U., and Alam, S. M. K. (2015). “Towards smart agriculture: An introduction,” in Smart Agriculture: An Approach towards Better Agriculture Management. Editor A. Rehman (Hyderabad, India: OMICS Group eBooks), 1–10. doi:10.4172/978-1-63278-023-2-024
Niño-Martínez, N., Salas Orozco, M. F., Martínez-Castañón, G. A., Torres Méndez, F., and Ruiz, F. (2019). Molecular mechanisms of bacterial resistance to metal and metal oxide nanoparticles. Int. J. Mol. Sci. 20, 2808–2823. doi:10.3390/ijms20112808
Noori, A. J., and Kareem, F. A. (2019). The effect of magnesium oxide nanoparticles on the antibacterial and antibiofilm properties of glass-ionomer cement. Heliyon 5 (10), e02568. doi:10.1016/j.heliyon.2019.e02568
Oves, M., Qari, H. A., Felemban, N. M., Khan, M. Z., Rehan, Z. A., and Ismail, I. M. (2016). Marinobacter lipolyticus from Red Sea for lipase production and modulation of silver nanomaterials for anti-candidal activities. IET Nanobiotechnol. 11 (4), 403–410. doi:10.1049/iet-nbt.2016.0104
Ovsyannikov, V. A., Zamoryanskaya, M. V., Semencha, A. V., Lycheva, K. A., Kol'tsova, T. S., Tolochko, O. V., et al. (2015). Development of bismuth oxide-based nanopreparation for the destruction of malignant neoplasms: theoretical prerequisites, challenges, and practical approaches. Glass Phys. Chem. 41, 533–536. doi:10.1134/S1087659615050119
Padalia, H., Moteriya, P., and Chanda, S. (2015). Green synthesis of silver nanoparticles from marigold flower and its synergistic antimicrobial potential. Arab. J. Chem. 8 (5), 732–741. doi:10.1016/j.arabjc.2014.11.015
Padrela, L., Rodrigues, M. A., Duarte, A., Dias, A. M., Braga, M. E., and de Sousa, H. C. (2018). Supercritical carbon dioxide-based technologies for the production of drug nanoparticles/nanocrystals–a comprehensive review. Adv. Drug Deli. Rev. 131, 22–78. doi:10.1016/j.addr.2018.07.010
Palmqvist, N. G. M., Seisenbaeva, G. A., Svedlindh, P., and Kessler, V. G. (2017). Maghemite nanoparticles acts as nanozymes, improving growth and abiotic stress tolerance in Brassica napus. Nanoscale Res. Lett. 12 (1), 631. doi:10.1186/s11671-017-2404-2
Park, T. J., Lee, K. G., and Lee, S. Y. (2016). Advances in microbial biosynthesis of metal nanoparticles. Appl. Microbiol. Biotechnol. 100, 521–534. doi:10.1007/s00253-015-6904-7
Parveen, R., and Tremiliosi-Filho, G. (2016). A step ahead towards the green synthesis of monodisperse gold nanoparticles: the use of crude glycerol as a greener and low-cost reducing agent. RSC Adv. 6 (97), 95210–95219. doi:10.1039/C6RA14259A
Pasupathy, K., Lin, S., Hu, Q., Luo, H., and Ke, P. C. (2008). Direct plant gene delivery with a poly (amidoamine) dendrimer. Biotechnol. J. Healthc. Nut. Technol. 3 (8), 1078–1082. doi:10.1002/biot.200800021
Patil, V. C., Al-Gaadi, K. A., Biradar, D. P., and Rangaswamy, N. (2012). Internet of things (IoT) and cloud computing for agriculture: An Overview. Agro-Informatics and Precision Agriculture 2012 (AIPA 2012), September 1, 2018, Hyderabad, India, 292–297.
Patra, J. K., and Baek, K. H. (2014). Green nanobiotechnology: Factors affecting synthesis and characterization techniques. J. Nanomater. 2014, 219. doi:10.1155/2014/417305
Paulraj, M. G., Ignacimuthu, S., Gandhi, M. R., Shajahan, A., Ganesan, P., Packiam, S. M., et al. (2017). Comparative studies of tripolyphosphate and glutaraldehyde cross-linked chitosan-botanical pesticide nanoparticles and their agricultural applications. Int. J. Biol. Macromol. 104, 1813–1819. doi:10.1016/j.ijbiomac.2017.06.043
Payne, J. N., Waghwani, H. K., Connor, M. G., Hamilton, W., Tockstein, S., Moolani, H., et al. (2016). Novel synthesis of kanamycin conjugated gold nanoparticles with potent antibacterial activity. Front. Microbiol. 7, 607. doi:10.3389/fmicb.2016.00607
Pazos-Ortiz, E., Roque-Ruiz, J. H., Hinojos-Márquez, E. A., López-Esparza, J., Donohué-Cornejo, A., Cuevas-González, J. C., et al. (2017). Dose-dependent antimicrobial activity of silver nanoparticles on polycaprolactone fibers against gram-positive and gram-negative bacteria. J. Nanomat. 2017, 4752314. doi:10.1155/2017/4752314
Pérez‐de‐Luque, A., and Rubiales, D. (2009). Nanotechnology for parasitic plant control. Pest Manag. Sci. 65 (5), 540–545. doi:10.1002/ps.1732
Pérez-de-Luque, A. (2017). Interaction of nanomaterials with plants: what do we need for real applications in agriculture?. Front. Environ. Sci. 5, 12. doi:10.3389/fenvs.2017.00012
Pirzadah, B., Pirzadah, T. B., Jan, A., and Hakeem, K. R. (2020). “Nanofertilizers: A way forward for green economy,” in Nanobiotechnology in Agriculture. Cham, Switzerland: Springer, 99–112. doi:10.1007/978-3-030-39978-8_5
Prabhu, S. G., and Pattabi, B. M. (2012). Incorporation of acetoacetanilide crystals in host PMMA polymer matrix and characterizations of the hybrid composite. J. Minerals Mater. Characterization Eng. 11 (5), 519–527. doi:10.4236/jmmce.2012.115037
Prasanna, S. S., Balaji, K., Pandey, S., and Rana, S. (2019). “Metal oxide based nanomaterials and their polymer nanocomposites,” in Nanomaterials And Polymer Nanocomposites. Editor N. Karak (Amsterdam, Netherlands: Elsevier), 123–144. doi:10.1016/B978-0-12-814615-6.00004-7
Prashanth, P. A., Raveendra, R. S., Hari Krishna, R., Ananda, S., Bhagya, N. P., Nagabhushana, B. M., et al. (2015). Synthesis, characterizations, antibacterial and photoluminescence studies of solution combustion-derived α-Al2O3 nanoparticles. J. Asian Cer. Soc. 3 (3), 345–351. doi:10.1016/j.jascer.2015.07.001
Prathna, T. C., Lazar Mathew, N. C., Ashok, M., and Raichur, A. M. (2010). “Biomimetic synthesis of nanoparticles: Science, technology & amp,” in Applicability, Biomimetics Learning From Nature. Editor M. Amitava (Rijeka: IntechOpen). doi:10.5772/8776.2010
Qais, F. A., Shafiq, A., Khan, H. M., Husain, F. M., Khan, R. A., Alenazi, B., et al. (2019). Antibacterial effect of silver nanoparticles synthesized using Murraya koenigii (L.) against multidrug-resistant pathogens. Bioinorg. Chem. Appl. 2019, 4649506. doi:10.1155/2019/4649506
Qamar, H., Rehman, S., Chauhan, D. K., Tiwari, A. K., and Upmanyu, V. (2020). Green synthesis, characterization and antimicrobial activity of copper oxide nanomaterial derived from Momordica charantia. Int. J. Nanomedicine 15, 2541–2553. doi:10.2147/IJN.S240232
Qi, M., Liu, Y., and Li, T. (2013). Nano-TiO2 improve the photosynthesis of tomato leaves under mild heat stress. Biol. Trace Elem. Res. 156 (1–3), 323–328. doi:10.1007/s12011-013-9833-2
Qian, K., Shi, T., He, S., Luo, L., and Cao, Y. (2013). Release kinetics of tebuconazole from porous hollow silica nanospheres prepared by miniemulsion method. Microporous Mesoporous Mater. 169, 1–6. doi:10.1016/j.micromeso.2012.10.017
Qian, K., Shi, T., Tang, T., Zhang, S., Liu, X., and Cao, Y. (2011). Preparation and characterization of nano-sized calcium carbonate as controlled release pesticide carrier for validamycin against Rhizoctonia solani. Microchim. Acta 173 (1-2), 51–57. doi:10.1007/s00604-010-0523-x
Qiu, S., Zhou, H., Shen, Z., Hao, L., Chen, H., and Zhou, X. (2020). Synthesis, characterization, and comparison of antibacterial effects and elucidating the mechanism of ZnO, CuO and CuZnO nanoparticles supported on mesoporous silica SBA-3. RSC Adv. 10 (5), 2767–2785. doi:10.1039/C9RA09829A
Rai, A., Prabhune, A., and Perry, C. C. (2010). Antibiotic mediated synthesis of gold nanoparticles with potent antimicrobial activity and their application in antimicrobial coatings. J. Mat. Chem. 20 (32), 6789–6798. doi:10.1039/C0JM00817F
Rai, M., Bansod, S., Bawaskar, M., Gade, A., dos Santos, C. A., Seabra, A. B., et al. (2015). “Nanoparticles-based delivery systems in plant genetic transformation,” in Nanotechnologies In Food And Agriculture. Editors M. Rai, C. Ribeiro, L. Mattoso, and N. Duran (Cham, Switzerland: Springer), 209–239. doi:10.1007/978-3-319-14024-7_10
Rai, M. K., Deshmukh, S. D., Ingle, A. P., and Gade, A. K. (2012). Silver nanoparticles: the powerful nanoweapon against multidrug‐resistant bacteria. J. Appl. Microbiol. 112 (5), 841–852. doi:10.1111/j.1365-2672.2012.05253.x
Rajakumar, G., Rahuman, A. A., Roopan, S. M., Khanna, V. G., Elango, G., Kamaraj, C., et al. (2012). Fungus-mediated biosynthesis and characterization of TiO2 nanoparticles and their activity against pathogenic bacteria. Spectrochimica Acta Part. A: Mol. Biomol. Spectro. 91, 23–29. doi:10.1016/j.saa.2012.01.011
Rajasekaran, P., and Santra, S. (2015). Hydrothermally treated chitosan hydrogel loaded with copper and zinc particles as a potential micronutrient-based antimicrobial feed additive. Front. Vet. Sci. 2, 62. doi:10.3389/fvets.2015.00062
Raliya, R., Franke, C., Chavalmane, S., Nair, R., Reed, N., and Biswas, P. (2016). Quantitative understanding of nanoparticle uptake in watermelon plants. Front. Plant Sci. 7, 1288. doi:10.3389/fpls.2016.01288
Ramacharyulu, P. V. R. K., Muhammad, R., Kumar, J. P., Prasad, G. K., and Mohanty, P. (2015). Iron phthalocyanine modified mesoporous titania nanoparticles for photocatalytic activity and CO2 capture applications. Phys. Chem. Chem. Phys. 17 (39), 26456–26462. doi:10.1039/C5CP03576G
Ramalingam, V. (2019). Multifunctionality of gold nanoparticles: plausible and convincing properties. Adv. Colloid Int. Sci. 271, 101989. doi:10.1016/j.cis.2019.101989
Ramalingam, V., Raja, S., Sundaramahalingam, T. S., and Rajaram, R. (2019). Chemical fabrication of graphene oxide nanosheets attenuates biofilm formation of human clinical pathogens. Bioorg. Chem. 83, 326–335. doi:10.1016/j.bioorg.2018.10.052
Ramalingam, V., Rajaram, R., Premkumar, C., Santhanam, P., Dhinesh, P., Vinothkumar, S., et al. (2014). Biosynthesis of silver nanoparticles from deep sea bacterium Pseudomonas aeruginosa JQ989348 for antimicrobial, antibiofilm, and cytotoxic activity. J. Basic Microbiol. 54, 928–936. doi:10.1002/jobm.201300514
Ramesh, R., Kavitha, P., Kanipandian, N., Arun, S., Thirumurugan, R., and Subramanian, P. (2013). Alteration of antioxidant enzymes and impairment of DNA in the SiO2 nanoparticles exposed zebra fish (Danio rerio). Environ. Monit. Assess. 185 (7), 5873–5881. doi:10.1007/s10661-012-2991-4
Rana, K. L., Kour, D., Yadav, N., and Yadav, A. N. (2020). “Endophytic microbes in nanotechnology: Current development, and potential biotechnology applications,” in Microbial Endophytes. Editors A. Kumar, and V. K. Singh (Cambridge, MA: Woodhead Publishing), 231–262. doi:10.1016/B978-0-12-818734-0.00010-3
Rani, P. U., Madhusudhanamurthy, J., and Sreedhar, B. (2014). Dynamic adsorption of α-pinene and linalool on silica nanoparticles for enhanced antifeedant activity against agricultural pests. J. Pest Sci. 87 (1), 191–200. doi:10.1007/s10340-013-0538-2
Ranjani, S., Shariq, A. M., Mohd, A., Senthil, K. N., Ruckmani, K., and Hemalatha, S. (2020). Synthesis, characterization and applications of endophytic fungal nanoparticles. Inorg. Nano-met. Chem. 27 (2), 706–712. doi:10.1080/24701556.2020.178423
Rastogi, A., Tripathi, D. K., Yadav, S., Chauhan, D. K., Živčák, M., Ghorbanpour, M., et al. (2019). Application of silicon nanoparticles in agriculture. Biotech. 9 (3), 90. doi:10.1007/s13205-019-1626-7
Rattanata, N., Klaynongsruang, S., Leelayuwat, C., Limpaiboon, T., Lulitanond, A., Boonsiri, P., et al. (2016). Gallic acid conjugated with gold nanoparticles: antibacterial activity and mechanism of action on foodborne pathogens. Int. J. Nanomedicine 11, 3347–3356. doi:10.2147/IJN.S109795
Ravoo, B. J. (2008). Nanofabrication with metal containing dendrimers. Dalton Trans. (12), 1533–1537. doi:10.1039/B718133G
Regiel-Futyra, A., Kus-Liśkiewicz, M., Sebastian, V., Irusta, S., Arruebo, M., Kyzioł, A., et al. (2017). Development of noncytotoxic silver–chitosan nanocomposites for efficient control of biofilm forming microbes. RSC Adv. 7 (83), 52398–52413. doi:10.1039/c7ra08359a
Remédios, C., Rosário, F., and Bastos, V. (2012). Environmental nanoparticles interactions with plants: Morphological, physiological, and genotoxic aspects. J. Bot. 2012, 751686. doi:10.1155/2012/751686
Ren, G., Hu, D., Cheng, E. W., Vargas-Reus, M. A., Reip, P., and Allaker, R. P. (2009). Characterisation of copper oxide nanoparticles for antimicrobial applications. Int. J. Antimicrob. Agents 33 (6), 587–590. doi:10.1016/j.ijantimicag.2008.12.004
Richards, R., Li, W., Decker, S., Davidson, C., Koper, O., Zaikovski, V., et al. (2000). Consolidation of metal oxide nanocrystals. Reactive pellets with controllable pore structure that represent a new family of porous, inorganic materials. J. Am. Chem. Soc. 122 (20), 4921–4925. doi:10.1021/ja994383g
Robinson, K. E., Worrall, E. A., and Mitter, N. (2014). Double stranded RNA expression and its topical application for non-transgenic resistance to plant viruses. J. Plant Biochem. Biotechnol. 23 (3), 231–237. doi:10.1007/s13562-014-0260-z
Rolland, J. P., Harberg, E. C., Denison, G. M., Carter, K. R., and DeSimone, J. M. (2004). High-resolution soft lithography: enabling materials for nanotechnologies. Angew. Chem. Int. Ed. 43 (43), 5796–5799. doi:10.1002/anie.200461122
Romero, G., and Moya, S. E. (2012). “Synthesis of organic nanoparticles,” in Nanobiotechnology: Inorganic Nanoparticles Vs Organic Nanoparticles. Frontiers of Nanoscience. Editors J. M. de la Fuente, and V. Grazu (Amsterdam, Netherlands: Elsevier), 4, 115–141. doi:10.1016/B978-0-12-415769-9.00004-2
Rossi, L., Zhang, W., Schwab, A. P., and Ma, X. (2017). Uptake, accumulation, and in planta distribution of coexisting cerium oxide nanoparticles and cadmium in Glycine max (L.) Merr. Environ. Sci. Technol. 51 (21), 12815–12824. doi:10.1021/acs.est.7b03363
Rouphael, Y., and Colla, G. (2020). Biostimulants in agriculture. Front. Plant Sci. 11, 40. doi:10.3389/fpls.2020.00040
Roy, A., Bulut, O., Some, S., Mandal, A. K., and Yilmaz, M. D. (2019). Green synthesis of silver nanoparticles: biomolecule-nanoparticle organizations targeting antimicrobial activity. RSC Adv. 9 (5), 2673–2702. doi:10.1039/C8RA08982E
Roy, A. S., Parveen, A., Koppalkar, A. R., and Prasad, M. A. (2010). Effect of nano-titanium dioxide with different antibiotics against methicillin-resistant Staphylococcus aureus. J. Biomater. Nanobiotechnol. 1, 37–49. doi:10.4236/jbnb.2010.11005
Ruiz, G., Tripathi, K., Okyem, S., and Driskell, J. D. (2019). pH impacts the orientation of antibody adsorbed onto gold nanoparticles. Bioconjug. Chem. 30 (4), 1182–1191. doi:10.1021/acs.bioconjchem.9b00123
Ruttkay-Nedecky, B., Krystofova, O., Nejdl, L., and Adam, V. (2017). Nanoparticles based on essential metals and their phytotoxicity. J. Nanobiotechnol. 15 (1), 33. doi:10.1186/s12951-017-0268-3
Saharan, V., and Pal, A. (2016). Chitosan based nanomaterials in plant growth and protection. New Delhi, India: Springer, 33–41. doi:10.1007/978-81-322-3601-6
Salas-Orozco, M., Niño-Martínez, N., Martínez-Castañón, G. A., Méndez, F. T., Jasso, M. E. C., and Ruiz, F. (2019). Mechanisms of resistance to silver nanoparticles in endodontic bacteria: a literature review. J. Nanomater. 2019, 7630316. doi:10.1155/2019/7630316
Salem, S. S., and Fouda, A. (2020). Green synthesis of metallic nanoparticles and their prosective biotechnological applications: an overview. Biol. Trace Elem. Res. 2020. doi:10.1007/s12011-020-02138-3
Sánchez-López, E., Gomes, D., Esteruelas, G., Bonilla, L., Lopez-Machado, A. L., Galindo, R., et al. (2020). Metal-based nanoparticles as antimicrobial agents: an overview. Nanomaterials 10 (2), 292. doi:10.3390/nano10020292
Santana, I., Wu, H., Hu, P., and Giraldo, J. P. (2020). Targeted delivery of nanomaterials with chemical cargoes in plants enabled by a biorecognition motif. Nat. Commun. 11 (1), 2045. doi:10.1038/s41467-020-15731-w
Sanzari, I., Leone, A., and Ambrosone, A. (2019). Nanotechnology in plant science: to make a long story short. Front. Bioeng. Biotechnol. 7, 120. doi:10.3389/fbioe.2019.00120
Sarlak, N., Taherifar, A., and Salehi, F. (2014). Synthesis of nanopesticides by encapsulating pesticide nanoparticles using functionalized carbon nanotubes and application of new nanocomposite for plant disease treatment. J. Agric. Food Chem. 62 (21), 4833–4838. doi:10.1021/jf404720d
Sathiyabama, M., and Manikandan, A. (2018). Application of copper-chitosan nanoparticles stimulate growth and induce resistance in finger millet (Eleusine coracana Gaertn.) plants against blast disease. J. Agric. Food Chem. 66 (8), 1784–1790. doi:10.1021/acs.jafc.7b05921
Sawangphruk, M., Srimuk, P., Chiochan, P., Sangsri, T., and Siwayaprahm, P. (2012). Synthesis and antifungal activity of reduced graphene oxide nanosheets. Carbon 50 (14), 5156–5161. doi:10.1016/j.carbon.2012.06.056
Bhatia, S. (2016). “Nanoparticles types, classification, characterization, fabrication methods and drug delivery applications,” in Natural Polymer Drug Delivery Systems (Cham, Switzerland: Springer), 33–93. doi:10.1007/978-3-319-41129-3_2
Schwab, F., Zhai, G., Kern, M., Turner, A., Schnoor, J. L., and Wiesner, M. R. (2016). Barriers, pathways and processes for uptake, translocation and accumulation of nanomaterials in plants – Critical review. Nanotoxicology 10 (3), 257–278. doi:10.3109/17435390.2015.1048326
Segota, S., and Tezˇak, Ð. (2006). Spontaneous formation of vesicles. Adv. Colloid Interf. Sci. 121 (1-3), 51–75. doi:10.1016/j.cis.2006.01.002
Shah, M., Fawcett, D., Sharma, S., Tripathy, S. K., and Poinern, G. E. J. (2015). Green of metallic synthesis nanoparticles via biological entities. Material 8, 7278–7308. doi:10.3390/ma8115377
Shahriary, M., Veisi, H., Hekmati, M., and Hemmati, S. (2018). In situ green synthesis of Ag nanoparticles on herbal tea extract (Stachys lavandulifolia)-modified magnetic iron oxide nanoparticles as antibacterial agent and their 4-nitrophenol catalytic reduction activity. Mater. Sci. Eng. C. 90, 57–66. doi:10.1016/j.msec.2018.04.044
Shaikh, S., Nazam, N., Rizvi, S. M. D., Ahmad, K., Baig, M. H., Lee, E. J., et al. (2019). Mechanistic insights into the antimicrobial actions of metallic nanoparticles and their implications for multidrug resistance. Int. J. Mol. Sci. 20 (10), 2468. doi:10.3390/ijms20102468
Shamaila, S., Zafar, N., Riaz, S., Sharif, R., Nazir, J., and Naseem, S. (2016). Gold nanoparticles: An efficient antimicrobial agent against enteric bacterial human pathogen. Nanomaterials 6 (4), 71. doi:10.3390/nano6040071
Shang, Y., Hasan, M., Ahammed, G. J., Li, M., Yin, H., and Zhou, J. (2019). Applications of nanotechnology in plant growth and crop protection: a review. Molecules 24 (14), 2558. doi:10.3390/molecules24142558
Shanmuganathan, R., Karuppusamy, I., Saravanan, M., Muthukumar, H., Ponnuchamy, K., Ramkumar, V. S., et al. (2019). Synthesis of silver nanoparticles and their biomedical applications - A comprehensive review. Curr. Pharm. Des. 25 (24), 2650–2660. doi:10.2174/1381612825666190708185506
Sharma, A., Goyal, A. K., and Rath, G. (2018). Recent advances in metal nanoparticles in cancer therapy. J. Drug Target. 26 (8), 617–632. doi:10.1080/1061186X.2017.1400553
Sharma, S. R., and Kar, D. (2019). “An Insight into plant nanobionics and its applications,” in Plant Nanobionics. Cham, Switzerland: Springer, 65–82.
Sheng, W. B., Li, W., Zhang, G. X., Tong, Y. B., Liu, Z. Y., and Jia, X. (2015). Study on the UV-shielding and controlled-release properties of a polydopamine coating for avermectin. New J. Chem. 39 (4), 2752–2757. doi:10.1039/C4NJ01744G
Shin, W. K., Cho, J., Kannan, A. G., Lee, Y. S., and Kim, D. W. (2016). Cross-linked composite gel polymer electrolyte using mesoporous methacrylate-functionalized SiO2 nanoparticles for lithium-ion polymer batteries. Sci. Rep. 6, 26332. doi:10.1038/srep26332
Shukla, P. K., Misra, P., and Kole, C. (2016). “Uptake, translocation, accumulation, transformation, and generational transmission of nanoparticles in plants,” in Plant Nanotechnology. Editors C. Kole, D. Kumar, and M. Khodakovskaya (Cham, Switzerland: Springer), 183–218. doi:10.1007/978-3-319-42154-4_8
Siddiqi, K. S., and Husen, A. (2017). Recent advances in plant-mediated engineered gold nanoparticles and their application in biological system. J. Trace Elem. Med. Biol. 40, 10–23. doi:10.1016/j.jtemb.2016.11.012
Silva, A. T., Nguyen, A., Ye, C., Verchot, J., and Moon, J. H. (2010). Conjugated polymer nanoparticles for effective siRNA delivery to tobacco BY-2 protoplasts. BMC Plant Biol. 10 (1), 291. doi:10.1186/1471-2229-10-291
Silva, L. P., Silveira, A. P., Bonatto, C. C., Reis, I. G., and Milreu, P. V. (2017). “Silver nanoparticles as antimicrobial agents: Past, present, and future,” in Nanostructures For Antimicrobial Therapy: Micro and Nano Technologies. Cham, Switzerland: Elsevier, 577–596. doi:10.1016/B978-0-323-46152-8.00026-3
Singh, A., Singh, N. Á., Afzal, S., Singh, T., and Hussain, I. (2018). Zinc oxide nanoparticles: a review of their biological synthesis, antimicrobial activity, uptake, translocation and biotransformation in plants. J. Mater. Sci. 53 (1), 185–201. doi:10.1007/s10853-017-1544-1
Singh, A., Singh, N. B., Hussain, I., Singh, H., and Singh, S. C. (2015). Plant-nanoparticle interaction: an approach to improve agricultural practices and plant productivity. Int. J. Pharm. Sci. Invent. 4 (8), 25–40.
Singh, J., Dutta, T., Kim, K. H., Rawat, M., Samddar, P., and Kumar, P. (2018). ‘Green’ synthesis of metals and their oxide nanoparticles: applications for environmental remediation. J. Nanobiotechnol. 16 (1), 84. doi:10.1186/s12951-018-0408-4
Singh, T., Jyoti, K., Patnaik, A., Singh, A., Chauhan, R., and Chandel, S. S. (2017). Biosynthesis, characterization and antibacterial activity of silver nanoparticles using an endophytic fungal supernatant of Raphanus sativus. J. Genet. Eng. Biotechnol. 15, 31–39. doi:10.1016/j.jgeb.2017.04.005
Singh, V. P., Sandeep, K., Kushwaha, H. S., Powar, S., and Vaish, R. (2018). Photocatalytic, hydrophobic and antimicrobial characteristics of ZnO nano needle embedded cement composites. Construc. Building Mat. 158, 285–294. doi:10.1016/j.conbuildmat.2017.10.035
Sirelkhatim, A., Mahmud, S., Seeni, A., Kaus, N. H. M., Ann, L. C., Bakhori, S. K. M., et al. (2015). Review on zinc oxide nanoparticles: antibacterial activity and toxicity mechanism. Nano-micro Lett. 7 (3), 219–242. doi:10.1007/s40820-015-0040-x
Slavin, Y. N., Asnis, J., Häfeli, U. O., and Bach, H. (2017). Metal nanoparticles: understanding the mechanisms behind antibacterial activity. J. Nanobiotechnol. 15 (1), 65. doi:10.1186/s12951-017-0308-z
Smetana, A. B., Klabunde, K. J., Marchin, G. R., and Sorensen, C. M. (2008). Biocidal activity of nanocrystalline silver powders and particles. Langmuir 24 (14), 7457–7464. doi:10.1021/la800091y
Soliman, H., Elsayed, A., and Dyaa, A. (2018). Antimicrobial activity of silver nanoparticles biosynthesised by Rhodotorula sp. strain ATL72. Egypt. J. Basic Appl. Sci. 5 (3), 228–233. doi:10.1016/j.ejbas.2018.05.005
Song, M. R., Cui, S. M., Gao, F., Liu, Y. R., Fan, C. L., Lei, T. Q., et al. (2012). Dispersible silica nanoparticles as carrier for enhanced bioactivity of chlorfenapyr. J. Pestic. Sci. 37 (3), D12–D027. doi:10.1584/jpestics.D12-027
Soo, J. Z., Chai, L. C., Ang, B. C., and Ong, B. H. (2020). Enhancing the antibacterial performance of titanium dioxide nanofibers by coating with silver nanoparticles. ACS Appl. Nano Mat. 3, 5743–5751.
Souza, R. C. D., Haberbeck, L. U., Riella, H. G., Ribeiro, D. H., and Carciofi, B. A. (2019). Antibacterial activity of zinc oxide nanoparticles synthesized by solochemical process. Braz. J. Chem. Eng. 36 (2), 885–893. doi:10.1590/0104-6632.20190362s20180027
Sticher, L., Mauch-Mani, B., and Métraux, A. J. (1997). Systemic acquired resistance. Ann. Rev. Phytopathol. 35 (1), 235–270. doi:10.1146/annurev.phyto.35.1.235
Subhapriya, S., and Gomathipriya, P. (2018). Green synthesis of titanium dioxide (TiO2) nanoparticles by Trigonella foenum-graecum extract and its antimicrobial properties. Microb. Pathog. 116, 215–220. doi:10.1016/j.micpath.2018.01.027
Surega, R., Anita, B., and Ramakrishnan, S. (2019). “Biological synthesis of nanoparticles and their antimicrobial properties,” in Advances in Agriculture. Editor R. K. Naresh (Delhi, India: AkiNik Publications), 19, 1–23. doi:10.22271/ed.book.421
Suresh, U., Murugan, K., Panneerselvam, C., Rajaganesh, R., Roni, M., Al-Aoh, H. A. N., et al. (2018). Suaeda maritima-based herbal coils and green nanoparticles as potential biopesticides against the dengue vector Aedes aegypti and the tobacco cutworm Spodoptera litura. Physiol. Mol. Plant Pathol. 101, 225–235. doi:10.1016/j.pmpp.2017.01.002
Syed, B., Prasad, M. N., and Satish, S. (2019). Synthesis and characterization of silver nanobactericides produced by Aneurinibacillus migulanus 141, a novel endophyte inhabiting Mimosa pudica L. Arab. J. Chem. 12 (8), 3743–3752. doi:10.1016/j.arabjc.2016.01.005
Syed, B., Prasad, N. M., and Satish, S. (2016). Endogenic mediated synthesis of gold nanoparticles bearing bactericidal activity. J. Microsc. Ultrastruct. 4, 162–166. doi:10.1016/j.jmau.2016.01.004
Szostak, K., Ostaszewski, P., Pulit-Prociak, J., and Banach, M. (2019). Bismuth oxide nanoparticles in drug delivery systems. Pharm. Chem. J. 53, 48–51. doi:10.1007/s11094-019-01954-9
Tai, C. Y., Tai, C. T., Chang, M. H., and Liu, H. S. (2007). Synthesis of magnesium hydroxide and oxide nanoparticles using a spinning disk reactor. Ind. Eng. Chem. Res. 46 (17), 5536–5541. doi:10.1021/ie060869b
Talekar, S., Ghodake, V., Ghotage, T., Rathod, P., Deshmukh, P., Nadar, S., et al. (2012). Novel magnetic cross-linked enzyme aggregates (magnetic CLEAs) of alpha amylase. Bioresour. Technol. 123, 542–547. doi:10.1016/j.biortech.2012.07.044
Tan, D., Yuan, P., Annabi-Bergaya, F., Dong, F., Liu, D., and He, H. (2015). A comparative study of tubular halloysite and platy kaolinite as carriers for the loading and release of the herbicide amitrole. Appl. Clay Sci. 114, 190–196. doi:10.1016/j.clay.2015.05.024
Tao, C. (2018). Antimicrobial activity and toxicity of gold nanoparticles: research progress, challenges and prospects. Lett. Appl. Microbiol. 67 (6), 537–543. doi:10.1111/lam.13082
Tarafdar, J. C., Raliya, R., Mahawar, H., and Rathore, I. (2014). Development of zinc nanofertilizer to enhance crop production in pearl millet (Pennisetum americanum). Agric. Res. 3 (3), 257–262. doi:10.1007/s40003-014-0113-y
Taran, M., Rad, M., and Alavi, M. (2017). Antibacterial activity of copper oxide (CuO) nanoparticles biosynthesized by Bacillus sp. FU4: Optimization of experiment design. Pharm. Sci. 23 (3), 198–206. doi:10.15171/PS.2017.30
Tayo, L. L. (2017). Stimuli-responsive nanocarriers for intracellular delivery. Biophys. Rev. 9, 931–940. doi:10.1007/s12551-017-0341-z
Thairu, M. W., Skidmore, I. H., Bansal, R., Nováková, E., Hansen, T. E., Li‐Byarlay, H., et al. (2017). Efficacy of RNA interference knockdown using aerosolized short interfering RNAs bound to nanoparticles in three diverse aphid species. Insect Mol. Biol. 26 (3), 356–368. doi:10.1111/imb.12301
Thakur, M., and Sohal, B. S. (2013). Role of elicitors in inducing resistance in plants against pathogen infection: a review. Int. Sch. Res. Notices 2013, 762412. doi:10.1155/2013/762412
Thanh, N. T., and Green, L. A. (2010). Functionalisation of nanoparticles for biomedical applications. Nano Today 5 (3), 213–230. doi:10.1016/j.nantod.2010.05.003
Tian, Q., Tang, M., Sun, Y., Zou, R., Chen, Z., Zhu, M., et al. (2011). Hydrophilic flower-like CuS superstructures as an efficient 980 nm laser-driven photothermal agent for ablation of cancer cells. Adv. Mater. 23, 3542–3547. doi:10.1002/adma.201101295
Tiwari, V., Mishra, N., Gadani, K., Solanki, P. S., Shah, N. A., and Tiwari, M. (2018). Mechanism of anti-bacterial activity of zinc oxide nanoparticle against carbapenem-resistant Acinetobacter baumannii. Front. Microbiol. 9, 1218. doi:10.3389/fmicb.2018.01218
Tomar, A., and Garg, G. (2013). Short review on application of gold nanoparticles. Glob. J. Pharmacol. 7 (1), 34–38. doi:10.5829/idosi.gjp.2013.7.1.66173
Torabi, S., Mansoorkhani, M. J. K., Majedi, A., and Motevalli, S. (2020). Synthesis, medical and photocatalyst applications of nano-Ag2O. J. Coord. Chem. 73 (13), 1861–1880. doi:10.1080/00958972.2020.1806252
Tormena, R. P. I., Rosa, E. V., Mota, B. D. F. O., Chaker, J. A., Fagg, C. W., Freire, D. O., et al. (2020). Evaluation of the antimicrobial activity of silver nanoparticles obtained by microwave-assisted green synthesis using Handroanthus impetiginosus (Mart. ex DC.) Mattos underbark extract. RSC Adv. 10 (35), 20676–20681. doi:10.1039/d0ra03240a
Torney, F., Trewyn, B. G., Lin, V. S. Y., and Wang, K. (2007). Mesoporous silica nanoparticles deliver DNA and chemicals into plants. Nat. Nanotechnol. 2 (5), 295–300. doi:10.1038/nnano.2007.108
Tripathi, S., Sonkar, S. K., and Sarkar, S. (2011). Growth stimulation of gram (Cicer arietinum) plant by water soluble carbon nanotubes. Nanoscale 3 (3), 1176–1181. doi:10.1039/C0NR00722F
Ulbrich, K., Hola, K., Subr, V., Bakandritsos, A., Tucek, J., and Zboril, R. (2016). Targeted drug delivery with polymers and magnetic nanoparticles: covalent and noncovalent approaches, release control, and clinical studies. Chem. Rev. 116 (9), 5338–5431. doi:10.1021/acs.chemrev.5b00589
Ullah, A. A., Kibria, A. F., Akter, M., Khan, M. N. I., Maksud, M. A., Jahan, R. A., et al. (2017). Synthesis of Mn3O4 nanoparticles via a facile gel formation route and study of their phase and structural transformation with distinct surface morphology upon heat treatment. J. Saudi Chem. Soc. 21 (7), 830–836. doi:10.1016/j.jscs.2017.03.008
Umair Hassan, M., Aamer, M., Umer Chattha, M., Haiying, T., Shahzad, B., Barbanti, L., et al. (2020). The critical role of zinc in plants facing the drought stress. Agriculture 10 (9), 396. doi:10.3390/agriculture10090396
UN DESA report (2015). World Population Prospects: the 2015 revision. United Nations Econ. Soc. Aff. 33 (2), 1–66. Available at: https://www.un.org/en/development/desa/publications/world-population-prospects-2015-revision.html.
Usman, M., Farooq, M., Wakeel, A., Nawaz, A., Cheema, S. A., ur Rehman, H., et al. (2020). Nanotechnology in agriculture: Current status, challenges and future opportunities. Sci. Total Environ. 721, 137778. doi:10.1016/j.scitotenv.2020.137778
Usman, M. S., El Zowalaty, M. E., Shameli, K., Zainuddin, N., Salama, M., and Ibrahim, N. A. (2013). Synthesis, characterization, and antimicrobial properties of copper nanoparticles. Int. J. Nanomedicine 8, 4467–4479. doi:10.2147/IJN.S50837
Vaishnav, J. K., and Mukherjee, T. K. (2019). Surfactant-induced self-assembly of CdTe quantum dots into multicolor luminescent hybrid vesicles. Langmuir 35 (19), 6409–6420. doi:10.1021/acs.langmuir.9b00357
Van Loon, L. C. (1997). Induced resistance in plants and the role of pathogenesis-related proteins. Eur. J. Plant Pathol. 103 (9), 753–765. doi:10.1023/A:1008638109140
Van, S. N., Minh, H. D., and Anh, D. N. (2013). Study on chitosan nanoparticles on biophysical characteristics and growth of Robusta coffee in green house. Biocat. Agric. Biotechnol. 2 (4), 289–294. doi:10.1016/j.bcab.2013.06.001
Varier, K. M., Gudeppu, M., Chinnasamy, A., Thangarajan, S., Balasubramanian, J., Li, Y., et al. (2019). “Nanoparticles: antimicrobial applications and its prospects,” in Advanced Nanostructured Materials For Environmental Remediation. Editors M. Naushad, S. Rajendran, and F. Gracia (Cham, Switzerland: Springer), 321–355. doi:10.1007/978-3-030-04477-0_12
Velusamy, P., Kumar, G. V., Jeyanthi, V., Das, J., and Pachaiappan, R. (2016). Bio-inspired green nanoparticles: Synthesis, mechanism, and antibacterial application. Toxicol. Res. 32 (2), 95–102. doi:10.5487/TR.2016.32.2.095
Venkatachalam, P., Priyanka, N., Manikandan, K., Ganeshbabu, I., Indiraarulselvi, P., Geetha, N., et al. (2017). Enhanced plant growth promoting role of phycomolecules coated zinc oxide nanoparticles with P supplementation in cotton (Gossypium hirsutum L.). Plant Physiol. Biochem. 110, 118–127. doi:10.1016/j.plaphy.2016.09.004
Verma, M., Kumar, V., and Katoch, A. (2018). Sputtering based synthesis of CuO nanoparticles and their structural, thermal and optical studies. Mater. Sci. Semicond. Process. 76, 55–60. doi:10.1016/j.mssp.2017.12.018
Verma, S. K., Gond, S. K., Mishra, A., Sharma, V. K., Kumar, J., Singh, D. K., et al. (2016). Biofabrication of antibacterial and antioxidant silver nanoparticles (AgNPs) by an endophytic fungus Pestalotia sp. isolated from Madhuca Longifolia. J. Nanomater. Mol. Nanotechnol. 5, 3. doi:10.4172/2324-8777.1000189
Wagner, G., Korenkov, V., Judy, J. D., and Bertsch, P. M. (2016). Nanoparticles composed of Zn and ZnO inhibit Peronospora tabacina spore germination in vitro and P. tabacina infectivity on tobacco leaves. Nanomaterials (Basel) 6 (3), 50. doi:10.3390/nano6030050
Wang, J. C., Neogi, P., and Forciniti, D. (2006). On one-dimensional self-assembly of surfactant-coated nanoparticles. J. Chem. Phys. 125 (19), 194717. doi:10.1063/1.2375091
Wang, L., Hu, C., and Shao, L. (2017). The antimicrobial activity of nanoparticles: present situation and prospects for the future. Int. J. Nanomed. 12, 1227–1249. doi:10.2147/IJN.S121956
Wang, X., Liu, X., Chen, J., Han, H., and Yuan, Z. (2014). Evaluation and mechanism of antifungal effects of carbon nanomaterials in controlling plant fungal pathogen. Carbon 68, 798–806. doi:10.1016/j.carbon.2013.11.072
Wang, X., Liu, X., and Han, H. Y. (2013). Evaluation of antibacterial effects of carbon nanomaterials against copper-resistant Ralstonia solanacearum. Colloids Surf. B. 103 (1), 136–142. doi:10.1016/j.colsurfb.2012.09.044
Wang, X., Liu, X., Han, H. Y., Gu, X. X., Chen, K., and Lu, D. L. (2012). Multi-walled carbon nanotubes can enhance root elongation of wheat (Triticum aestivum) plants. J. Nanopart. Res. 14 (6), 841–850. doi:10.1007/s11051-012-0841-5
Wang, X., Ma, X., Huang, P., Wang, J., Du, T., Du, X., et al. (2018). Magnetic Cu-MOFs embedded within graphene oxide nanocomposites for enhanced preconcentration of benzenoid-containing insecticides. Talanta 181, 112–117. doi:10.1016/j.talanta.2018.01.004
Wang, Y., Cui, H., Sun, C., Zhao, X., and Cui, B. (2014). Construction and evaluation of controlled-release delivery system of Abamectin using porous silica nanoparticles as carriers. Nanoscale Res. Lett. 9 (1), 655. doi:10.1186/1556-276X-9-655
Wang, Y., Li, C., Wang, Y., Zhang, Y., and Li, X. (2018). Compound pesticide controlled release system based on the mixture of poly (butylene succinate) and PLA. J. Microencapsul. 35 (5), 494–503. doi:10.1080/02652048.2018.1538265
Wang, Y., and Xia, Y. (2004). Bottom-up and top-down approaches to the synthesis of monodispersed spherical colloids of low melting-point metals. Nano Lett. 4 (10), 2047–2050. doi:10.1021/nl048689j
Wang, Y., Xu, C., Chang, Y., Zhao, L., Zhang, K., Zhao, Y., et al. (2017). Ultrasmall superparamagnetic iron oxide nanoparticle for T 2-weighted magnetic resonance imaging. ACS Appl. Mater. Inter. 9, 28959–28966. doi:10.1021/acsami.7b10030
Wanyika, H. (2013). “Sustained release of fungicide metalaxyl by mesoporous silica nanospheres,” in Nanotechnology for Sustainable Development. Editors M. S. Diallo, N. A. Fromer, and M. S. Jhon (Cham, Switzerland: Springer), 321–329. doi:10.1007/978-3-319-05041-6_25
Wen, L. X., Li, Z. Z., Zou, H. K., Liu, A. Q., and Chen, J. F. (2005). Controlled release of avermectin from porous hollow silica nanoparticles. Pest Manag. Sci. 61 (6), 583–590. doi:10.1002/ps.1032
Wibowo, D., Zhao, C. X., Peters, B. C., and Middelberg, A. P. (2014). Sustained release of fipronil insecticide in vitro and in vivo from biocompatible silica nanocapsules. J. Agric. Food Chem. 62 (52), 12504–12511. doi:10.1021/jf504455x
Wilson, M., Kannangara, K., Smith, G., Simmons, M., and Raguse, B. (2002). Nanotechnology: Basic science and emerging technologies. Boca Raton, FL: Chapman and Hall/CRC.
Wimmer, M. A., Abreu, I., Bell, R. W., Bienert, M. D., Brown, P. H., Dell, B., et al. (2019). Boron: an essential element for vascular plants. New Phytol. 226 (5), 1232–1237. doi:10.1111/nph.16127
Wongyai, K., Wintachai, P., Maungchang, R., and Rattanakit, P. (2020). Exploration of the antimicrobial and catalytic properties of gold nanoparticles greenly synthesized by Cryptolepis buchanani Roem. and Schult Extract. J. Nanomat. 2020, 1320274. doi:10.1155/2020/1320274
Worrall, E. A., Bravo-Cazar, A., Nilon, A. T., Fletcher, S. J., Robinson, K. E., Carr, J. P., et al. (2019). Exogenous application of RNAi-inducing double-stranded RNA inhibits aphid-mediated transmission of a plant virus. Front. Plant Sci. 10, 265. doi:10.3389/fpls.2019.00265
Worrall, E. A., Hamid, A., Mody, K. T., Mitter, N., and Pappu, H. R. (2018). Nanotechnology for plant disease management. Agronomy 8 (12), 285. doi:10.3390/agronomy8120285
Wu, M., Hou, P., Dong, L., Cai, L., Chen, Z., Zhao, M., et al. (2019). Manganese dioxide nanosheets: from preparation to biomedical applications. Int. J. Nanomed. 14, 4781–4800. doi:10.2147/IJN.S207666
Wu, R., Zhang, H., Pan, J., Zhu, H., Ma, Y., Cui, W., et al. (2016). Spatio‐design of multidimensional prickly Zn‐doped CuO nanoparticle for efficient bacterial killing. Adv. Mat. Inter. 3 (18), 1600472. doi:10.1002/admi.201600472
Wu, X., Ding, S. J., Lin, K., and Su, J. (2017). A review on the biocompatibility and potential applications of graphene in inducing cell differentiation and tissue regeneration. J. Mater. Chem. B. 5 (17), 3084–3102. doi:10.1039/C6TB03067J
Wu, Z. (2017). Nanocarbon-based photocatalysts: rGO/metal oxides composite membranes. RSC Adv. 4, 3823–3851. doi:10.1039/C3RA45013A
Xiao, T., Huang, J., Wang, D., Meng, T., and Yang, X. (2019). Au and Au-Based nanomaterials: synthesis and recent progress in electrochemical sensor applications. Talanta 206, 120210. doi:10.1016/j.talanta.2019.120210
Xiaowen, H., Saravankumar, K., Jin, T., and Myeong-Hyeon, W. (2019). Mycosynthesis, characterization, anticancer and antibacterial activity of silver nanoparticles from endophytic fungus Talaromyces purpureogenus. Int. J. Nanomedicine 14, 3427–3438. doi:10.2147/IJN.S200817
Xie, Y., He, Y., Irwin, P. L., Jin, T., and Shi, X. (2011). Antibacterial activity and mechanism of action of zinc oxide nanoparticles against Campylobacter jejuni. Appl. Environ. Microb. 77 (7), 2325–2331. doi:10.1128/AEM.02149-10
Xu, L., Cao, L. D., Li, F. M., Wang, X. J., and Huang, Q. L. (2014). Utilization of chitosan-lactide copolymer nanoparticles as controlled release pesticide carrier for pyraclostrobin against Colletotrichum gossypii Southw. J. Dispers. Sci. Technol. 35 (4), 544–550. doi:10.1080/01932691.2013.800455
Yadav, T. P., Yadav, R. M., and Singh, D. P. (2012). Mechanical milling: a top down approach for the synthesis of nanomaterials and nanocomposites. Nanosci. Nanotechnol. 2 (3), 22–48. doi:10.5923/j.nn.20120203.01
Yan, A., and Chen, Z. (2019). Impacts of silver nanoparticles on plants: a focus on the phytotoxicity and underlying mechanism. Int. J. Mol. Sci. 20 (5), 1003. doi:10.3390/ijms20051003
Yang, F. L., Li, X. G., Zhu, F., and Lei, C. L. (2009). Structural characterization of nanoparticles loaded with garlic essential oil and their insecticidal activity against Tribolium castaneum (Herbst)(Coleoptera: Tenebrionidae). J. Agric. Food Chem. 57 (21), 10156–10162. doi:10.1021/jf9023118
Yang, G., Phua, S. Z. F., Bindra, A. K., and Zhao, Y. (2019). Degradability and clearance of inorganic nanoparticles for biomedical applications. Adv. Mater. 31 (10), 1805730. doi:10.1002/adma.201805730
Yang, J., Cao, W., and Rui, Y. (2017). Interactions between nanoparticles and plants: phytotoxicity and defense mechanisms. J. Plant Interact. 12 (1), 158–169. doi:10.1080/17429145.2017.1310944
Yang, Y., Cheng, J., Garamus, V. M., Li, N., and Zou, A. (2018). Preparation of an environmentally friendly formulation of the insecticide nicotine hydrochloride through encapsulation in chitosan/tripolyphosphate nanoparticles. J. Agric. Food Chem. 66 (5), 1067–1074. doi:10.1021/acs.jafc.7b04147
Yaqoob, A. A., Ahmad, H., Parveen, T., Ahmad, A., Oves, M., Ismail, I. M., et al. (2020). Recent advances in metal decorated nanomaterials and their various biological applications: A review. Front. Chem. 8, 341. doi:10.3389/fchem.2020.00341
Yarema, M., Kovalenko, M. V., Hesser, G., Talapin, D. V., and Heiss, W. (2010). Highly monodisperse bismuth nanoparticles and their three-dimensional superlattices. J. Am. Chem. Soc. 132 (43), 15158–15159. doi:10.1021/ja107458s
Yew, Y. P., Shameli, K., Miyake, M., Khairudin, N. B. B. A., Mohamad, S. E. B., Naiki, T., et al. (2020). Green biosynthesis of superparamagnetic magnetite Fe3O4 nanoparticles and biomedical applications in targeted anticancer drug delivery system: A review. Arab. J. Chem. 13 (1), 2287–2308. doi:10.1016/j.arabjc.2018.04.013
Yu, S., Liu, J., Yin, Y., and Shen, M. (2018). Interactions between engineered nanoparticles and dissolved organic matter: a review on mechanisms and environmental effects. J. Environ. Sci. 63, 198–217. doi:10.1016/j.jes.2017.06.021
Zehra, A., Dubey, M. K., Tiwari, A., Meena, M., Kumari, P., Singh, V. K., et al. (2015). “Fungal biomolecules and their implications,” in Fungal Biomolecules: Source Applications And Recent Developments. Editors V. K. Gupta, R. L. Mach, and S. Sreenivasaprasad (Hoboken, NJ: Wiley Blackwell, John Wiley & Sons Ltd.), 363–375.
Zehra, A., Meena, M., Swapnil, P., Raytekar, N. A., and Upadhyay, R. S. (2020). “Sustainable approaches to remove heavy metals from water,” in Microbial Biotechnology: Basic Research And Applications. Editors J. Singh, A. Vyas, S. Wang, and R. Prasad (Singapore: Springer), 127–146. doi:10.1007/978-981-15-2817-0
Zhang, H., Qin, H., Li, L., Zhou, X., Wang, W., and Kan, C. (2017). Preparation and characterization of controlled-release avermectin/castor oil-based polyurethane nanoemulsions. J. Agric. Food Chem. 66 (26), 6552–6560. doi:10.1021/acs.jafc.7b01401
Zhang, H., Wang, Y., Zhu, X., Li, Y., and Cai, W. (2019). Bilayer Au nanoparticle-decorated WO3 porous thin films: On-chip fabrication and enhanced NO2 gas sensing performances with high selectivity. Sensor. Actuat. B: Chem. 280, 192–200. doi:10.1016/j.snb.2018.10.065
Zhang, J., Li, M., Fan, T., Xu, Q., Wu, Y., Chen, C., et al. (2013). Construction of novel amphiphilic chitosan copolymer nanoparticles for chlorpyrifos delivery. J. Polym. Res. 20 (3), 107. doi:10.1007/s10965-013-0107-7
Zhang, K., He, J., Liu, L., Xie, R., Qiu, L., Li, X., et al. (2020). A convenient, rapid and efficient method for establishing transgenic lines of Brassica napus. Plant Meth 16, 1–14. doi:10.1186/s13007-020-00585-6
Zhang, P., Ma, Y., Xie, C., Guo, Z., He, X., Valsami-Jones, E., et al. (2019). Plant species-dependent transformation and translocation of ceria nanoparticles. Environ. Sci. Nano 6 (1), 60–67. doi:10.1039/C8EN01089G
Zhang, R., Meng, Z., Abid, M. A., and Zhao, X. (2019). Novel pollen magnetofection system for transformation of Cotton plant with magnetic nanoparticles as gene carriers. in Trans. Cotton. New York, NY: Humana Press, 47–54.
Zhang, Y., Peng, H., Huang, W., Zhou, Y., and Yan, D. (2008). Facile preparation and characterization of highly antimicrobial colloid Ag or Au nanoparticles. J. Colloid Interf. Sci. 325 (2), 371–376. doi:10.1016/j.jcis.2008.05.063
Zhao, J., and Stenzel, M. H. (2018). Entry of nanoparticles into cells: The importance of nanoparticle properties. Polym. Chem. 9 (3), 259–272. doi:10.1039/C7PY01603D
Zhao, K., Li, D., Shi, C., Ma, X., Rong, G., Kang, H., et al. (2016). Biodegradable polymeric nanoparticles as the delivery carrier for drug. Curr. Drug Del. 13 (4), 494–499. doi:10.2174/156720181304160521004609
Zhao, M., Biswas, A., Hu, B., Joo, K., Wang, P., Gu, Z., et al. (2011). Redox-responsive nanocapsules for intracellular protein delivery. Biomaterials 32, 5223–5230. doi:10.1016/j.biomaterials.2011.03.060
Zhao, P., Cao, L., Ma, D., Zhou, Z., Huang, Q., and Pan, C. (2017). Synthesis of pyrimethanil-loaded mesoporous silica nanoparticles and its distribution and dissipation in cucumber plants. Molecules 22 (5), 817. doi:10.3390/molecules22050817
Zhao, P., Cao, L., Ma, D., Zhou, Z., Huang, Q., and Pan, C. (2018). Translocation, distribution and degradation of prochloraz-loaded mesoporous silica nanoparticles in cucumber plants. Nanoscale 10 (4), 1798–1806. doi:10.1039/C7NR08107C
Zhao, X., Meng, Z., Wang, Y., Chen, W., Sun, C., Cui, B., et al. (2017). Pollen magnetofection for genetic modification with magnetic nanoparticles as gene carriers. Nat. Plants 3 (12), 956–964. doi:10.1038/s41477-017-0063-z
Zhu, C., Ahmed, I., Parsons, A., Liu, J., and Liu, X. (2019). The mechanical property, degradation and cytocompatibility analysis of novel phosphate glass fiber textiles. Textile Res. J. 89, 3280–3290. doi:10.1177/0040517518809052
Zhu, C., Han, L., Hu, P., and Dong, S. (2012). In situ loading of well-dispersed gold nanoparticles on two-dimensional graphene oxide/SiO2 composite nanosheets and their catalytic properties. Nanoscale 4 (5), 1641–1646. doi:10.1039/C2NR11625A
Zhu, J., Zhou, Z., Yang, C., Kong, D., Wan, Y., and Wang, Z. (2011). Folate-conjugated amphiphilic starshaped block copolymers as targeted nanocarriers. J. Biomed. Mater. Res. A. 97A, 498–508. doi:10.1002/jbm.a.33071
Zienkiewicz-Strzałka, M., Deryło-Marczewska, A., Skorik, Y. A., Petrova, V. A., Choma, A., and Komaniecka, I. (2020). Silver nanoparticles on chitosan/silica nanofibers: Characterization and antibacterial activity. Int. J. Mol. Sci. 21 (1), 166. doi:10.3390/ijms21010166
Zorraquín-Peña, I., Cueva, C., Bartolomé, B., and Moreno-Arribas, M. (2020). Silver nanoparticles against foodborne bacteria. Effects at intestinal level and health limitations. Microorganisms 8 (1), 132. doi:10.3390/microorganisms8010132
Keywords: nanotechnology, nanoparticles, crop yield, genetic engineering, molecular approaches, gene carriers
Citation: Meena M, Zehra A, Swapnil P, Harish , Marwal A, Yadav G and Sonigra P (2021) Endophytic Nanotechnology: An Approach to Study Scope and Potential Applications. Front. Chem. 9:613343. doi: 10.3389/fchem.2021.613343
Received: 02 October 2020; Accepted: 18 January 2021;
Published: 25 May 2021.
Edited by:
Sougata Ghosh, RK University, IndiaReviewed by:
Pravin Patil, SVKM's Narsee Monjee Institute of Management Studies, IndiaRebecca Thombre, Pune University, India
Chetan Keswani, Banaras Hindu University, India
Umesh Pravin Dhuldhaj, Banaras Hindu University, India
Copyright © 2021 Meena, Zehra, Swapnil, Harish, Marwal, Yadav and Sonigra. This is an open-access article distributed under the terms of the Creative Commons Attribution License (CC BY). The use, distribution or reproduction in other forums is permitted, provided the original author(s) and the copyright owner(s) are credited and that the original publication in this journal is cited, in accordance with accepted academic practice. No use, distribution or reproduction is permitted which does not comply with these terms.
*Correspondence: Mukesh Meena, bXVrZXNobWVlbmFtbHN1QGdtYWlsLmNvbQ== Prashant Swapnil, bWJodXByYXNoYW50QGdtYWlsLmNvbQ== Andleeb Zehra, emFuZGxlZWIxNDNAZ21haWwuY29t
†ORCID: Mukesh Meena, orcid.org/0000-0002-6336-1140