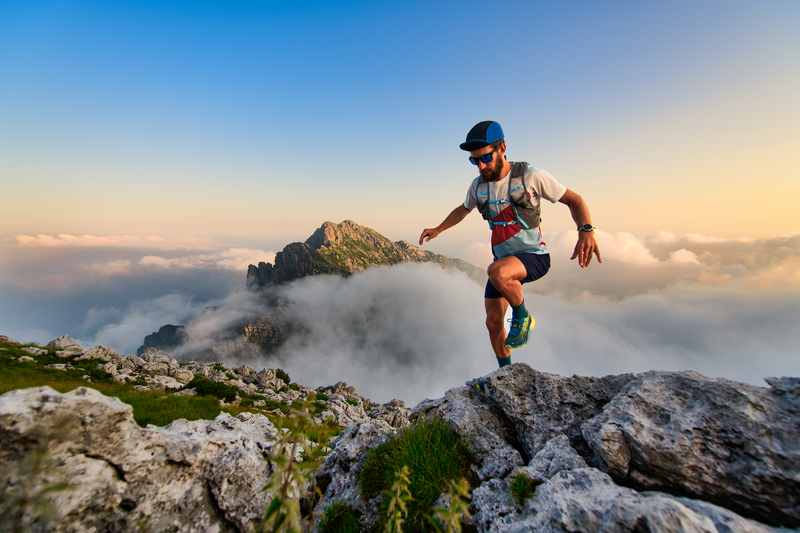
95% of researchers rate our articles as excellent or good
Learn more about the work of our research integrity team to safeguard the quality of each article we publish.
Find out more
MINI REVIEW article
Front. Chem. , 14 December 2020
Sec. Green and Sustainable Chemistry
Volume 8 - 2020 | https://doi.org/10.3389/fchem.2020.608236
This article is part of the Research Topic Advances in Analytical Techniques and Methodology for Chemical Speciation Study View all 16 articles
The decontamination of water containing toxic metals is a challenging problem, and in the last years many efforts have been undertaken to discover efficient, cost-effective, robust, and handy technology for the decontamination of downstream water without endangering human health. According to the World Health Organization (WHO), 180 million people in the world have been exposed to toxic levels of arsenic from potable water. To date, a variety of techniques has been developed to maintain the arsenic concentration in potable water below the limit recommended by WHO (10 μg/L). Recently, a series of technological advancements in water remediation has been obtained from the rapid development of nanotechnology-based strategies that provide a remarkable control over nanoparticle design, allowing the tailoring of their properties toward specific applications. Among the plethora of nanomaterials and nanostructures proposed in the remediation field, graphene-based materials (G), due to their unique physico-chemical properties, surface area, size, shape, ionic mobility, and mechanical flexibility, are proposed for the development of reliable tools for water decontamination treatments. Moreover, an emerging class of 3D carbon materials characterized by the intrinsic properties of G together with new interesting physicochemical properties, such as high porosity, low density, unique electrochemical performance, has been recently proposed for water decontamination. The main design criteria used to develop remediation nanotechnology-based strategies have been reviewed, and special attention has been reserved for the advances of magnetic G and for nanostructures employed in the fabrication of membrane filtration.
Arsenic is a ubiquitous element, present in all environmental compartments as well as in living organisms (Merian et al., 2004). It is a component of the earth's crust, minerals, and soils, and it is used as a wood preservative, a component of fertilizers and pesticides, in the mining, metallurgical, glass-making and semiconductor industries. Arsenic toxicity has become a public health problem and an environmental question. The World Health Organization (WHO) estimated that about 180 million people in 50 countries have been exposed to toxic arsenic levels (at least 10 μg/L in drinking water) (International Agency for Research on Cancer IARC, 2012). Arsenic is included among class I carcinogens (International Agency for Research on Cancer IARC, 2012); its toxicity and bioaccumulation greatly depend on its chemical state and on the metabolic pathways in which it is involved (Costa, 2019). Acute and chronic toxicity mechanisms are well-studied, whereas the mechanisms that underlie arsenic-mediated carcinogenesis, including epigenetic alterations, remain largely unknown (Costa, 2019; Nurchi et al., 2020). Arsenic is a metalloid, with four oxidation states (−3, 0, +3, +5), and it exists in a variety of inorganic and organic forms with different toxicity levels, depending on its speciation.
Speciation in aqueous solutions is mostly controlled by redox potential (Eh) and pH. Potential-pH diagrams (Brookins, 1988) show that arsenic in water exists mainly in trivalent or pentavalent form.
Under oxidizing conditions (high Eh values), the As (V) species prevail, and their distribution is related to their pH. In natural pH environments (i.e., 4 < pH < 8), As(V) is present as H2As and HAs(Cassone et al., 2018), and the presence of other metal cations must be considered in the natural waters (Nordstrom et al., 2014; Cardiano et al., 2018; Chillè et al., 2018; Giuffrè et al., 2020). Speciation studies performed in presence of Ca2+ and Mg2+ highlighted the fact that the distribution of As(V) is strongly influenced by the high concentration of these cations and, in sea water, As (V) is mainly present as CaAs (46.8%) and MgHAs (31.8%), while in fresh water, the main species are HAs and H2As (31% each), together with CaHAs (25.8%) (Chillè et al., 2018).
Under reducing conditions (low Eh values), arsenic mainly exists as As (III) and (Cassone et al., 2018) the oxoanions distribution is associated with the pH; up to pH ≈ 9, As (III) is present as arsenous acid H3AsO3, whereas its anion H2As represents the stable species for 9 < pH ≤ 11. As (III) can also interact with different classes of chelators (Cassone et al., 2019, 2020; Chillè et al., 2020a,b).
Recently, the techniques developed for arsenic removal, such as membrane filtration, coagulation, adsorption, ion exchange, have been implemented by nanotechnology-based strategies (Ungureanu et al., 2015; Siddiqui et al., 2019). Here, we discuss and summarize (Table 1) the literature on technological advancements in arsenic remediation using graphene-based materials.
Graphene-based materials (G) include numerous carbon nanomaterials with different morphology, size, shape, chemical surface, and physical-chemical properties (Georgakilas et al., 2012; Yang et al., 2016; Siddiqui and Chaudhry, 2018; Neri et al., 2019; Cordaro et al., 2020; Kokkinos et al., 2020). The G family includes several members such as graphene oxide (GO), reduced graphene oxide (RGO), and their derivatives (e.g., functionalized G and G nanocomposites). Native G showed many remarkable properties, but its poor processability together with the production difficulty on a large scale limited its practical use (Neri et al., 2015b). The development of new derivatives hosting additional functional groups is the main strategy for developing G for practical applications (Neri et al., 2015a). The modification/functionalization processes tune the intrinsic features and allow the assembly of G in various structures (Figure 1).
Figure 1. (A) Graphene-based materials (graphene, graphene oxide, reduced graphene oxide and engineered graphene). (B) Schematic representation of GO-iron nanohybrid (GFeN) and the proposed mechanism involved for arsenic removal. (C) Representative example of hierarchically porus 3D assembly (G-CNT-Fe 3D) and the related SEM image (D) “Reproduced with permission of (Vadahanambi et al., 2013), (Copyright 2020), American Chemical Society”.
GO and its composites, in the form of membranes, thin films, paper-like materials, have increasing use in water decontamination, due to their unique physicochemical features (Siddiqui and Chaudhry, 2018).
DFT calculations pointed out that pristine GO binds strongly to heavy metals, like As and Pb, with binding energy of −4.5 and −4.7 eV, respectively, and weakly to Hg (Panigrahi et al., 2018).
The capability of GO to adsorb As species is directly affected by GO oxidation, and its increase from 1.98 to 1.35 (C/O ratio) prompted the As (III) maximum adsorption capacity from 123 to 288 mg/g (Reynosa-Martínez et al., 2020).
The use of GO-based chromatographic stationary phases has allowed the simultaneous separation of different types of arsenic species, avoiding multiple analyses (Reid et al., 2020). Porous GO functionalized with hyperbranched polyethyleneimine (PEI-GO) was proposed for arsenic-selective solid phase extraction (SPE) column. PEI increased the sorption capacity by interacting with both As (III) and As (V) through complexation and electrostatic interactions, respectively (Ahmad et al., 2018).
GO-functionalized silica microspheres (GO@SiO2) was investigated for metal speciation analysis of two inorganic arsenicals (arsenite and arsenate) and two organic arsenicals (monomethyl arsenic MMA and dimethylarsenic DMA). No retention by the native GO@SiO2 column was observed for the tested arsenicals, that are anions around pH 6.0, as they may be electrostatically expelled. To improve their retaining behaviors, aromatic quaternary ammoniums were added to electrostatically attract arsenic anions. The separation performance of GO@SiO2 was compared with that of G@SiO2, showing a negligible difference in retention time and resolution, confirming no affinity of oxygenated groups on pristine GO to arsenic anions (Cheng et al., 2018; Zhao et al., 2018).
One of the main concerns related to the use of GO-based materials is the problem of recovery after adsorption, which was resolved using magneto-responsive GO (Hemmati et al., 2018). Iron compounds were reported to form cross-linking with the oxygen functionalities on the surface of carbon materials (Su et al., 2017a). The incorporation of magnetic nanoparticles in GO prevents the aggregation and eases the separation by using an external magnetic field. A comparative study highlighted the fact that As removal was more effective using Fe3O4-GO composite (M-GO) than Fe3O4-reduced GO composite (M-RGO), due to the difference in the amount of oxygenated functional groups (Yoon et al., 2016).
Magnetic nanoparticles decorated with β-cyclodextrins-functionalized GO (β-CDs-GO@Fe3O4 NPs) were proposed as scalable adsorbents of As (III)/As (V) for their excellent water dispersibility and magnetic properties due to the combination of the individual advantages of both materials (Kumar and Jiang, 2017). A nanocomposite based on chitosan and magnetic GO (CMGO) showed the best As (III) adsorption capacity (45 mg/g) at pH 7 (Sherlala et al., 2019).
To increase the water stability, magnetite and GO were encapsulated inside a non-toxic alginate bead (mGO/bead) and the adsorption of Cr (VI) and As (V) from multicomponent systems and contaminated wastewater was evaluated. mGO/bead showed excellent performance (80–100% removal) and recyclability in a complex mixture of heavy metals (Vu et al., 2017).
GO-iron nanohybrid (GFeN) systems were prepared by a sol-gel process for the concurrent removal of As(III)/As(V), without previous oxidation of As(III) to As(V) (Das et al., 2020). As(V) absorption involves electrostatic interactions as well as surface complexation with corrosion products, whereas only surface complexation leads the As(III) absorption. Adsorption capacity was high for both As (V) and As(III) species (Table 1) without iron leaching while it decreased in the presence of and ions. GO acts as a reservoir for the electrons released during the oxidation of Fe0, allowing the electrons to come back to Fe NPs (Figure 1B).
Magnetite partially reduced GO (Mag-PRGO) nanocomposite obtained via laser vaporization-controlled condensation method (Bobb et al., 2020) was exploited to remove As(V). Mag-PRGO showed the ability to remove 100% of As(V) up to 100 ppm final concentration (pH range 4–6), without the loss of iron ions in solution.
GO and ferrocene were used for the preparation of smart magnetic graphene (SMG) by a solvent-free microwave-induced process (Gollavelli et al., 2013). Upon irradiation, GO became graphene and ferrocene decomposed to metallic Fe core (~5 nm in size). SMG showed a maximum As(V) absorption capacity of 3.26 mg/g (Table 1), starting from an arsenic concentration of 5.0 ppm.
Adsorbent systems containing two or more metals or metal oxides were designed to improve arsenic adsorption performance. Graphene nanoplates (GNPs) supported with Fe-Mg binary oxide (La et al., 2017b) or spinel CuFe2O4 (La et al., 2017a) showed a significant As(V) adsorption. The better adsorption capacity was reached at low pH values, due to the protonation of OH, which attract As(V) oxyanions, whereas the decrease of net positive charge, at higher pH values, leads to a decrease of As(V) adsorption ability. Both systems showed a relevant selectivity toward arsenic anions compared to other ion species.
Fe@Cu&GO systems fabricated by coprecipitation of CuO and Fe3O4 on GO surface showed good values of absorption for both As(III)/As(V) (Table 1) with a competitive adsorption of phosphate ions (Wu et al., 2019). As(III) adsorption was independent from pH variation, whereas As(V) adsorption decreases under alkali conditions.
Considering the ability of Gadolinium (Gd) oxonium to form binary compounds with arsenic species and its sizeable magnetic moment, a Fe-GO-Gd system (Lingamdinne et al., 2020) was tested for As(V) adsorption. Both ion exchange surface complexation and electrostatic interactions allowed As(V) removal. The adsorption ability decreased in the presence of competitive ions (, , and ) and after four adsorption/desorption cycles, probably due to the leak of Fe and Gd ions from the GO surface.
Multifunctional magnetic graphene (MG@PDA@PGMA-AET), prepared by surface-initiated ICAR ATRP, was investigated for simultaneous adsorption and sequential elution of As(III) and As(V) (Wang et al., 2019). As(V) oxyanions were absorbed by electrostatic interactions by protonated functional groups of MG@PDA@PGMA-AET, conversely neutral H3AsO3 species were absorbed by chelation mechanism with –OH, –SH and –NH2 groups. The speciation analysis demonstrated a quantitative and simultaneous adsorption of both arsenic species (Table 1), using MG@PDA@PGMA-AET as column packing material.
The heterogeneous Fenton-like system (M-RGO) was proposed for the degradation of 4-aminophenylarsonic (p-ASA) and for the adsorption of arsenic species from wastewater (Wu et al., 2020). Removal rate of 89.8% for total As and 98.8% for p-ASA were estimated at neutral pH value.
Mn-Al-Fe RGO based hybrid system (MAF-RGO) was proposed to remove arsenic species by electro-sorption and reduction process (Penke et al., 2020). Relevant maximum sorption values for both As(III)/As(V) (Table 1) were estimated by the Freundlich model. Interestingly, the irradiation of MAF-RGO with white light (> 420 nm) increased two-fold the arsenic loading.
Macro/micro/meso porous structures guarantee an excellent permeation of gas and solution, promoting active interior sites. Moreover, 3D G systems are characterized by major mechanical stability avoiding the aggregation phenomenon typical of graphene layers (He et al., 2020). G-CNT-Fe 3D nanohybrid (Figure 1C), composed of CNTs vertically standing on G surface and FenOm NPs dispersed on CNT and G surfaces, (Vadahanambi et al., 2013) showed a higher performance to capture As(III) species compared to 2D iron-decorated G system. The high surface-to-volume ratio and the mesoporous morphology facilitated the molecular diffusion and the accessibility of iron oxides, which acted as arsenic interactions sites.
Mesoporous 3D G aerogels (GA) homogenously decorated with Fe3O4 NPs (Ye et al., 2015) showed a higher maximum adsorptive capacity (Table 1) compared with 2D Fe-G systems and porous Fe3O4. To keep the chemical structure of GO sheets and avoid the damage of oxygen groups, porous 3D magnetic GO hydrogel (MGOH) was prepared by generation of chemical bubbles mixing GO, Fe3O4 NPs, and polyacrylamide hydrochloride (PA) at room temperature (Liang et al., 2019). MGOH showed good maximum adsorption capacity values (Table 1) with one of the fastest adsorption speeds, reaching equilibrium within only 2 min for both As(III)/As(V) species.
Arsenic capture ability of engineered carbon nanospheres (CNs) with a mesopore/macropore structure depends on the amount of loaded FenOm (Su et al., 2017b). FenOm content of 7 and 13 wt% resulted in a maximum adsorption capacity of 246 and 416 mg/g for As(III), and 93 and 201 mg/g for As(V), respectively; at higher FenOm content a significant decrease in absorption capacity was observed, probably due to the formation of Fe oxide agglomerates that block the pores.
Nanofiltration membranes technology is a promising environment-friendly alternative to the conventional adsorbent materials or ion exchange resins (Shukla et al., 2018), providing the rejection of arsenic pollutants by low-cost filtration operations at low transmembrane pressure, through systems suitable to avoid fouling (i.e., cross-flow module) (Sen et al., 2010; Pal et al., 2014). Nanofiltration membranes suitability is mainly affected by the Donnan exclusion principle (Dresner, 1972; Bowen and Mukhtar, 1996; Jye and Ismail, 2017).
The structure and the porosity of polysulfone (PSU)-based membranes including GO can be tuned by exploiting PSU hydrophobicity and GO hydrophilicity (Rezaee et al., 2015; Shukla et al., 2018).
Pure PSU membrane exhibited a sponge-like system with a dense skin layer and a few pores with drop-like ends; the addition of 0.5 (w)% GO resulted in the formation of finger-like pores with closed ends. Further GO loading resulted in a drastic drop of the sponge-like structure, while the pores appeared open-ended and even bigger in size. The negatively charged surface was active in Donnan repulsion of negatively charged pollutants. With an increase in pH, the negative charge of the membrane surface increases, and the predominant arsenate species becomes the divalent ion (), enhancing the rejection performances (Rezaee et al., 2015). Although the rejection performance is negatively influenced by the contemporary presence of cations and anions, a higher efficiency of the PSU/carboxylated-GO membrane to reject mixed metal ions solutions than that of pure PPSU was evidenced (Shukla et al., 2018). PSU-based membranes containing GO were prepared also by interfacial polymerization (Pal et al., 2018b). The polyethersulfone (PES) membrane was covered with polyamide, and the residual acid groups belonging to the polyamide-matrix were used to bind a GO layer. This membrane was able to selectively remove ionic As(V) (Table 1), retaining useful metal ions of drinking water, without GO losses in the permeated stream. An economic industrial scale-up was also considered (Pal et al., 2018a).
A PAN-based electrospun composite containing GO and γ-Fe2O3 was developed by electrospinning of PAN in DMF with GO and γ-Fe2O3 (Tripathy and Hota, 2019). PAN-GO-γ-Fe2O3 membrane exhibited high affinity toward As(V) removal (36.1 mg/g) and the presence of anions such as chloride, nitrate, and sulfate do not affect the efficiency, whereas phosphate anions' copresence strongly decreases As(V) chemisorption. As(V) adsorption is proposed as an electrostatic attraction and surface complexation mechanism, operated by the -C-OH and Fe-O groups present on the membrane surface, able to form a complex with arsenate species H2As.
GO-manganese ferrite membranes (PES-GMF, from 0.5 to 2 wt% content) were prepared by dispersing GO-manganese ferrite (GMF) in a polymer mixed matrix of polyvinylpyrrolidone (PVP) and polyethersulfone (PES) that acted as a pore former and support, respectively. GO induced a pore size increase, although high GMF content prompted the agglomerates' formation due to dipole-dipole interactions. GMF increased the membrane hydrophilicity, and the addition of 2 w% of GMF resulted in an increased membrane water flux of 46% in the pure PES membrane. A pH-dependent efficiency was detected: in acidic conditions the electrostatic attraction prevails—positively charged GMF and As(V) in the form of H2As; in alkali conditions the electrostatic rejection occurs (due to the deprotonation of GMF and As(V) in form of HAs. The maximum As(V) adsorption capacity of 75.5 mg/g was found for the membrane loaded with 2% GMF (Shahrin et al., 2019).
The adsorptive processes based on electrostatic interactions are suitable only for As(V) species rejection whereas for As(III) removal the affinity of thiolated groups grafted onto engineered membranes was exploited.
A complex 3D porous membrane was synthesized by using GO, single-walled carbon nanotubes (SWCNT) and an antimicrobial PGLa peptide (Viraka Nellore et al., 2015) and tested for the removal of toxic As(III), As(V), Pb(II) and for the disinfection of pathogenic bacteria. Nanofiltration of multiple metal ions solution containing both As(III) and As(V) (10 ppm) and bacteria revealed that 96% of As(III) and 92% of As(V) were rejected from the membrane (Table 1). Although As(III) ions are difficult to remove through nanofiltration, they exhibit high affinity for thiolated groups of glutathione. As a confirmation of the binding affinity of thiolated proteins and As(III), an efficient GO-based membrane suitable for As(III) preconcentration in column phase processes was reported (Ahmad et al., 2020). GO and Bovine Serum albumin (BSA) solution was vacuum-filtered through a cellulose nitrate paper (0.22 μm) to obtain a self-standing polymer-laminated GO membrane (PLGO). BSA was physisorbed onto the GO sheets through electrostatic interactions inducing the formation of interlayer nano capillaries on the membrane surface. PLGO exhibited a maximum adsorption capacity of 140 mg/g, which is about three times higher than that of GO. The presence of other metal ions in solution slightly influences the As(III) selectivity. The recovery and reuse of the membrane do not affect the adsorption efficiency, confirming their usefulness for pre-concentration and speciation of As(III).
A Dispersive Micro-Solid Phase Extraction (DMSPE) membrane was developed by deposition of Al2O3/GO onto a membrane filter through a vacuum filtration procedure (Baranik et al., 2018). The membrane quantitatively binds As(V) deprotonated species (i.e. H2As and HAs) thanks to the high concentrations of surface hydroxylic groups with a pH-dependent performance.
The selected case studies showed the high potentiality of G nanotechnology to remove As from contaminated water. It is worth noting the ability of engineered graphene to effectively remove complex mixtures of organic and inorganic pollutants from water and its remarkable antimicrobial activity (Karahan et al., 2018).
Although some nanotechnological tools for water purification are already marketed (Khan and Malik, 2019), the use of G in water purification, in particular for As remediation, must be implemented to advance G nanotechnology from lab to the market. Specifically, major concerns such as safety, economic feasibility, and aggregation phenomena, especially in scaled up water purification systems, need to be reasonably addressed. To minimize the health risk, safety issues require a careful evaluation (Caccamo et al., 2020) with the implementation of in-vivo studies. The lack of standardized ways for G univocal characterization and the different fabrication methods make the replication of G published results difficult (Piperno et al., 2018). Characterization of G should be carried out by standardized ways to support the new laws for their regulation. REACH (Registration, Evaluation, Authorization, and Restriction of Chemicals) in the European Union is being updated for nanomaterial regulation. Finally, for commercial applications, G would need to be manufactured in standardized way and reduced cost considering that water scarcity is a serious problem in underdeveloped countries.
CF, PM, AN, AS, GN, and AP contributed to the design and writing of the mini-review. CF, PM, and AP supervision the work. AP funding acquisition. All authors contributed to the article and approved the submitted version.
Publication fees will be partially covered by Department Fund and by Frontiers discount DSC-07001469422PRD.
The authors declare that the research was conducted in the absence of any commercial or financial relationships that could be construed as a potential conflict of interest.
Ahmad, H., Huang, Z., Kanagaraj, P., and Liu, C. (2020). Separation and preconcentration of arsenite and other heavy metal ions using graphene oxide laminated with protein molecules. J. Hazard. Mater. 384:121479. doi: 10.1016/j.jhazmat.2019.121479
Ahmad, H., Umar, K., Ali, S. G., Singh, P., Islam, S. S., and Khan, H. M. (2018). Preconcentration and speciation of arsenic by using a graphene oxide nanoconstruct functionalized with a hyperbranched polyethyleneimine. Microchim. Acta 185:290. doi: 10.1007/s00604-018-2829-z
Baranik, A., Gagor, A., Queralt, I., Marguí, E., Sitko, R., and Zawisza, B. (2018). Determination and speciation of ultratrace arsenic and chromium species using aluminium oxide supported on graphene oxide. Talanta 185, 264–274. doi: 10.1016/j.talanta.2018.03.090
Bobb, J. A., Awad, F. S., Moussa, S., and El-Shall, M. S. (2020). Laser synthesis of magnetite-partially reduced graphene oxide nanocomposites for arsenate removal from water. J. Mater. Sci. 55, 5351–5363. doi: 10.1007/s10853-020-04363-6
Bowen, W. R., and Mukhtar, H. (1996). Characterisation and prediction of separation performance of nanofiltration membranes. J. Memb. Sci. 112, 263–274. doi: 10.1016/0376-7388(95)00302-9
Brookins, D. G. (1988). Eh-pH Diagrams for Geochemistry. Heidelberg: Springer-Verlag. doi: 10.1007/978-3-642-73093-1
Caccamo, D., Currò, M., Ientile, R., Verderio, E. A., Scala, A., Mazzaglia, A., et al. (2020). Intracellular fate and impact on gene expression of doxorubicin/cyclodextrin-graphene nanomaterials at sub-toxic concentration. Int. J. Mol. Sci. 21:4891. doi: 10.3390/ijms21144891
Cardiano, P., Chillè, D., Foti, C., and Giuffrè, O. (2018). Effect of the ionic strength and temperature on the arsenic(V) -Fe3+ and -Al3+ interactions in aqueous solution. Fluid Phase Equilib 458, 9–15. doi: 10.1016/j.fluid.2017.11.002
Cassone, G., Chillé, D., Foti, C., Giuffré, O., Ponterio, R. C., Sponer, J., et al. (2018). Stability of hydrolytic arsenic species in aqueous solutions: As3+vs. As5+. Phys. Chem. Chem. Phys. 20, 23272–23280.
Cassone, G., Chillè, D., Giacobello, F., Giuffrè, O., Mollica Nardo, V., Ponterio, R. C., et al. (2019). Interaction between As(III) and simple thioacids in water: an experimental and ab initio molecular dynamics investigation. J. Phys. Chem. B 123, 6090–6098. doi: 10.1021/acs.jpcb.9b04901
Cassone, G., Chillè, D., Mollica Nardo, V., Giuffrè, O., Ponterio, R. C., Sponer, J., et al. (2020). Arsenic–nucleotides interactions: an experimental and computational investigation. Dalton Trans. 49, 6302–6311. doi: 10.1039/D0DT00784F
Cheng, H., Zhang, W., Wang, Y., and Liu, J. (2018). Graphene oxide as a stationary phase for speciation of inorganic and organic species of mercury, arsenic and selenium using HPLC with ICP-MS detection. Microchim. Acta 185:425. doi: 10.1007/s00604-018-2960-x
Chillè, D., Aiello, D., Grasso, G. I., Giuffrè, O., Napoli, A., Sgarlata, C., et al. (2020a). Complexation of As(III) by phosphonate ligands in aqueous fluids: thermodynamic behavior, chemical binding forms and sequestering abilities. J. Environ. Sci. 94, 100–110. doi: 10.1016/j.jes.2020.03.056
Chillè, D., Cassone, G., Giacobello, F., Giuffrè, O., Nardo, V. M., Ponterio, R. C., et al. (2020b). Removal of As(III) from biological fluids: mono- versus dithiolic ligands. Chem. Res. Toxicol. 33, 967–974. doi: 10.1021/acs.chemrestox.9b00506
Chillè, D., Foti, C., and Giuffrè, O. (2018). Thermodynamic parameters for the protonation and the interaction of arsenate with Mg2+, Ca2+ and Sr2+: application to natural waters. Chemosphere 190, 72–79. doi: 10.1016/j.chemosphere.2017.09.115
Cordaro, A., Neri, G., Sciortino, M. T., Scala, A., and Piperno, A. (2020). Graphene-based strategies in liquid biopsy and in viral diseases diagnosis. Nanomaterials 10:1014. doi: 10.3390/nano10061014
Costa, M. (2019). Review of arsenic toxicity, speciation and polyadenylation of canonical histones. Toxicol. Appl. Pharmacol. 375, 1–4. doi: 10.1016/j.taap.2019.05.006
Das, T. K., Sakthivel, T. S., Jeyaranjan, A., Seal, S., and Bezbaruah, A. N. (2020). Ultra-high arsenic adsorption by graphene oxide iron nanohybrid: removal mechanisms and potential applications. Chemosphere 253:126702. doi: 10.1016/j.chemosphere.2020.126702
Dresner, L. (1972). Some remarks on the integration of the extended nernst-planck equations in the hyperfiltration of multicomponent solutions. Desalination 10, 27–46. doi: 10.1016/S0011-9164(00)80245-3
Georgakilas, V., Otyepka, M., Bourlinos, A. B., Chandra, V., Kim, N., Kemp, K. C., et al. (2012). Functionalization of graphene: covalent and non-covalent approaches, derivatives and applications. Chem. Rev. 112, 6156–6214. doi: 10.1021/cr3000412
Giuffrè, O., Aiello, D., Chillè, D., Napoli, A., and Foti, C. (2020). Binding ability of arsenate towards Cu2+ and Zn2+: thermodynamic behavior and simulation under natural water conditions. Environ. Sci. Process. Impacts 22, 1731–1742. doi: 10.1039/D0EM00136H
Gollavelli, G., Chang, C.-C., and Ling, Y.-C. (2013). Facile synthesis of smart magnetic graphene for safe drinking water: heavy metal removal and disinfection control. ACS Sustain. Chem. Eng. 1, 462–472. doi: 10.1021/sc300112z
He, J., Ni, F., Cui, A., Chen, X., Deng, S., Shen, F., et al. (2020). New insight into adsorption and co-adsorption of arsenic and tetracycline using a Y-immobilized graphene oxide-alginate hydrogel: adsorption behaviours and mechanisms. Sci. Tot. Environ. 701:134363. doi: 10.1016/j.scitotenv.2019.134363
Hemmati, M., Rajabi, M., and Asghari, A. (2018). Magnetic nanoparticle based solid-phase extraction of heavy metal ions: a review on recent advances. Microchim. Acta 185:160. doi: 10.1007/s00604-018-2670-4
International Agency for Research on Cancer IARC (2012). “Arsenic, metals, fibres and dusts”, in Monographs on the Evaluation of Carcinogenic Risks to Humans. (Lyon: International Agency for Research on Cancer IARC).
Jye, L. W., and Ismail, A. F. (2017). Nanofiltration Membranes: Synthesis, Characterization, and Applications. Boca Raton, FL: CRC Press, Taylor and Francis Group. doi: 10.1201/9781315181479
Karahan, H. E., Wiraja, C., Xu, C., Wei, J., Wang, Y., Wang, L., et al. (2018). Graphene materials in antimicrobial nanomedicine: current status and future perspectives. Adv. Healthc. Mater. 7:1701406. doi: 10.1002/adhm.201701406
Khan, S. T., and Malik, A. (2019). Engineered nanomaterials for water decontamination and purification: from lab to products. J. Hazard. Mater. 363, 295–308. doi: 10.1016/j.jhazmat.2018.09.091
Kokkinos, P., Mantzavinos, D., and Venieri, D. (2020). Current trends in the application of nanomaterials for the removal of emerging micropollutants and pathogens from water. Molecules 25:2016. doi: 10.3390/molecules25092016
Kumar, A. S. K., and Jiang, S.-J. (2017). Synthesis of magnetically separable and recyclable magnetic nanoparticles decorated with β-cyclodextrin functionalized graphene oxide an excellent adsorption of As(V)/(III). J. Mol. Liq. 237, 387–401. doi: 10.1016/j.molliq.2017.04.093
La, D. D., Nguyen, T. A., Jones, L. A., and Bhosale, S. V. (2017a). Graphene supported spinel CuFe2O4 composites: novel adsorbents for arsenic removal in aqueous media. Sensors 17:1292. doi: 10.3390/s17061292
La, D. D., Patwari, J. M., Jones, L. A., Antolasic, F., and Bhosale, S. V. (2017b). Fabrication of a GNP/Fe–Mg binary oxide composite for effective removal of arsenic from aqueous solution. ACS Omega 2, 218–226. doi: 10.1021/acsomega.6b00304
Liang, J., He, B., Li, P., Yu, J., Zhao, X., Wu, H., et al. (2019). Facile construction of 3D magnetic graphene oxide hydrogel via incorporating assembly and chemical bubble and its application in arsenic remediation. Chem. Eng. J. 358, 552–563. doi: 10.1016/j.cej.2018.09.213
Lingamdinne, L. P., Choi, J.-S., Choi, Y.-L., Chang, Y.-Y., Yang, J.-K., Karri, R. R., et al. (2020). Process modeling and optimization of an iron oxide immobilized graphene oxide gadolinium nanocomposite for arsenic adsorption. J. Mol. Liq. 299:112261. doi: 10.1016/j.molliq.2019.112261
Merian, E., Anke, M., Ihnat, M., and Stoeppler, M. (2004). Elements and Their Compounds in the Environment: Occurrence, Analysis and Biological Relevance. Weinheim: WILEY-VCH Verlag GMBH and Co. KGaA. doi: 10.1002/9783527619634
Neri, G., Fazio, E., Mineo, P. G., Scala, A., and Piperno, A. (2019). SERS sensing properties of new graphene/gold nanocomposite. Nanomaterials 9:1236. doi: 10.3390/nano9091236
Neri, G., Scala, A., Barreca, F., Fazio, E., Mineo, P. G., Mazzaglia, A., et al. (2015a). Engineering of carbon based nanomaterials by ring-opening reactions of a reactive azlactone graphene platform. Chem. Commun. 51, 4846–4849. doi: 10.1039/C5CC00518C
Neri, G., Scala, A., Fazio, E., Mineo, P. G., Rescifina, A., Piperno, A., et al. (2015b). Repurposing of oxazolone chemistry: gaining access to functionalized graphene nanosheets in a top-down approach from graphite. Chem. Sci. 6, 6961–6970. doi: 10.1039/C5SC02576A
Nordstrom, D. K., Majzlan, J., and Königsberger, E. (2014). Thermodynamic properties for arsenic minerals and aqueous species. Rev. Mineral. Geochem. 79, 217–255. doi: 10.2138/rmg.2014.79.4
Nurchi, V. M., Buha Djordjevic, A., Crisponi, G., Alexander, J., Bjørklund, G., and Aaseth, J. (2020). Arsenic toxicity: molecular targets and therapeutic agents. Biomolecules 10:235. doi: 10.3390/biom10020235
Pal, M., Chakrabortty, S., Nayak, J., and Pal, P. (2018a). Removing toxic contaminants from groundwater by graphene oxide nanocomposite in a membrane module under response surface optimization. Int. J. Environ. Sci. Technol. 16, 4583–4594. doi: 10.1007/s13762-018-1924-3
Pal, M., Mondal, M. K., Paine, T. K., and Pal, P. (2018b). Purifying arsenic and fluoride-contaminated water by a novel graphene-based nanocomposite membrane of enhanced selectivity and sustained flux. Environ. Sci. Pollut. Res. 25, 16579–16589. doi: 10.1007/s11356-018-1829-1
Pal, P., Chakrabortty, S., and Linnanen, L. (2014). A nanofiltration–coagulation integrated system for separation and stabilization of arsenic from groundwater. Sci. Tot. Environ. 476–477, 601–610. doi: 10.1016/j.scitotenv.2014.01.041
Panigrahi, P., Dhinakaran, A. K., Sekar, Y., Ahuja, R., and Hussain, T. (2018). Efficient adsorption characteristics of pristine and silver-doped graphene oxide towards contaminants: a potential membrane material for water purification? Chemphyschem 19, 2250–2257. doi: 10.1002/cphc.201800223
Penke, Y. K., Yadav, A. K., Sinha, P., Malik, I., Ramkumar, J., and Kar, K. K. (2020). Arsenic remediation onto redox and photo-catalytic/electrocatalytic Mn-Al-Fe impregnated rGO: sustainable aspects of sludge as supercapacitor. Chem. Eng. J. 390:124000. doi: 10.1016/j.cej.2019.124000
Piperno, A., Scala, A., Mazzaglia, A., Neri, G., Pennisi, R., Sciortino, M. T., et al. (2018). Cellular signaling pathways activated by functional graphene nanomaterials. Int. J. Mol. Sci. 19:3365. doi: 10.3390/ijms19113365
Reid, M. S., Hoy, K. S., Schofield, J. R. M., Uppal, J. S., Lin, Y., Lu, X., et al. (2020). Arsenic speciation analysis: a review with an emphasis on chromatographic separations. TrAC Trends Anal. Chem. 123:115770. doi: 10.1016/j.trac.2019.115770
Reynosa-Martínez, A. C., Tovar, G. N., Gallegos, W. R., Rodríguez-Meléndez, H., Torres-Cadena, R., Mondragón-Solórzano, G., et al. (2020). Effect of the degree of oxidation of graphene oxide on As(III) adsorption. J. Hazard. Mater. 384:121440. doi: 10.1016/j.jhazmat.2019.121440
Rezaee, R., Nasseri, S., Mahvi, A. H., Nabizadeh, R., Mousavi, S. A., Rashidi, A., et al. (2015). Fabrication and characterization of a polysulfone-graphene oxide nanocomposite membrane for arsenate rejection from water. J. Environ. Health Sci. Eng. 13:61. doi: 10.1186/s40201-015-0217-8
Sen, M., Manna, A., and Pal, P. (2010). Removal of arsenic from contaminated groundwater by membrane-integrated hybrid treatment system. J. Memb. Sci. 354, 108–113. doi: 10.1016/j.memsci.2010.02.063
Shahrin, S., Lau, W.-J., Goh, P.-S., Ismail, A. F., and Jaafar, J. (2019). Adsorptive mixed matrix membrane incorporating graphene oxide-manganese ferrite (GMF) hybrid nanomaterial for efficient As(V) ions removal. Composites B Eng. 175:107150. doi: 10.1016/j.compositesb.2019.107150
Sherlala, A. I. A., Raman, A. A. A., Bello, M. M., and Buthiyappan, A. (2019). Adsorption of arsenic using chitosan magnetic graphene oxide nanocomposite. J. Environ. Manage. 246, 547–556. doi: 10.1016/j.jenvman.2019.05.117
Shukla, A. K., Alam, J., Alhoshan, M., Arockiasamy Dass, L., Ali, F. A. A., Muthumareeswaran, M. R., et al. (2018). Removal of heavy metal ions using a carboxylated graphene oxide-incorporated polyphenylsulfone nanofiltration membrane. Environ. Sci. Water Res. Technol. 4, 438–448. doi: 10.1039/C7EW00506G
Siddiqui, S. I., and Chaudhry, S. A. (2018). A review on graphene oxide and its composites preparation and their use for the removal of As3+and As5+ from water under the effect of various parameters: application of isotherm, kinetic and thermodynamics. Process Saf. Environ. Protect. 119, 138–163. doi: 10.1016/j.psep.2018.07.020
Siddiqui, S. I., Naushad, M., and Chaudhry, S. A. (2019). Promising prospects of nanomaterials for arsenic water remediation: a comprehensive review. Process Saf. Environ. Protect. 126, 60–97. doi: 10.1016/j.psep.2019.03.037
Su, H., Ye, Z., and Hmidi, N. (2017a). High-performance iron oxide–graphene oxide nanocomposite adsorbents for arsenic removal. Colloids Surfaces A Physicochem. Eng. Aspects 522, 161–172. doi: 10.1016/j.colsurfa.2017.02.065
Su, H., Ye, Z., Hmidi, N., and Subramanian, R. (2017b). Carbon nanosphere–iron oxide nanocomposites as high-capacity adsorbents for arsenic removal. RSC Adv. 7, 36138–36148. doi: 10.1039/C7RA06187K
Tripathy, M., and Hota, G. (2019). Maghemite and graphene oxide embedded polyacrylonitrile electrospun nanofiber matrix for remediation of arsenate ions. ACS Appl. Polym. Mater. 2, 604–617. doi: 10.1021/acsapm.9b00982
Ungureanu, G., Santos, S., Boaventura, R., and Botelho, C. (2015). Arsenic and antimony in water and wastewater: overview of removal techniques with special reference to latest advances in adsorption. J. Environ. Manage. 151, 326–342. doi: 10.1016/j.jenvman.2014.12.051
Vadahanambi, S., Lee, S.-H., Kim, W.-J., and Oh, I.-K. (2013). Arsenic removal from contaminated water using three-dimensional graphene-carbon nanotube-iron oxide nanostructures. Environ. Sci. Technol. 47, 10510–10517. doi: 10.1021/es401389g
Viraka Nellore, B. P., Kanchanapally, R., Pedraza, F., Sinha, S. S., Pramanik, A., Hamme, A. T., et al. (2015). Bio-conjugated CNT-bridged 3D porous graphene oxide membrane for highly efficient disinfection of pathogenic bacteria and removal of toxic metals from water. ACS Appl. Mater. Interfaces 7, 19210–19218. doi: 10.1021/acsami.5b05012
Vu, H. C., Dwivedi, A. D., Le, T. T., Seo, S.-H., Kim, E.-J., and Chang, Y.-S. (2017). Magnetite graphene oxide encapsulated in alginate beads for enhanced adsorption of Cr(VI) and As(V) from aqueous solutions: role of crosslinking metal cations in pH control. Chem. Eng. J. 307, 220–229. doi: 10.1016/j.cej.2016.08.058
Wang, J., Zhang, W., Zheng, Y., Zhang, N., and Zhang, C. (2019). Multi-functionalization of magnetic graphene by surface-initiated ICAR ATRP mediated by polydopamine chemistry for adsorption and speciation of arsenic. Appl. Surf. Sci. 478, 15–25. doi: 10.1016/j.apsusc.2019.01.188
Wu, K., Jing, C., Zhang, J., Liu, T., Yang, S., and Wang, W. (2019). Magnetic Fe3O4@CuO nanocomposite assembled on graphene oxide sheets for the enhanced removal of arsenic(III/V) from water. Appl. Surf. Sci. 466, 746–756. doi: 10.1016/j.apsusc.2018.10.091
Wu, S., Yang, D., Zhou, Y., Zhou, H., Ai, S., Yang, Y., et al. (2020). Simultaneous degradation of p-arsanilic acid and inorganic arsenic removal using M-rGO/PS fenton-like system under neutral conditions. J. Hazard. Mater. 399:123032. doi: 10.1016/j.jhazmat.2020.123032
Yang, X., Xia, L., and Song, S. (2016). Arsenic adsorption from water using graphene-based materials: a critical review. Surface Rev. Lett. 24:1730001. doi: 10.1142/S0218625X17300015
Ye, Y., Yin, D., Wang, B., and Zhang, Q. (2015). Synthesis of three-dimensional Fe3O4-graphene aerogels for the removal of arsenic ions from water. J. Nanomater. 2015:864864. doi: 10.1155/2015/864864
Yoon, Y., Park, W. K., Hwang, T.-M., Yoon, D. H., Yang, W. S., and Kang, J.-W. (2016). Comparative evaluation of magnetite–graphene oxide and magnetite-reduced graphene oxide composite for As(III) and As(V) removal. J. Hazard. Mater. 304, 196–204. doi: 10.1016/j.jhazmat.2015.10.053
Keywords: arsenic, graphene, potable water, magnetic nanomaterials, nanofiltration membrane, nanoadsorbent, remediation
Citation: Foti C, Mineo PG, Nicosia A, Scala A, Neri G and Piperno A (2020) Recent Advances of Graphene-Based Strategies for Arsenic Remediation. Front. Chem. 8:608236. doi: 10.3389/fchem.2020.608236
Received: 21 September 2020; Accepted: 11 November 2020;
Published: 14 December 2020.
Edited by:
Carlos Rey-Castro, Universitat de Lleida, SpainReviewed by:
Václav Ranc, Palacký University, Olomouc, CzechiaCopyright © 2020 Foti, Mineo, Nicosia, Scala, Neri and Piperno. This is an open-access article distributed under the terms of the Creative Commons Attribution License (CC BY). The use, distribution or reproduction in other forums is permitted, provided the original author(s) and the copyright owner(s) are credited and that the original publication in this journal is cited, in accordance with accepted academic practice. No use, distribution or reproduction is permitted which does not comply with these terms.
*Correspondence: Placido Giuseppe Mineo, Z21pbmVvQHVuaWN0Lml0; Claudia Foti, Y2ZvdGlAdW5pbWUuaXQ=; Anna Piperno, YXBpcGVybm9AdW5pbWUuaXQ=
Disclaimer: All claims expressed in this article are solely those of the authors and do not necessarily represent those of their affiliated organizations, or those of the publisher, the editors and the reviewers. Any product that may be evaluated in this article or claim that may be made by its manufacturer is not guaranteed or endorsed by the publisher.
Research integrity at Frontiers
Learn more about the work of our research integrity team to safeguard the quality of each article we publish.