- 1Institute of Material, Sichuan University of Science and Engineering, Zigong, China
- 2Material Corrosion and Protection Key Laboratory of Sichuan Province, Zigong, China
- 3Langxingda Technology Co, Ltd., Zigong, China
Li2ZnTi2.9Cr0.1O8 and Li2ZnTi3O8 were synthesized by the liquid phase method and then studied comparatively using X-ray diffraction (XRD), scanning electron microscopy (SEM), X-ray photoelectron spectroscopy (XPS), galvanostatic charge–discharge testing, cyclic stability testing, rate performance testing, and electrochemical impedance spectroscopy (EIS). The results showed that Cr-doped Li2ZnTi3O8 exhibited much improved cycle performance and rate performance compared with Li2ZnTi3O8. Li2ZnTi2.9Cr0.1O8 exhibited a discharge ability of 156.7 and 107.5 mA h g−1 at current densities of 2 and 5 A g−1, respectively. In addition, even at a current density of 1 A g−1, a reversible capacity of 162.2 mA h g−1 was maintained after 200 cycles. The improved electrochemical properties of Li2ZnTi2.9Cr0.1O8 are due to its increased electrical conductivity.
Introduction
Lithium-ion batteries (LIBs) are a new type of rechargeable batteries that are characterized by high specific capacities and specific energies, small volumes, long life cycles, low cost, low energy consumption, low self-discharge efficiencies, small internal resistance, and high working voltages (Yoshio, 2001; Junmin et al., 2005; Dong-il and Han, 2006; Wang M. X. et al., 2019; Wang S. et al., 2019; Huang et al., 2020; Wang et al., 2020; Yi et al., 2020a), and they have wide applications, including in mobile phones, laptops, cameras, digital cameras, electric automobiles, energy storage, aerospace, and space exploration (Tarascon and Armand, 2001; Xiao et al., 2018, 2019; An et al., 2019; Hong et al., 2019). The selection of suitable anode materials for LIBs is extremely important for the performance of these batteries to have excellent life cycles and charge/discharge rate characteristics.
Recently, there have been numerous studies of anode materials, including LiTi2O4, Li2Ti3O7, Li2Ti6O13, Li4Ti5O12, Na2Li2Ti6O14, TiNb2O7, and Li2ZnTi3O8 (Tang et al., 2014a; Chen B. K. et al., 2015; Li G. H. et al., 2016; Liu et al., 2016; Li Z. F. et al., 2016; Yi et al., 2020b). Li2ZnTi3O8 with the cubic spinel structure has been considered as a promising material because of its lack of toxicity, low cost, relatively high theoretical capacity of 227 mA h g−1, and its low discharge voltage plateau of ~0.5 V (vs. Li/Li+) (Jović et al., 2009; Chen B. K. et al., 2015; Chen W. et al., 2015). However, compared with other anode materials, Li2ZnTi3O8 suffers from low electronic conductivity and an even worse rate performance, which means that its performance in practice is lower than the theoretical value (Tang and Tang, 2014; Chen B. K. et al., 2015). Hence, by increasing its electronic conductivity, its use can be extended to a wider range of applications.
Until now, this problem has been solved by applying a synthesized coating containing conductive species, nano-sized particles, and doping ions (Shenouda and Murali, 2008; Qi et al., 2009; Tian et al., 2010; Lin et al., 2011, 2013; Yu et al., 2011; Zhang et al., 2011; Bai et al., 2012; Jhan and Duh, 2012; Wu et al., 2013; Xu et al., 2013; Mani et al., 2014; Tang et al., 2014a; Yi et al., 2020c). As is widely known, by using a synthesized coating with conductive species on Li2ZnTi3O8, the transportation of electrons can be improved while decreasing the particle size, which further accelerates ionic transportation. Moreover, it has been shown that doping ions into the material can increase its internal electronic conductivity (Lee et al., 2010; Fang et al., 2013; Lin et al., 2014). According to previous studies, the introduction of Al (Tang et al., 2014b), Ag (Tang et al., 2014a), Mo (Wang M. X. et al., 2019), and Ti (III) (Chen et al., 2018) to Li2ZnTi3O8 greatly improved the electrochemical properties of these materials. For example, Tang et al. (2014b) partially replaced Ti with Al, and the resulting Li2ZnTi2.9Al0.1O8 composite showed a given capacity of 223.1 mA h g−1 at 0.1 A g−1 (Tang et al., 2014b). Wang M. X. et al. (2019) improved the electronic conductivity of the Li2ZnTi3O8 compound by introducing Mo, and Li2Zn0.93Mo0.07Ti3O8@graphene showed a high cycle capacity at high current densities of 5 A g−1, with a power rating of 153 mA h g−1 still being delivered on the 200th cycle (Wang M. X. et al., 2019). Recently, researchers have paid close attention to the use of Cr doping, which, according to one study (Ruan et al., 2017), can further enhance the electronic conductivity. Pan et al. (2018) have successfully prepared Cr-doped γ-Fe2O3/rGO cathode material by microwave method. The as-obtained 4.0 at% Cr-doped γ-Fe2O3/rGO sample exhibits the capacity of 1,060 mAh g−1 after 100 cycles at 100 mA g−1. Liu et al. (2018) have prepared Fe1.95Cr0.05F5·H2O material, which retains a discharge capacity of 171 mAh g−1 after 100 cycles at 0.1 C (1 C = 200 mAh g−1). Feng et al. (2009) have successfully synthesis Cr-LiV3O8 cathode material, and it showed an excellent electrochemical performance, with the retention of 94.4% after 100 cycles. To the best of our knowledge, the introduction of Cr3+-doped ions into Li2ZnTi3O8 has not yet been reported. In this paper, we describe the improvement in electrochemical performance of Li2ZnTi3O8 anode material as a result of Cr doping.
Materials and Methods
Li2ZnTi2.9Cr0.1O8 was prepared by the liquid phase method. Stoichiometric amounts of TiO2, (CH3COO)Li, (CH3COO)2Zn, and Cr(NO3)3 were mixed with anhydrous ethanol as solvent. The mixture was dried at 80°C for 2 h and then roasted in a muffle furnace at 700°C for 10 h to obtain the final Li2ZnTi2.9Cr0.1O8 compound. For comparison, Li2ZnTi3O8 was also synthesized without using Cr(NO3)3 as the raw material.
X-ray diffraction (XRD, Brook AXS's D2 PHASER) was used to examine the crystalline phase of both Li2ZnTi3O8 and Li2ZnTi2.9Cr0.1O8, which was recorded within the range of 10–70° (2θ). Scanning electron microscopy (SEM, TESCAN VEGA3) was used to examine the morphology of the samples. X-ray photoelectron spectroscopy (XPS) with Cr-Kα radiation was used to monitor the surface electronic states of the elements that were the sources of X-rays.
To construct the working electrodes, the as-prepared material, Super-P and LA-132 were mixed in weight ratios of 80:10:10 with water as solvent, and the prepared slurry was then pasted onto copper foil and dried at 100°C for 10 h in a vacuum oven. The working electrode, lithium metal, Celegard 2400 separator, and 1 M LiPF6 electrolyte solution containing a 1:1:1 mixture of ethylene carbonate (EC), dimethyl carbonate (DMC), and ethylmethyl carbonate (EMC) were used to prepare CR2032 coin cells in an Ar-filled glovebox. All electrochemical performances were tested in the voltage range of 0.05–3.0V at room temperature.
Results and Discussion
Figure 1 shows the XRD patterns of Li2ZnTi3O8 and Li2ZnTi2.9Cr0.1O8. It is clear from the figure that the diffraction peaks of the samples conform to the standard diffraction peaks of cubic spinel Li2ZnTi3O8, which demonstrates that the presence of Cr(NO3)3 does not obviously affect the structure of cubic spinel Li2ZnTi3O8. The crystal lattice constants calculated from the recorded XRD data are listed in Table 1, and the lattice parameters of Li2ZnTi3O8 and Li2ZnTi2.9Cr0.1O8 were estimated to be a = 8.4305 and 8.4340 Å, respectively. The lattice parameters increased following introduction of Cr3+ into the Li2ZnTi3O8 crystal structure due to the larger radius of Cr3+ (0.062 nm) compared with that of Ti4+ (0.061 nm) (Novikova et al., 2018), which promotes the transportation of lithium ions and further enhances the electrochemical performance (Tai et al., 2020).
Additional information about the surface electronic states of the elements was gained from XPS measurements. Figure 2A shows the XPS survey spectra of Li2ZnTi2.9Cr0.1O8. As can be seen from the figure, the peaks of Li 1s, C 1s, O 1s, Ti 2p, Zn 2p, and Cr 2p are clearly visible. Figure 2B reveals that two peaks centered at approximately 576.36 and 585.60 eV correspond well to the Cr 2p3/2 and Cr 2p1/2 peaks, demonstrating that Cr is present as Cr3+ ions in the as-synthesized Li2ZnTi2.9Cr0.1O8. The two peaks shown in Figure 2C centered at approximately 458.4 and 464.40 eV are detected for Li2ZnTi3O8 and Li2ZnTi2.9Cr0.1O8, which can correspond to the peaks of Ti (IV) 2p3/2 and Ti (IV) 2p1/2.
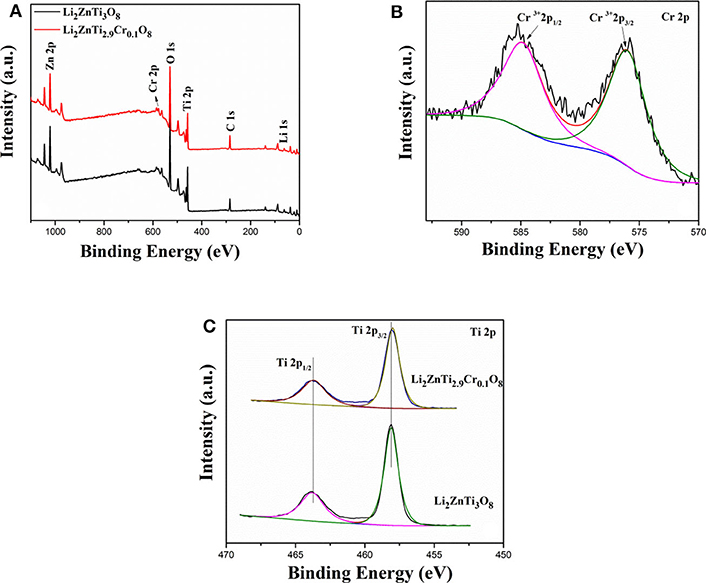
Figure 2. XPS spectra of (A) XPS survey spectrum, (B) Cr 2p, (C) Ti 2p of Li2ZnTi3O8 and Li2ZnTi2.9Cr0.1O8.
Figure 3 shows the SEM images of the Li2ZnTi3O8 and Li2ZnTi2.9Cr0.1O8 samples. It can be clearly seen that both samples are well-crystallized, with a small grain size distribution. It should be noted that the morphology of the particles did not appreciably change following doping with minute amounts of Cr3+. While increasing the contact area between active particles and the electrolyte, a good dispersion can narrow the transmission distance between Li+ and the electrons and increase the high-rate performance of 5C.
The initial charge/discharge curves of Li2ZnTi3O8 and Li2ZnTi2.9Cr0.1O8 are shown in Figure 4, which shows that the charge/discharge curves of Li2ZnTi2.9Cr0.1O8 are similar to those of Li2ZnTi3O8, suggesting that Cr3+ doping exerts an effect on the electrochemical reaction. The specific capacities of Li2ZnTi3O8 and Li2ZnTi2.9Cr0.1O8 at rates of 1 A g−1 after the first cycle were 130.5 and 166.8 mA h g−1, respectively. As can clearly be seen, Li2ZnTi2.9Cr0.1O8 demonstrates a higher specific capacity than Li2ZnTi3O8. This can be explained by the fact that Cr doping can enlarge the transport tunnel of lithium ions, which further increases lithium ion transportation and electron transfer, thus enhancing the electronic conductivity (Zhang et al., 2018). In addition, Li2ZnTi2.9Cr0.1O8 appears to have the least voltage platform difference, indicating that a lower electrode polarization was obtained following Cr3+ doping and Li+ transport was improved.
The rate performances of Li2ZnTi3O8 and Li2ZnTi2.9Cr0.1O8 are compared in Figure 5. Li2ZnTi2.9Cr0.1O8 delivered maximum discharge capacities of 221.7, 210.3, 185.1, 166, 156.7, and 107.5 mA h g−1 at 0.1, 0.2, 0.5, 1, 2, and 5 C, respectively, whereas Li2ZnTi3O8 delivered 193.4, 170.2, 147.8, 131, 98.3, and 43.5 mA h g−1 at the same rates, respectively. As can be seen, Li2ZnTi2.9Cr0.1O8 showed better rate performance compared with Li2ZnTi3O8, which may be due to either or both of the following reasons: (1) Cr doping improves the electronic conductivity, which leads to Li2ZnTi2.9Cr0.1O8 demonstrating better electrochemical performance than Li2ZnTi3O8; (2) the improved cell volume following Cr doping enhances lithium ion diffusion and electron transfer (Chen et al., 2009; Nie et al., 2020).
The cycling performance of Li2ZnTi3O8 and Li2ZnTi2.9Cr0.1O8 was examined at a rate of 1 A g−1, and the results are shown in Figure 6. As seen, the coulombic efficiency of Li2ZnTi3O8 and Li2ZnTi2.9Cr0.1O8 were 80.2 and 84.8% in the first cycle, indicating that the coulombic efficiency can be enhanced after Cr doped. After several cycles, the coulombic efficiency of two samples is close to 100%. After 200 cycles, the reserving ratios were 90.2 and 97.2% for Li2ZnTi3O8 and Li2ZnTi2.9Cr0.1O8, respectively. Therefore, Li2ZnTi2.9Cr0.1O8 possesses the better cycling performance. These results indicate that Li2ZnTi2.9Cr0.1O8 shows better capacity retention than Li2ZnTi3O8, which can probably be explained by assuming that moderate Cr doping can enlarge the transport tunnel of lithium ions, which further increases lithium ion transportation and electron transfer.
Electrochemical impedance spectroscopy (EIS) measurements for Li2ZnTi3O8 and Li2ZnTi2.9Cr0.1O8 were carried out to probe the kinetic properties of these anode materials, and the results are shown in Figure 7. Similar patterns are displayed by the impedance spectra of the two samples, which formed a semicircle in the high-frequency regions and a straight line in the low-frequency regions. The semicircle in the high-frequency regions is associated with charge transfer resistance on the electrode/electrolyte interface, while the straight line in the low-frequency regions is ascribed to the diffusion of Li+ into the Warburg resistance (Long et al., 2011; Tang et al., 2019), which constitutes the bulk of the electrode materials. It is clear that the charge transfer resistance of the Li2ZnTi2.9Cr0.1O8 electrode material is lower than that of Li2ZnTi3O8, showing that a certain small amount of Cr3+ doping of Li2ZnTi3O8 is useful for enhancing the electronic conductivity (Li Z. F. et al., 2016; Kou et al., 2020). In addition, the slope for the Li2ZnTi2.9Cr0.1O8 electrode material in the low-frequency regions is slightly higher than that for Li2ZnTi3O8 because Cr doping can strengthen lithium ion migration through Li2ZnTi3O8.
Conclusions
This study successfully prepared Li2ZnTi3O8 and Li2ZnTi2.9Cr0.1O8 samples with the cubic spinel structure by the sol-gel method. XRD revealed that the element Cr successfully doped into the Li2ZnTi3O8 exerted no effect on the spinel structure of Li2ZnTi3O8. SEM (Chen B. K. et al., 2015) results demonstrated that the morphology of the particles did not obviously change following Cr3+ doping. The measured electrochemical properties indicated that Li2ZnTi2.9Cr0.1O8 shows a better rate performance and excellent cyclic reversibility than Li2ZnTi3O8. The electrochemical performance might be significantly enhanced as a result of the higher electronic conductivity following Cr3+ doping, demonstrating that Li2ZnTi2.9Cr0.1O8 is a promising anode material for high-rate LIBs.
Data Availability Statement
All datasets presented in this study are included in the article/supplementary material.
Author Contributions
XZ and JP contributed conception and design of the study. HZ and YG organized the database. JP and XH wrote the first draft of the manuscript. XZ revised the whole manuscript. All authors contributed to the article and approved the submitted version.
Funding
This work was financially supported by Key Projects of Zigong Science and Technology Bureau (2018CXJD07).
Conflict of Interest
HZ was employed by the company Langxingda Technology Co, Ltd.
The remaining authors declare that the research was conducted in the absence of any commercial or financial relationships that could be construed as a potential conflict of interest.
References
An, F. Q., Zhao, H. L., Cheng, Z., Qiu, J. Y. C., Zhou, W. N., and Li, P. (2019). Development status and research progress of power battery for pure electric vehicles. Chin. J. Eng. 1, 22–42. doi: 10.13374/j.issn2095-9389.2019.01.003
Bai, Y. J., Gong, C., Qi, Y. X., Lun, N., and Feng, J. (2012). Excellent long-term cycling stability of La-doped Li4Ti5O12 anode material at high current rate. J. Mater. Chem. 22, 19054–19060. doi: 10.1039/c2jm34523d
Chen, B. K., Du, C. J., Zhang, Y. Z., Sun, R. X., Zhou, L., and Wang, L. J. (2015). A new strategy for synthesis of lithium zinc titanate as an anode material for lithium ion batteries. Electrochim. Acta 159, 102–110. doi: 10.1016/j.electacta.2015.01.206
Chen, C., Ai, C. C., and Liu, X. Y. (2018). Ti(III) self-doped Li2ZnTi3O8 as a superior anode material for Li-ion batteries. Electrochim. Acta 265, 448–454. doi: 10.1016/j.electacta.2018.01.159
Chen, W., Zhou, Z. R., Wang, R. R., Wu, Z. T., Liang, H. F., Shao, L. Y., et al. (2015). High performance Na-doped lithium zinc titanate as anode material for Li-ion batteries. RSC Adv. 5, 49890–49898. doi: 10.1039/C5RA06365E
Chen, Y. H., Zhao, Y. M., An, X. N., Liu, J. M., Dong, Y. Z., and Chen, L. (2009). Preparation and electrochemical performance studies on Cr-doped Li3V2(PO4)3 as cathode materials for lithium-ion batteries. Electrochim. Acta 54, 5844–5850. doi: 10.1016/j.electacta.2009.05.041
Dong-il, R., and Han, K. S. (2006). Used lithium ion rechargeable battery recycling using Etoile-Rebatt technology. J. Power Sources 163, 284–288. doi: 10.1016/j.jpowsour.2006.05.040
Fang, W., Cheng, X. Q., Zuo, P. J., Ma, Y. L., and Yin, G. P. (2013). A facile strategy to prepare nano-crystalline Li4Ti5O12/C anode material via polyvinyl alcohol as carbon source for high-rate rechargeable Li-ion batteries. Electrochim. Acta 93, 173–178. doi: 10.1016/j.electacta.2013.01.112
Feng, Y., Li, Y. L., and Hou, F. (2009). Preparation and electrochemical properties of Cr doped LiV3O8 cathode for lithium ion batteries. Mater. Lett. 63, 1338–1340. doi: 10.1016/j.matlet.2009.03.009
Hong, X. D., Huang, X. B., Ren, Y. R., Wang, H. Y., Ding, X., and Jin, J. L. (2019). Superior Na-storage performance of Na3V2(PO4)3/C-Ag composites as cathode material for Na-ion battery. J. Alloys Compd. 822:153587. doi: 10.1016/j.jallcom.2019.153587
Huang, X. B., Yin, X., Yang, Q., Guo, Z. H., Ren, Y. R., and Zeng, X. G. (2020). Outstanding electrochemical performance of N/S co-doped carbon/Na3V2(PO4)3 hybrid as the cathode of a sodium-ion battery. Ceramics Int. 46, 28084–28090. doi: 10.1016/j.ceramint.2020.07.303
Jhan, Y. R., and Duh, J. G. (2012). Electrochemical performance and low discharge cut-off voltage behavior of ruthenium doped Li4Ti5O12 with improved energy density. Electrochim. Acta 63, 9–15. doi: 10.1016/j.electacta.2011.12.014
Jović, N., Vučinić-Vasić, M., Kremenović, A., Antić, B., Jovalekić, C., Vulić, P., et al. (2009). HEBM synthesis of nanocrystalline LiZn0.5Ti1.5O4 spinel and thermally induced order-disorder phase transition. Mater. Chem. Phys. 116, 542–549. doi: 10.1016/j.matchemphys.2009.04.033
Junmin, N., Han, D., and Zuo, X. (2005). Recovery of metal values from spent lithium-ion batteries with chemical deposition and solvent extraction. J. Power Sources 152, 278–284. doi: 10.1016/j.jpowsour.2005.03.134
Kou, Z. Y., Miao, C., Mei, P., Zhang, Y., Yan, X. M., Jiang, Y., et al. (2020). Enhancing the cycling stability of all-solid-state lithium-ion batteries assembled with Li1.3Al0.3Ti1.7(PO4)3 solid electrolytes prepared from precursor solutions with appropriate pH values. Ceramics Int. 46, 9629–9636. doi: 10.1016/j.ceramint.2019.12.229
Lee, J. S., Wang, X. Q., Luo, H. M., and Dai, S. (2010). Fluidic carbon precursors for formation of functional carbon under ambient pressure based on ionic liquids. Adv. Mater. 22, 1004–1007. doi: 10.1002/adma.200903403
Li, G. H., Li, F. C., Yang, H., Cheng, F. Y., Xu, N., Shi, W., et al. (2016). Graphene oxides doped MIL-101(Cr) as anode materials for enhanced electrochemistry performance of lithium ion battery. Inorg. Chem. Commun. 64, 63–66. doi: 10.1016/j.inoche.2015.12.017
Li, Z. F., Cui, Y. H., Wu, J. W., Du, C. Q., Zhang, X. H., and Tang, Z. Y. (2016). Synthesis and electrochemical properties of lithium zinc titanate as an anode material for lithium ion batteries via microwave method. RSC Adv. 45, 39209–39215. doi: 10.1039/C6RA05244D
Lin, C. F., Lai, M. O., Zhou, H. H., and Lu, L. (2014). Mesoporous Li4Ti5O12−x/C submicrospheres with comprehensively improved electrochemical performances for high-power lithium-ion batteries. Phys. Chem. Chem. Phys. 16, 24874–24883. doi: 10.1039/C4CP03826F
Lin, J. Y., Hsu, C. C., Ho, H. P., and Wu, S. H. (2013). Sol-gel synthesis of aluminum doped lithium titanate anode material for lithium ion batteries. Electrochim. Acta 87, 126–132. doi: 10.1016/j.electacta.2012.08.128
Lin, Y., Jhan, Y. R., and Duh, J. G. (2011). Improved capacity and rate capability of Ru-doped and carbon-coated Li4Ti5O12 anode material. J. Alloys Compd. 509, 6965–6968. doi: 10.1016/j.jallcom.2011.04.018
Liu, M., Wang, X. Y., Wei, S. Y., Hu, H., Zhang, R., and Liu, L. (2018). Cr-doped Fe2F5·H2O with open framework structure as a high performance cathode material of sodium-ion batteries. Electrochim. Acta 269, 479–489. doi: 10.1016/j.electacta.2018.02.159
Liu, T., Tang, H. Q., Zan, L. X., and Tang, Z. Y. (2016). Comparative study of Li2ZnTi3O8 anode material with good high rate capacities prepared by solid state, molten salt and sol-gel methods. J. Electroanal. Chem. 771, 10–16. doi: 10.1016/j.jelechem.2016.03.036
Long, W. M., Wang, X. Y., Yang, S. Y., Shu, H. B., Wu, Q., Bai, Y. S., et al. (2011). Electrochemical properties of Li4Ti5−2xNixMnxO12 compounds synthesized by sol-gel process. Mater. Chem. Phys. 131, 431–435. doi: 10.1016/j.matchemphys.2011.09.071
Mani, V., Nathiya, K., and Kalaiselvi, N. (2014). Role of carbon content in qualifying Li3V2(PO4)3/C as a high capacity anode for high rate lithium battery applications. RSC Adv. 4, 17091–17096. doi: 10.1039/C4RA00963K
Nie, Y., Xiao, W., Miao, C., Fang, R., Kou, Z. Y., Wang, D., et al. (2020). Boosting the electrochemical performance of LiNi0.8Co0.15Al0.05O2 cathode materials in-situ modified with Li1.3Al0.3Ti1.7(PO4)3 fast ion conductor for lithium-ion batteries. Electrochim. Acta 353:136477. doi: 10.1016/j.electacta.2020.136477
Novikova, S. A., Larkovich, R. V., Chekannikov, A. A., Kulova, T. L., and Yaroslavtsev, A. B. (2018). Electrical conductivity and electrochemical characteristics of Na3V2(PO4)3-based NASICON-type materials. Inorg. Mater. 54, 794–804. doi: 10.1134/S0020168518080149
Pan, X., Duan, X. C., Lin, X. P., Zong, F. Y., Tong, X. B., Li, Q. H., et al. (2018). Rapid synthesis of Cr-doped gamma-Fe2O3/reduced graphene oxide nanocomposites as high performance anode materials for lithium ion batteries. J. Alloys Compd. 732, 270–279. doi: 10.1016/j.jallcom.2017.10.222
Qi, Y. L., Huang, Y. D., Jia, D. Z., Bao, S. J., and Guo, Z. P. (2009). Preparation and characterization of novel spinel Li4Ti5O12−xBrx anode materials. Electrochim. Acta 54, 4772–4776. doi: 10.1016/j.electacta.2009.04.010
Ruan, Y. L., Wang, K., Song, S. D., Liu, J. J., and Han, X. (2017). Improved structural stability and electrochemical performance of Na3V2(PO4)3 cathode material by Cr doping. Ionics 23, 1097–1105. doi: 10.1007/s11581-016-1919-3
Shenouda, Y., and Murali, K. R. (2008). Electrochemical properties of doped lithium titanate compounds and their performance in lithium rechargeable batteries. J. Power Sources 176, 332–339. doi: 10.1016/j.jpowsour.2007.10.061
Tai, Z. G., Zhu, W., Shi, M., Xin, Y. F., Guo, S. W., Wu, Y. F., et al. (2020). Improving electrochemical performances of Lithium-rich oxide by cooperatively doping Cr and coating Li3PO4 as cathode material for Lithium-ion batteries. J. Colloid Interface Sci. 576, 468–475. doi: 10.1016/j.jcis.2020.05.015
Tang, H. Q., and Tang, Z. Y. (2014). Effect of different carbon sources on electrochemical properties of Li2ZnTi3O8/C anode material in lithium-ion batteries. J. Alloys Compd. 613, 267–274. doi: 10.1016/j.jallcom.2014.06.050
Tang, H. Q., Tang, Z. Y., Du, C. Q., Qie, F. C., and Zhu, J. T. (2014a). Ag-doped Li2ZnTi3O8 as a high rate anode material for rechargeable lithium-ion batteries. Electrochim. Acta 120, 187–192. doi: 10.1016/j.electacta.2013.12.090
Tang, H. Q., Zhu, J. T., Tang, Z. Y., and Ma, C. X. (2014b). Al-doped Li2ZnTi3O8 as an effective anode material for lithium-ion batteries with good rate capabilities. J. Electroanal. Chem. 731, 60–65. doi: 10.1016/j.jelechem.2014.08.011
Tang, Y. Q., Liu, L., Huang, X. B., Ding, X., Zhou, S. B., and Chen, Y. D. (2019). Synthesis and electrochemical properties of Li2FeSiO4/C/Ag composite as a cathode material for Li-ion battery. J. Central South Univ. 26, 1443–1448. doi: 10.1007/s11771-019-4100-0
Tarascon, J. M., and Armand, M. (2001). Issues and challenges facing rechargeable lithium batteries. Nature 414, 359–367. doi: 10.1038/35104644
Tian, B. B., Xiang, H. F., Zhang, L., Li, Z., and Wang, H. H. (2010). Niobium doped lithium titanate as a high rate anode material for Li-ion batteries. Electrochim. Acta 55, 5453–5458. doi: 10.1016/j.electacta.2010.04.068
Wang, M. X., Huang, X. B., Wang, H. Y., Zhou, T., Xie, H. S., and Ren, Y. R. (2019). Synthesis and electrochemical performances of Na3V2(PO4)2F3/C composites as cathode materials for sodium ion batteries. RSC Adv. 9, 30628–30636. doi: 10.1039/C9RA05089B
Wang, M. X., Wang, K., Huang, X. B., Zhou, T., Xie, H. S., and Ren, Y. R. (2020). Improved sodium storage properties of Zr-doped Na3V2(PO4)2F3/C as cathode material for sodium ion batteries. Ceramics Int. 46, 28490–28498. doi: 10.1016/j.ceramint.2020.08.006
Wang, S., Bi, Y. F., Wang, L. J., Meng, Z. H., and Luo, B. M. (2019). Mo-doped Li2ZnTi3O8@graphene as a high performance anode material for lithium-ion batteries. Electrochim. Acta 301, 319–324. doi: 10.1016/j.electacta.2019.01.168
Wu, H. B., Chang, S., Liu, X. L., Yu, L. Q., Wang, G. L., Cao, D. X., et al. (2013). Sr-doped Li4Ti5O12 as the anode material for lithium-ion batteries. Solid State Ionics 232, 13–18. doi: 10.1016/j.ssi.2012.10.027
Xiao, H., Huang, X. B., Ren, Y. R., Ding, X., Chen, Y. D., and Zhou, S. B. (2019). Improved electrochemical performance of Na3V2(PO4)3 modified by N-doped carbon coating and Zr doping. Solid State Ionics 333, 93–100. doi: 10.1016/j.ssi.2019.01.025
Xiao, H., Huang, X. B., Ren, Y. R., Wang, H. Y., Ding, J. N., Zhou, S. B., et al. (2018). Enhanced sodium ion storage performance of Na3V2(PO4)3 with N-doped carbon by folic acid as carbon-nitrogen source. J. Alloys Compd. 732, 454–459. doi: 10.1016/j.jallcom.2017.10.195
Xu, Y. X., Hong, Z. S., Xia, L. C., Yang, J., and Wei, M. D. (2013). One step sol-gel synthesis of Li2ZnTi3O8/C nanocomposite with enhanced lithium-ion storage properties. Electrochim. Acta 88, 74–78. doi: 10.1016/j.electacta.2012.10.044
Yi, T. F., Pan, J. J., Wei, T. T., Li, Y. W., and Cao, G. Z. (2020a). NiCo2S4-based nanocomposites for energy storage in supercapacitors and batteries. Nano Today 33:100894. doi: 10.1016/j.nantod.2020.100894
Yi, T. F., Qiu, L. Y., Mei, J., Qi, S. Y., Cui, P., Luo, S. H., et al. (2020c). Porous spherical NiO@NiMoO4@PPy nanoarchitectures as advanced electrochemical pseudocapacitor materials. Sci. Bull. 65, 546–556. doi: 10.1016/j.scib.2020.01.011
Yi, T. F., Wei, T. T., Li, Y., He, Y. B., and Wang, Z. B. (2020b). Efforts on enhancing the Li-ion diffusion coefficient and electronic conductivity of titanate-based anode materials for advanced Li-ion batteries. Energy Stor. Mater. 26, 165–197. doi: 10.1016/j.ensm.2019.12.042
Yoshio, N. (2001). Lithium ion secondary batteries; past 10 years and the future. J. Power Sources 100, 101–106. doi: 10.1016/S0378-7753(01)00887-4
Yu, Z. J., Zhang, X. F., Yang, G. L., Liu, J., Wang, J. W., Wang, R. S., et al. (2011). High rate capability and long-term cyclability of Li4Ti4.9V0.1O12 as anode material in lithium ion battery. Electrochim. Acta 56, 8611–8617. doi: 10.1016/j.electacta.2011.07.051
Zhang, B., Huang, Z. D., Oh, S. W., and Kim, J. K. (2011). Improved rate capability of carbon coated Li3.9Sn0.1Ti5O12 porous electrodes for Li-ion batteries. J. Power Sources 196, 10692–10697. doi: 10.1016/j.jpowsour.2011.08.114
Keywords: lithium ion battery, Li2ZnTi3O8, Cr doping, anode material, Li2ZnTi2.9Cr0.1O8
Citation: Zeng X, Peng J, Zhu H, Gong Y and Huang X (2021) Cr-Doped Li2ZnTi3O8 as a High Performance Anode Material for Lithium-Ion Batteries. Front. Chem. 8:600204. doi: 10.3389/fchem.2020.600204
Received: 29 August 2020; Accepted: 30 September 2020;
Published: 28 January 2021.
Edited by:
Bin Huang, Guilin University of Technology, ChinaReviewed by:
Ting-Feng Yi, Northeastern University at Qinhuangdao, ChinaHongwei Mi, Shenzhen University, China
Copyright © 2021 Zeng, Peng, Zhu, Gong and Huang. This is an open-access article distributed under the terms of the Creative Commons Attribution License (CC BY). The use, distribution or reproduction in other forums is permitted, provided the original author(s) and the copyright owner(s) are credited and that the original publication in this journal is cited, in accordance with accepted academic practice. No use, distribution or reproduction is permitted which does not comply with these terms.
*Correspondence: Xianguang Zeng, aG56eGcxOTc5JiN4MDAwNDA7MTI2LmNvbQ==; Huafeng Zhu, NTMwNjg5NzImI3gwMDA0MDtxcS5jb20=