- 1School of Materials Science and Engineering, Xiangtan University, Xiangtan, China
- 2Tianjin Key Laboratory of Composite and Functional Materials, School of Materials Science and Engineering, Tianjin University, Tianjin, China
Reasonable design and development of a low-cost and high-efficiency bifunctional electrocatalyst for oxygen evolution reaction (OER) and oxygen reduction reaction (ORR) is essential for promoting the development of Zinc-air battery technology. Herein, we obtained an integrated catalytic electrode, NiFe nanoparticles supported on nitrogen-doped carbon (NC) directly grown on the carbon cloth (designated as Ni3Fe2@NC/CC), by pyrolysis of bimetallic NiFe metal-organic framework (MOF) precursor. There is a synergistic effect between nickel and iron component, which enhances the bifunctional catalytic activity. In addition, the underlying carbon cloth is conducive to the efficient electron transfer and also benefits the uniform loading of catalytically active materials. Thus, the integrated electrode shows good OER/ORR dual-functional catalytic performance, and the OER overpotential is much lower than that of the traditional drop-coating electrode and precious metal catalyst (IrO2). Moreover, the Ni3Fe2@NC/CC integrated electrode used in zinc-air batteries shows good flexibility and cycle stability. Our findings provide a new avenue for the development of efficient and stable bifunctional oxygen electrocatalysts.
Introduction
The crisis from environmental and energy issues have driven the research and development of new energy technologies on a global scale (Poizot and Dolhem, 2011; Larcher and Tarascon, 2015; Chu et al., 2016; Stamenkovic et al., 2016; Li and Wang, 2019; Chen et al., 2020). Among new energy technologies, sustainable energy conversion and storage technologies such as fuel cells, electrolyzed water devices, and metal-air batteries are developing rapidly (Anantharaj et al., 2016; Lee et al., 2016a; Zhang et al., 2016; Li and Lu, 2017; Yu et al., 2019; Wang et al., 2020). The metal-air battery has received increasing attention attribute to the advantages of low cost, high specific energy density, remarkable long-term stability, and environmental benignity (Fu et al., 2017b; Pan et al., 2018; Xiong and Ivey, 2018). Moreover, the theoretical energy density of metal-air battery is 5~10 times than that of lithium-ion batteries (Gu et al., 2017). Metal-air batteries include a metal anode (metal=lithium, zinc, magnesium, aluminum, etc.) and an air cathode. On the one hand, the metal-air battery is suitable for practical applications due to its abundant resources, low price, and environmental friendliness (Chen et al., 2018; Wang et al., 2019). On the other hand, the battery performance can be further improved by optimizing the electrode reaction kinetic of the air cathode (Cheng and Chen, 2012; Lee et al., 2016a). During the charge-discharge process, the electrode reactions on the air cathode are oxygen evolution reaction (OER) and oxygen reduction reaction (ORR) (Jiang et al., 2016; Han et al., 2017; Suen et al., 2017). Rational design and synthesis of catalytic materials to promote the process of OER/ORR have attracted the interest of many researchers.
So far, precious metals and their alloys are still considered to be state-of-the-art electrocatalysts for OER/ORR, such as Pt-based material catalysts with high ORR activity, Ir-based or Ru-based material catalysts with high OER activity (Lee et al., 2012; Liu et al., 2019a). However, the prohibitive cost, low reserves, and poor stability severely restrict the large-scale production and commercial application. Since transition metals (such as iron, cobalt, and nickel) based catalysts have the characteristics of low cost, earth-abundance, and good chemical stability, they are recognized as ideal materials to replace noble metals (Han et al., 2019a; Shi et al., 2019). In recent years, there has been a lot of research results on transition metal catalysts (Aijaz et al., 2016; Han et al., 2016). Transition metal, transition metal alloys and their derivatives (such as oxides, hydroxides and carbides) are developed as low-cost oxygen electrocatalysts, which have become a kind of substitute for precious metals and have attracted widespread attention (Gong et al., 2013; Cai et al., 2016; Fu et al., 2017a; Liu et al., 2019b; Xie et al., 2019). However, a large number of experimental studies have shown that it is difficult for a single metal catalyst to meet the bifunctional catalytic requirements (Liang et al., 2013; Park et al., 2017; Li et al., 2018). Therefore, the development of OER/ORR bifunctional catalysts based on earth-abundant elements with satisfied electrochemical activity and excellent stability still remains a great challenge.
The synergistic effect between different metals in bimetallic materials can effectively change the electronic structure of materials and reduce the free energy of reaction, leading to the effective bifunctional capability (Su et al., 2017). Besides, rational design of catalytic electrode structure is an important approach to improve the electrochemical performance. The traditional electrode preparation process is to disperse the powdered catalyst in a solvent and prepare an electrode slurry, which is then coated on carbon cloth or carbon paper to prepare the final air cathode. The preparation method not only makes the catalyst loaded on the support non-uniform, but also causes the weak interaction between the functional phase and the support, resulting in poor electrode stability. Moreover, the binder added in the preparation of electrode slurry will cover the catalytic active site and increase the interfacial resistance, unfavorable for the activity enhancement.
In this work, a novel NiFe nanoparticles supported on nitrogen-doped carbon (NC) hybrid material, directly grown on the carbon cloth (designated as Ni3Fe2@NC/CC), was proposed and synthesized as an integrated electrode for promoting OER/ORR electrocatalysis and zinc-air batteries. The designed synthetic strategy includes a chemical precipitation method and then a one-step pyrolysis procedure. Compared with the traditional electrode in which Ni3Fe2@NC is prepared as an electrode slurry drop-coated on carbon cloth, the Ni3Fe2@NC/CC integrated electrode exhibits greatly improved catalytic activity. When the anodic current density reaches 10 mA cm−2, the OER overpotential is 238 mV, which is lower than the Ni3Fe2@NC drop coating electrode (340 mV) and the precious metal oxide IrO2 (400 mV). The Ni3Fe2@NC/CC also exhibits remarkable long-term catalytic durability. It can be directly used as the positive electrode for practical aqueous and flexible semi-solid zinc-air batteries, which deliver large discharging capacity, more than 80 discharging-charging cycles and good flexibility.
Experimental Section
Materials Synthesis
In a typical synthetic procedure, potassium hexamethylene ferrite (1.33 g) was put in a 200 mL beaker, and then added in 100 mL deionized water and pre-treated carbon cloths to form settled solution A. In the meantime, nickel nitrate hexahydrate (1.74 g) and trisodium citrate (2.36 g) were dissolved into 200 mL water to form clear solution B. Solution A and solution B were mixed under strong magnetic stirring to form a homogeneous solution. After 24 h standing and ripening, NiFe-MOFs coated carbon cloths were taken out to dry at room temperature. At the same time, the solution was centrifuged to obtain the solid, washed with water and ethanol for three times, and then dried at room temperature to achieve the NiFe-MOFs powder. The NiFe-MOFs carbon cloths and powders were transferred into the porcelain boat. And then, the boat was placed in a tube furnace, heated to 500°C with a slow heating rate of 2°C min−1 under Ar atmosphere and kept for 1 h. After the temperature was naturally down to room temperature, the obtained integrated electrode and powder sample were collected and signed as Ni3Fe2@NC/CC and Ni3Fe2@NC, respectively.
Materials Characterization
The phase and crystal structure were characterized by an X-ray diffraction analyzer (XRD, Bruker D8 Advanced, CuKα radiation). Scanning electron microscope (SEM, s4800 Hitachi, 30 kV) with energy dispersive spectrometer (EDS), transmission electron microscope (TEM, JEOL JEM-2100F, 200 kV) and high-angle annular dark-field scanning transmission electron microscope (HAADF-STEM, JEM-ARM200F, 200 kV) were used to observe the microstructure and nanostructure of samples. The chemical valence and surface composition were detected by Thermo Scientific X-ray photoelectron spectroscopy (XPS, Escalab 250Xi).
Electrocatalytic Measurements
The electrochemical performance was tested at the IviumStat workstation. The electrochemical test is carried out in a three-electrode system, including a working electrode, a reference electrode, and a counter electrode. The integrated Ni3Fe2@NC/CC electrode prepared in this work can be directly used as the working electrode. The saturated calomel electrode is the reference electrode, and the carbon rod or platinum is the counter electrode. The obtained Ni3Fe2@NC powder samples were made into working electrodes according to traditional electrode preparation methods for comparison. Electrode slurry was prepared by suspending 10 mg Ni3Fe2@NC in a mixed solvent of deionized water 0.68 mL, isopropanol 0.23 mL, and Nafion 0.09 mL (v/v/v = 15/5/2). For noble metal catalysts, 8 mg IrO2 was mixed with 2 mg carbon powder. The OER working electrode was prepared by the drop-casting method, and the slurry was deposited on 1.0 × 1.0 cm2 carbon cloth (loading mass is about 1.0 mg cm−2) and dried at 60°C. The OER performance was tested in 1M KOH saturated with high purity N2. IrO2 electrode is also prepared by the same process. The ink of the catalyst was deposited on the glass carbon of the rotating disk electrode (RDE) with a diameter of 5 mm to prepare an ORR working electrode. Then, 10 μL of the resulting ink was coated on the electrode and dried in the air environment. The ORR performance of the catalyst was measured in 0.1 M KOH solution saturated with oxygen. First, several cyclic voltammetry (CV) curves were performing until the signal was stable. Linear sweep voltammetry of ORR and OER was performed at a scan rate of 10 mV s−1. The electrochemical impedance spectroscopy is performed in the frequency range of 100 kHz to 100 mHz. The electric double-layer capacitance (Cdl) was measured by CV. For data analysis, convert the potential to a reversible hydrogen electrode (RHE) according to the following formula: E (vs. RHE) = E (vs. SCE) + 0.059 × pH + 0.241 V. The OER polarization measurements were iR-corrected. The Koutecky-Levich (K-L) curve is obtained by the following formula:
Among them, I, Ik and Id represent measurement, kinetics and limit diffusion current density, n is the number of electrons transferred by oxygen reduction, F is the Faraday constant, A (cm2) is the geometric area of the electrode, k is the rate constant of the reaction, and C0 is the oxygen constant in a saturated 0.1 M KOH solution, D is the oxygen diffusion coefficient, ν is the solvent dynamic viscosity, and ω is the speed in rad s−1.
Assembly of Aqueous Zn-Air Batteries
The aqueous zinc-air battery was constructed by the anode, the cathode, and the electrolyte. The anode of the battery was a zinc plate, the electrolyte was 6.0 M KOH and 0.2 M ZnCl2 solution, and the air cathode was a catalyst coated on a 1 × 1 cm2 carbon cloth. The integrated electrode obtained in this work was directly used as an air cathode. Before testing, high-purity oxygen must be allowed to enter the electrolyte for about 20 min to reach the oxygen saturation state. During the test, oxygen should be continuously introduced at a small flow rate to ensure oxygen saturation of the electrolyte. The open-circuit voltage and reversible cycle of the zinc-air battery were tested by the LAND-CT2001A testing device.
Assembly of Flexible Semi-Solid Zn-Air Batteries
The flexible semi-solid zinc-air battery was composed of a zinc anode, a Polyvinyl alcohol (PVA) KOH solid electrolyte, and Ni3Fe2@NC/CC air cathode. The preparation method of the PVA-KOH electrolyte was shown below. Dissolve 3 g of PVA powder in 25 mL of deionized water and stir thoroughly at 90 °C to form a clear solution. Then add 6 mL 9 M KOH solution to mix, continue to stir at 90°C for 4 h, then put in the refrigerator to freeze for at least 3 h.
Results and Discussion
Figure 1 schematically elucidates the preparation process of the Ni3Fe2@NC/CC. In brief, nickel (II) nitrate hexahydrate, sodium citrate, and potassium ferricyanide are co-precipitated in deionized water to form NiFe-MOFs precursor. Carbon cloths are added during the precipitation formation. Ni3Fe2@NC and Ni3Fe2@NC/CC are finally achieved by calcining the precursor and the carbon cloth under the argon atmosphere at 773 K, respectively. The NiFe-MOFs nanoparticles with particle size about 100 nm uniformly loaded on the carbon fiber (Supplementary Figures 1A–C, Supporting Information). The XRD pattern of the precursor that had been sonicated from the carbon cloth was consistent with the theoretically fitted Ni3[Fe(CN)6]2·H2O, (Zhang et al., 2017), which means the successful formation of the NiFe-MOFs precursor (Supplementary Figure 2). After calcination at 773 K under an argon atmosphere, the organic precursors were carbonized into NC and the nanoparticles were anchored on the NC matrix. XRD analysis of the resultant composites elucidated that the dominant phase was bimetal NiFe with three obvious diffraction peaks at 44, 51, and 75°, which can be assigned, respectively, to the (111), (200), and (220) planes of NiFe alloy (Supplementary Figure 3A). Energy dispersive spectrum (EDS) showed that the atomic ratio of Ni and Fe in the calcined sample was 3:2 (Supplementary Figure 3B and Supplementary Table 1). Comprehensive analysis of XRD and EDS data demonstrates the successful synthesis of nickel-iron nanoparticles on the N-doped carbon nanocube structure. Furthermore, as shown in Figures 2a–c, Ni3Fe2@NC nanoparticles evenly loaded on the carbon cloth with the average particle size of ~100 nm. For further structural analysis of nanoparticles on carbon cloth, put the calcined integrated electrode in absolute ethanol and sonicate, and the dissolving sample was taken to perform TEM test. TEM further confirms the nanoparticles with the size of about 100 nm (Figure 2d). The high-resolution TEM (HRTEM) image proved distinct domains of NiFe alloy with the interplanar spacing of 0.20 and 0.17 nm, corresponding to the (111) and (200) facets of NiFe alloy, respectively (Figure 2e). To further characterize the NiFe alloy, an aberration-corrected high-angle annular dark-field scanning transmission electron microscope (HAADF-STEM) test was performed on it. The HAADF-STEM image showed NiFe alloy with a heavier element mass on an NC substrate exists in the form of bright spots, and the particle size of the NiFe alloys is about 2 ~ 3 nm (Figure 2f). Also, elemental mapping of Ni3Fe2@NC revealed the homogeneous dispersion of C, N, Ni, and Fe species throughout the entire nanoarchitecture (Figure 2g), suggesting the uniform loading of NiFe nanoparticles on N-doped carbon structure.
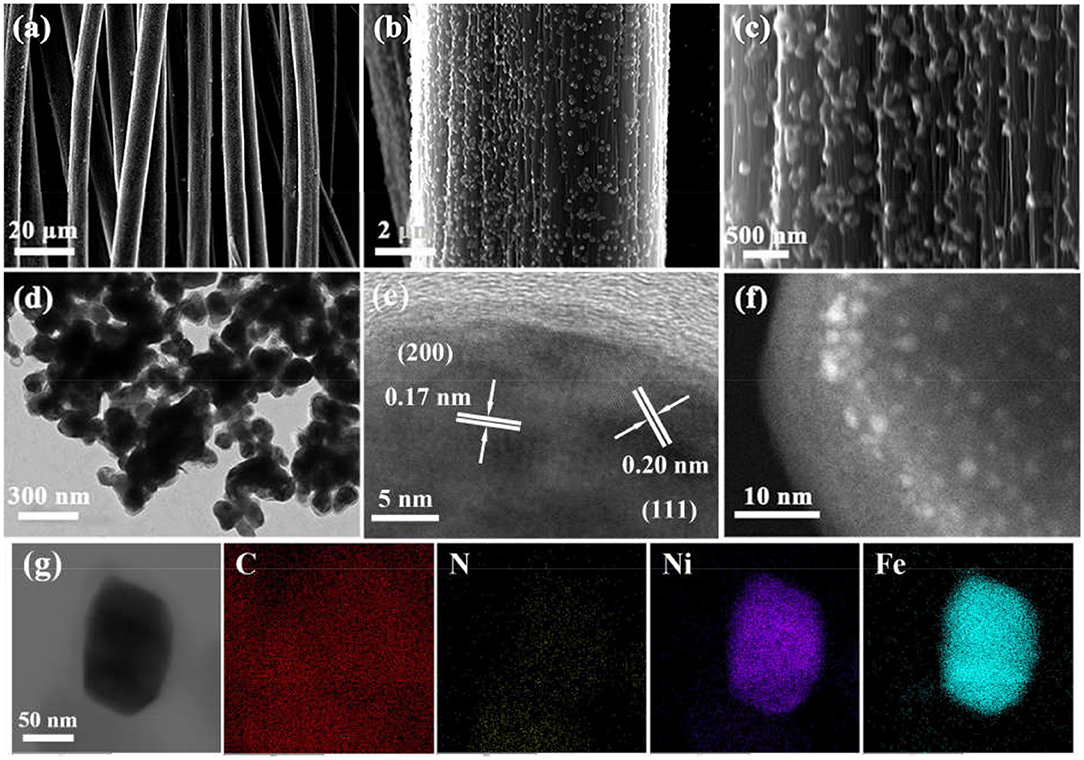
Figure 2. (a–c) SEM images from low to high magnification of Ni3Fe2@NC/CC. (d) TEM, (e) HRTEM, (f) HAADH-STEM, and (g) elemental mapping images of Ni3Fe2@NC.
X-ray photoelectron spectroscopy (XPS) was employed to characterize the chemical states and surface structure of Ni3Fe2@NC materials. The photoelectron peaks in XPS survey spectra confirm the existence of C, N, O, Fe, and Ni species (Supplementary Figure 4). The high-resolution C 1s spectrum revealed the presence of C-C (284.7 eV), C=N (285.8 eV), C-N (287.5 eV), and O-C=O (289.6 eV) bonds in Ni3Fe2@NC (Figure 3A) (Sheng et al., 2011; Gao et al., 2014). The N 1s spectrum was deconvoluted into three peaks, which can be assigned to pyridinic N (398.5 eV), pyrrolic N (400.2 eV), and N-O (403.5 eV) (Figure 3B) (Niu et al., 2015; Han et al., 2019b). However, it is worth noting that pyridinic N can bond with metal atoms. The existence of C=N and C-N bond indicated the successful doping of N into the carbon skeleton. The two peaks at 852.8 and 855.2 eV in Ni 2p spectrum were ascribed to Ni 2p3/2 of Ni0 and Ni2+ (Figure 3C), respectively. The other two fitting peaks at 870.1 and 872.5 eV were attributed to Ni 2p1/2 of Ni0 and Ni2+ (Lee et al., 2016b; Yang et al., 2016; Huang et al., 2017; Wan et al., 2017). The two shakeup satellites of ionic state nickel located at 860.4 and 878.9 eV. The peaks in the Fe 2p spectrum included two peaks of zero-valence state (707.1 and 720.2 eV) and two peaks of high valence state (711.1 and 724.2 eV) with two peaks of shakeup satellites (716.3 and 733.2 eV), which were derived from NiFe nanoparticle and Fe-N species (Figure 3D) (Cui et al., 2013; Jiang et al., 2015; Dai et al., 2016). In general, these aforementioned results demonstrated the presence of NiFe alloy and the metal-N coordination in prepared Ni3Fe2@NC/CC electrode.
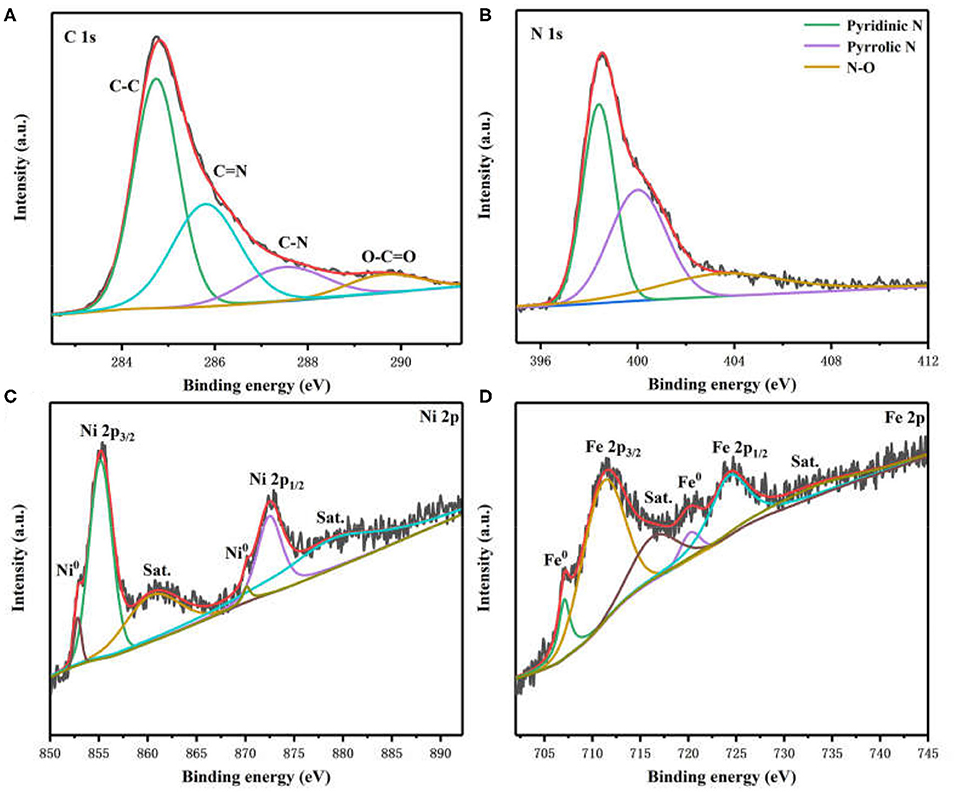
Figure 3. High-resolution XPS spectra of (A) C 1 s, (B) N 1 s, (C) Ni 2p, and (D) Fe 2p in Ni3Fe2@NC.
The electrocatalytic activities of Ni3Fe2@NC/CC were evaluated by comparing with noble metal IrO2 catalyst and Ni3Fe2@NC in a typical three-electrode configuration using 1.0 M KOH solution as the electrolyte. As displayed in the OER linear sweep voltammograms (LSV), Ni3Fe2@NC/CC possessed superior OER activity (Figure 4A). Ni3Fe2@NC/CC required an overpotential of 238 mV to reach a 10 mA cm−2 current density, which was much lower than those of Ni3Fe2@NC (340 mV) and IrO2 (400 mV). The OER activity of Ni3Fe2@NC/CC actually surpasses most previously reported electrocatalysts (Table 1). The fitted Tafel plots revealed that Ni3Fe2@NC/CC equipped a Tafel slope of 50.2 mV dec−1, lower than that of Ni3Fe2@NC (56.5 mV dec−1) and IrO2 (83.1 mV dec−1), which indicated its favorable reaction kinetic (Figure 4B). Electrochemical impedance spectroscopy (EIS) analysis suggested that the charge-transfer resistance (Rct) values were around 0.3, 2.2, and 3.4 Ω for Ni3Fe2@NC/CC, Ni3Fe2@NC and IrO2, respectively (Figure 4C), which was an indication that a rapid charge transfer rate in Ni3Fe2@NC/CC. This trend was in accordance with the polarization results and Tafel data. The above results show that, compared with the traditional drop-coated electrode, the integrated electrode gives excellent catalytic activity, which fully confirmed the structural advantages of the integrated electrode in improving the overall electrochemical activity. In addition to its high catalytic activity, the long-term stability of Ni3Fe2@NC/CC is also an important parameter for practical performance. As shown in Supplementary Figure 5, the catalyst Ni3Fe2@NC/CC exhibited remarkable OER stability at a constant current of 10 mA cm−2 after continuous operation for 200 min. The LSV curve of Ni3Fe2@NC/CC nearly overlapped the initial one after continuous 1,000 cyclic voltammetry (CV) cycles (Figure 4D). This result manifested that the Ni3Fe2@NC/CC hybrid can still maintain its activity after a large number of cycles and has fairly good stability. It can be seen from the Supplementary Figure 6 that the morphology of the Ni3Fe2@NC/CC has not changed significantly after the stability test, which further proves the strong interaction. Since bifunctional oxygen catalytic performance is required in rechargeable Zinc-air battery, the ORR activity of synthesized composites was assessed by coating on rotating disk electrodes (RDEs). As displayed in Figure 4E, Ni3Fe2@NC exhibited an onset potential of 0.87 V, a half-wave potential of 0.73 V, and a limiting diffusion current density of 4.5 mA cm−2 at a rotating speed of 1,600 round per minute (rpm), which is comparable to many reported bifunctional oxygen electrocatalysts (Supplementary Table 2). The fitted Koutechy-Levich plot of Ni3Fe2@NC disclosed an apparent 4-electron reaction pathway, which is deemed to a highly efficient mechanism dominating the ORR process (Figure 4F). The electrochemically active surface area (EASC) was measured by the double-layer capacitance (Cdl) to be 2.5 mF cm−2 for Ni3Fe2@NC, indicating the benefits of NiFe nanoparticles and NC substrate in exposing more electrochemical active sites (Supplementary Figures 7A,B). Apart from the catalytic activity, Ni3Fe2@NC also showed remarkable ORR catalytic stability. After 9 h continuous chronoamperometric treatment, the active current retention of Ni3Fe2@NC was 80% (Supplementary Figure 8). The long-term stability was further demonstrated by the almost overlapping CV curves after 1,000 cycles (Supplementary Figure 8 inset). The aforementioned experimental results jointly confirm that the Ni3Fe2@NC/CC hybrid electrode has efficient bifunctional OER/ORR performance and long-term durability, indicating that it has promising application prospects in reversible oxygen electrocatalysis for rechargeable metal-air batteries and regenerative fuel cells.
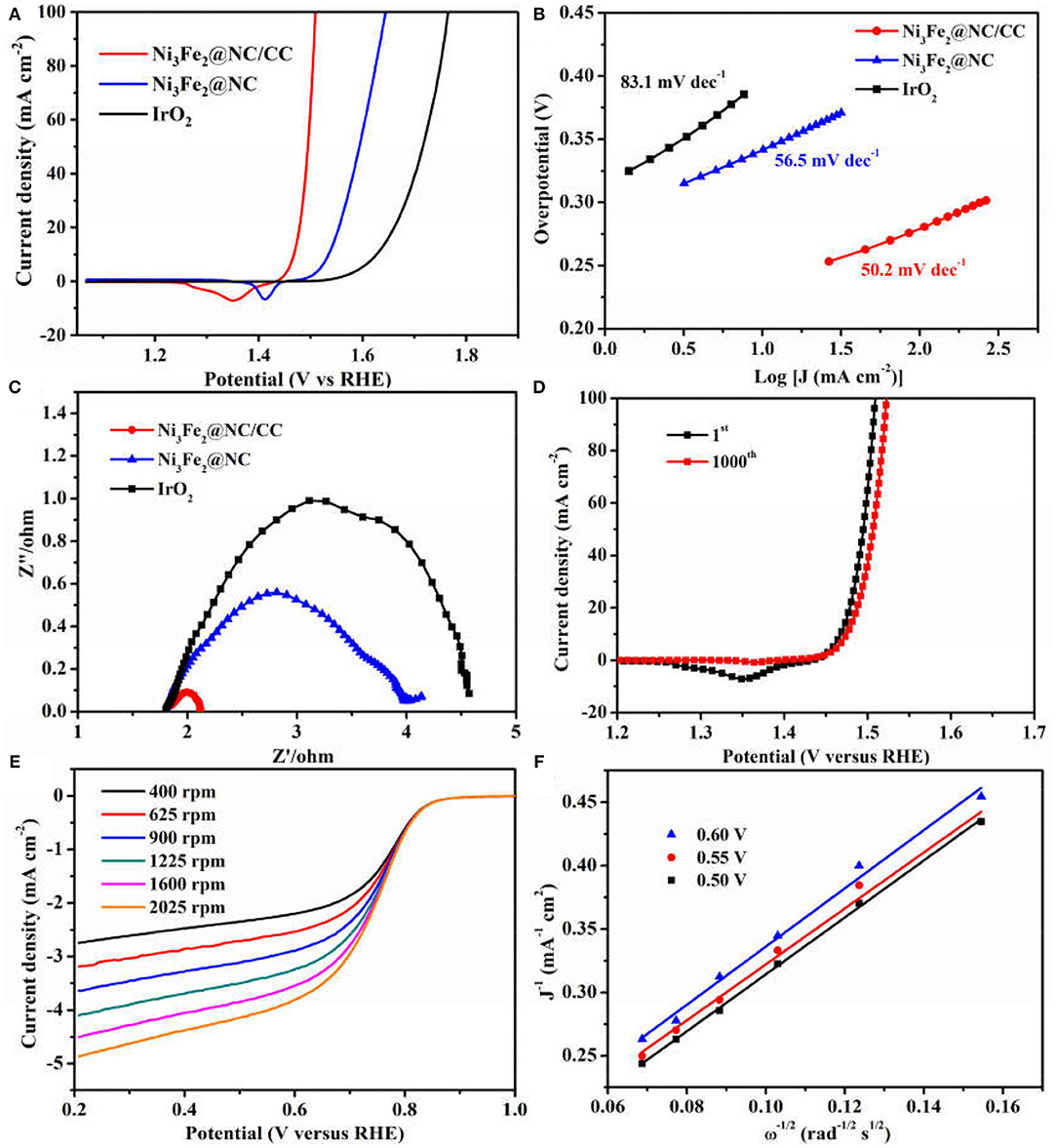
Figure 4. (A) OER polarization curves of Ni3Fe2@NC/CC, Ni3Fe2@NC, and IrO2. (B) Corresponding Tafel plots. (C) Corresponding EIS spectra. (D) OER LSV plots of Ni3Fe2@NC/CC before and after 1,000 cycles. (E) ORR polarization curves of Ni3Fe2@NC at different rotation rates. (F) The K-L plots for Ni3Fe2@NC at different potentials.
A home-made liquid zinc-air battery was constructed to evaluate the practical electrochemical performance of Ni3Fe2@NC/CC, in which a zinc plate was served as the anode, the Ni3Fe2@NC/CC integrated electrode as the air cathode, and 6.0 M KOH and 0.2 M ZnCl2 as the electrolyte (Figure 5A). The alkaline zinc-air batteries based on Ni3Fe2@NC/CC materials exhibit a steady open-circuit voltage of 1.45 V (Figure 5B). In addition, with the current density of 5 mA cm−2 and the duration of each cycle of 20 min, the cycle chargeability of the secondary battery was further studied (Figure 5C). It is worth noting that under the catalysis of Ni3Fe2@NC/CC electrode, the discharge voltage of the battery is 1.11 V, the charging voltage is 1.95 V, the voltage gap is 0.84 V, and the energy efficiency is 56.9%. Small voltage decay is observed after 80 cycles for Ni3Fe2@NC/CC cathode, which reflects the excellent rechargeability and is much better than the precious metal Pt/C-IrO2 catalyst (<50 cycles). The discharge specific capacity of a primary Zinc-air battery with Ni3Fe2@NC/CC cathode is 655 mA h g−1 at 20 mA cm−2 based on the mass of consumed zinc (Supplementary Figure 9). Encouraged by the potential application for portable and wearable devices, a flexible solid-state zinc-air battery was assembled with zinc plate anode, PVA-KOH electrolyte and Ni3Fe2@NC/CC integrated cathode. The open circuit voltage of solid zinc-air battery promoted by Ni3Fe2@NC/CC can reach 1.37 V, again indicating the efficient ORR activity of the integrated cathode (Supplementary Figure 10). As shown in Figure 5D inset, a light emitting diode (LED) screen was powered by two Ni3Fe2@NC/CC-based solid flexible Zinc-air batteries in series. The voltage-current polarization curves revealed that the Ni3Fe2@NC/CC cathode possessed a good charge-discharge performance (Figure 5D). The discharge voltage at 10 mA cm−2 is 1.09 V and the charge voltage is 2.08 V when the battery is flat. Moreover, the battery exhibits considerable flexibility. When the assembled battery is bent about 30°, the polarization curve shows that the discharge voltage is 1.07 V at a current density of 10 mA cm−2, which is only 1.8% lower than the discharge voltage in the flat state of the battery, and the charging voltage is 2.20 V, which is 5.7% higher. As shown in Figure 5E, the assembled battery can be alternately flat and bent (once every 5 charge-discharge cycles). Under a large mechanical strain, the battery can maintain a good charge and discharge cycle under continuous charging and discharging conditions. These results fully prove the good flexibility and cycle stability of Ni3Fe2@NC/CC-based rechargeable zinc-air batteries.
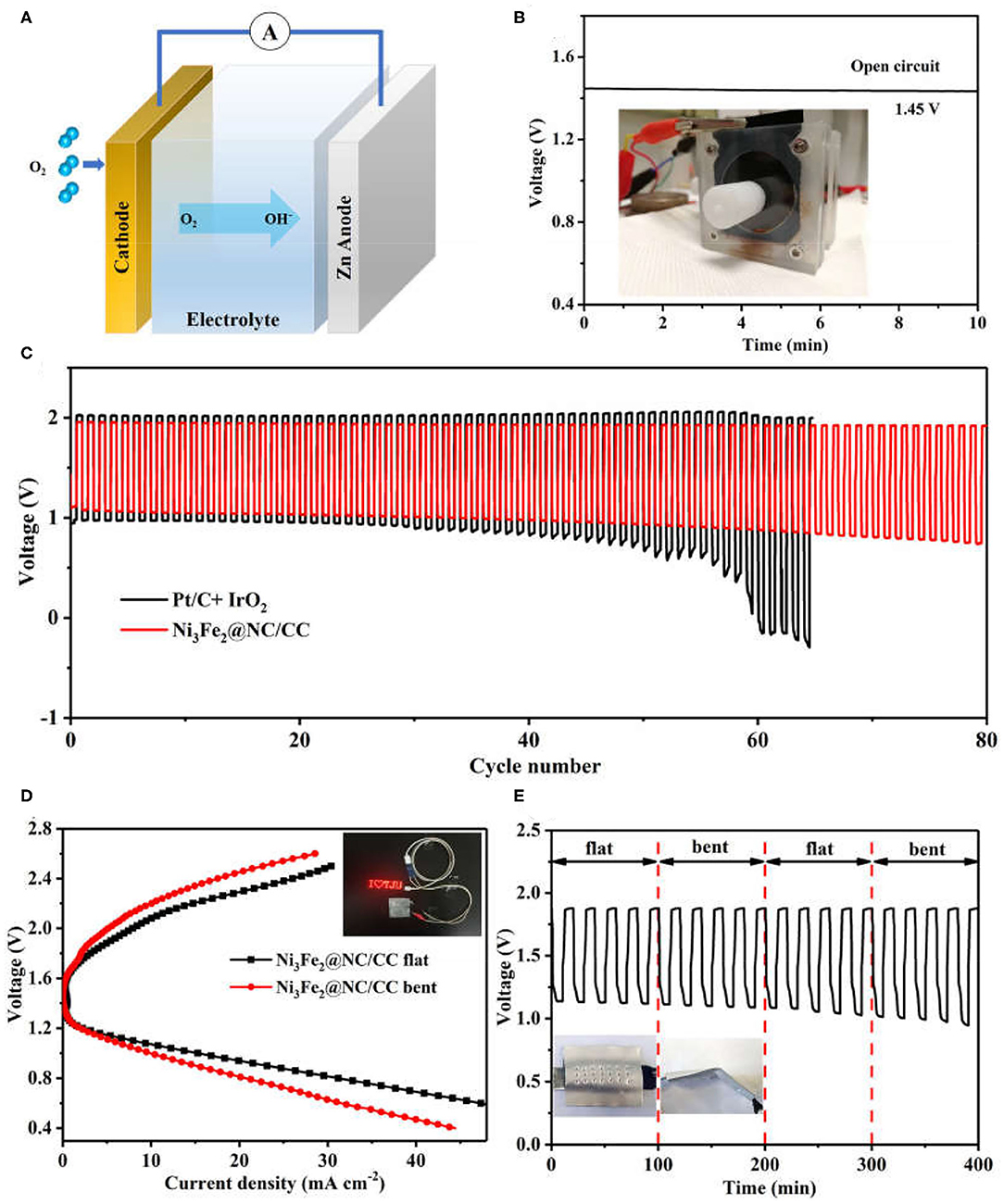
Figure 5. (A) Schematic configuration of an aqueous Zinc-air battery. (B) Open-circuit voltages of Ni3Fe2@NC/CC based zinc-air batteries. Inset shows a Zinc-air battery under test. (C) Cycling performance of rechargeable zinc-air batteries. (D) Discharge and charge polarization curves and (E) cycling performance of Ni3Fe2@NC/CC-based flexible battery at 2 mA cm−2. Inset shows a LED screen powered by Ni3Fe2@NC/CC-based zinc-air batteries.
Conclusion
In conclusion, Ni3Fe2@NC/CC integrated electrode was prepared by uniformly loading nickel-iron bimetallic nanoparticles on nitrogen-doped carbon nanostructures grown on carbon cloth support by chemical precipitation and then high-temperature calcination. The preparation technology of the integrated electrode with the catalyst uniformly supported on the conductive carrier is simple, and the electrode presents high catalytic activity and good stability. The prepared Ni3Fe2@NC/CC shows enhanced OER/ORR activity and durability either in electrochemical half-reaction tests or reversible zinc-air batteries, which is superior to noble metals and many previously developed electrocatalysts. Further experimental analysis demonstrates the advantages of in-situ integrated electrode in facilitating the electrode transfer and strong interaction between the active materials and the current collector, thereby promoting the electrocatalytic performance. Our findings provide a simple strategy for the preparation of bimetallic nanocomposites and open up a new way for the development of promising hybrid catalysts for electrochemical and energy-related applications.
Data Availability Statement
The original contributions presented in the study are included in the article/Supplementary Material, further inquiries can be directed to the corresponding author/s.
Author Contributions
HH and XL conducted the experiments and write the manuscript. CT helped with operating the experiments and data analysis. JL and XH interpreted the results. XH and WH supervised the research. All authors approved the submission of final manuscript.
Supporting Information
SEM images and XRD pattern of precursors, XRD pattern, EDS, survey XPS, CVs, Cdl and chronoamperometric curves of Ni3Fe2@NC/CC. Primary discharge curve of zinc-air battery, Table of comparison of OER/ORR electrocatalytic performance.
Funding
This work was supported by the National Natural Science Foundation of China (51972224 and U1601216), Young Elite Scientists Sponsorship Program by CAST (2018QNRC001).
Conflict of Interest
The authors declare that the research was conducted in the absence of any commercial or financial relationships that could be construed as a potential conflict of interest.
Supplementary Material
The Supplementary Material for this article can be found online at: https://www.frontiersin.org/articles/10.3389/fchem.2020.575288/full#supplementary-material
References
Aijaz, A., Masa, J., Rosler, C., Xia, W., Weide, P., Botz, A. J., et al. (2016). Co@Co3O4 encapsulated in carbon nanotube-grafted nitrogen-doped carbon polyhedra as an advanced bifunctional oxygen electrode. Angew. Chem. Int. Ed. 55, 4087–4091. doi: 10.1002/anie.201509382
Anantharaj, S., Ede, S. R., Sakthikumar, K., Karthick, K., Mishra, S., and Kundu, S. (2016). Recent trends and perspectives in electrochemical water splitting with an emphasis on sulfide, selenide, and phosphide catalysts of Fe, Co, and Ni: a review. ACS Catal. 6, 8069–8097. doi: 10.1021/acscatal.6b02479
Barati Darband, G., Aliofkhazraei, M., and Rouhaghdam, A. S. (2019). Facile electrodeposition of ternary Ni-Fe-Co alloy nanostructure as a binder free, cost-effective and durable electrocatalyst for high-performance overall water splitting. J. Colloid Interface Sci. 547, 407–420. doi: 10.1016/j.jcis.2019.03.098
Cai, P., Ci, S., Zhang, E., Shao, P., Cao, C., and Wen, Z. (2016). FeCo alloy nanoparticles confined in carbon layers as high-activity and robust cathode catalyst for Zn-air battery. Electrochim. Acta 220, 354–362. doi: 10.1016/j.electacta.2016.10.070
Chen, A., Zhang, X., and Zhou, Z. (2020). Machine learning: accelerating materials development for energy storage and conversion. InfoMat. 2, 553–576. doi: 10.1002/inf2.12094
Chen, R., Hung, S. F., Zhou, D., Gao, J., Yang, C., Tao, H., et al. (2019). Layered structure causes bulk NiFe layered double hydroxide unstable in alkaline oxygen evolution reaction. Adv. Mater. 31:1903909. doi: 10.1002/adma.201903909
Chen, X., Zhong, C., Liu, B., Liu, Z., Bi, X., Zhao, N., et al. (2018). Atomic layer Co3O4 nanosheets: the key to knittable Zn-air batteries. Small 14:1702987. doi: 10.1002/smll.201702987
Cheng, F., and Chen, J. (2012). Metal-air batteries: from oxygen reduction electrochemistry to cathode catalysts. Chem. Soc. Rev. 41, 2172–2192. doi: 10.1039/c1cs15228a
Chu, S., Cui, Y., and Liu, N. (2016). The path towards sustainable energy. Nat. Mater. 16, 16–22. doi: 10.1038/nmat4834
Cui, H.-J., Shi, J.-W., Yuan, B., and Fu, M.-L. (2013). Synthesis of porous magnetic ferrite nanowires containing Mn and their application in water treatment. J. Mater. Chem. A 1, 5902–5907. doi: 10.1039/c3ta01692g
Dai, Y., Chan, Y., Jiang, B., Wang, L., Zou, J., Pan, K., et al. (2016). Bifunctional Ag/Fe/N/C catalysts for enhancing oxygen reduction via cathodic biofilm inhibition in microbial fuel cells. ACS Appl. Mater. Interfaces 8, 6992–7002. doi: 10.1021/acsami.5b11561
Fang, L., Jiang, Z., Xu, H., Liu, L., Guan, Y., Gu, X., et al. (2018). Crystal-plane engineering of NiCo2O4 electrocatalysts towards efficient overall water splitting. J. Catal. 357, 238–246. doi: 10.1016/j.jcat.2017.11.017
Fu, G., Cui, Z., Chen, Y., Xu, L., Tang, Y., and Goodenough, J. B. (2017a). Hierarchically mesoporous nickel-iron nitride as a cost-efficient and highly durable electrocatalyst for Zn-air battery. Nano Energy 39, 77–85. doi: 10.1016/j.nanoen.2017.06.029
Fu, J., Cano, Z. P., Park, M. G., Yu, A., Fowler, M., and Chen, Z. (2017b). Electrically rechargeable zinc-air batteries: progress, challenges, and perspectives. Adv. Mater. 29:1604685. doi: 10.1002/adma.201604685
Gao, M. R., Cao, X., Gao, Q., Xu, Y. F., Zheng, Y. R., Jiang, J., et al. (2014). Nitrogen-doped graphene supported CoSe2 nanobelt composite catalyst for efficient water oxidation. ACS Nano 8, 3970–3978. doi: 10.1021/nn500880v
Gong, M., Li, Y., Wang, H., Liang, Y., Wu, J. Z., Zhou, J., et al. (2013). An advanced Ni-Fe layered double hydroxide electrocatalyst for water oxidation. J. Am. Chem. Soc. 135, 8452–8455. doi: 10.1021/ja4027715
Gu, P., Zheng, M., Zhao, Q., Xiao, X., Xue, H., and Pang, H. (2017). Rechargeable zinc–air batteries: a promising way to green energy. J. Mater. Chem. A 5, 7651–7666. doi: 10.1039/C7TA01693J
Han, L., Dong, S., and Wang, E. (2016). Transition-metal (Co, Ni, and Fe)-based electrocatalysts for the water oxidation reaction. Adv. Mater. 28, 9266–9291. doi: 10.1002/adma.201602270
Han, X., Ling, X., Wang, Y., Ma, T., Zhong, C., Hu, W., et al. (2019a). Generation of nanoparticle, atomic-cluster, and single-atom cobalt catalysts from zeolitic imidazole frameworks by spatial isolation and their use in zinc-air batteries. Angew. Chem. Int. Ed. 58, 5359–5364. doi: 10.1002/anie.201901109
Han, X., Ling, X., Yu, D., Xie, D., Li, L., Peng, S., et al. (2019b). Atomically dispersed binary Co-Ni sites in nitrogen-doped hollow carbon nanocubes for reversible oxygen reduction and evolution. Adv. Mater. 31:e1905622. doi: 10.1002/adma.201905622
Han, X., Wu, X., Zhong, C., Deng, Y., Zhao, N., and Hu, W. (2017). NiCo2S4 nanocrystals anchored on nitrogen-doped carbon nanotubes as a highly efficient bifunctional electrocatalyst for rechargeable zinc-air batteries. Nano Energy 31, 541–550. doi: 10.1016/j.nanoen.2016.12.008
He, P., Yu, X. Y., and Lou, X. W. (2017). Carbon-incorporated nickel-cobalt mixed metal phosphide nanoboxes with enhanced electrocatalytic activity for oxygen evolution. Angew. Chem. Int. Ed. 56, 3897–3900. doi: 10.1002/anie.201612635
Huang, L., Ge, X., and Dong, S. (2017). A facile conversion of a Ni/Fe coordination polymer to a robust electrocatalyst for the oxygen evolution reaction. RSC Adv. 7, 32819–32825. doi: 10.1039/C7RA04280A
Jia, X., Zhao, Y., Chen, G., Shang, L., Shi, R., Kang, X., et al. (2016). Ni3FeN nanoparticles derived from ultrathin nife-layered double hydroxide nanosheets: an efficient overall water splitting electrocatalyst. Adv. Energy Mater. 6:1502585. doi: 10.1002/aenm.201502585
Jiang, H., Yao, Y., Zhu, Y., Liu, Y., Su, Y., Yang, X., et al. (2015). Iron carbide nanoparticles encapsulated in mesoporous Fe-N-doped graphene-like carbon hybrids as efficient bifunctional oxygen electrocatalysts. ACS Appl. Mater. Interfaces 7, 21511–21520. doi: 10.1021/acsami.5b06708
Jiang, W. J., Gu, L., Li, L., Zhang, Y., Zhang, X., Zhang, L. J., et al. (2016). Understanding the high activity of Fe-N-C electrocatalysts in oxygen reduction: Fe/Fe3C nanoparticles boost the activity of Fe-N(x). J. Am. Chem. Soc. 138, 3570–3578. doi: 10.1021/jacs.6b00757
Larcher, D., and Tarascon, J. M. (2015). Towards greener and more sustainable batteries for electrical energy storage. Nat Chem 7, 19–29. doi: 10.1038/nchem.2085
Lee, D. U., Xu, P., Cano, Z. P., Kashkooli, A. G., Park, M. G., and Chen, Z. (2016a). Recent progress and perspectives on bi-functional oxygen electrocatalysts for advanced rechargeable metal–air batteries. J. Mater. Chem. A 4, 7107–7134. doi: 10.1039/C6TA00173D
Lee, S. M., Cho, A., and Cho, Y. S. (2016b). Enhanced optical and piezoelectric characteristics of transparent Ni-doped BiFeO3 thin films on a glass substrate. RSC Adv. 6, 16602–16607. doi: 10.1039/C5RA27674H
Lee, Y., Suntivich, J., May, K. J., Perry, E. E., and Shao-Horn, Y. (2012). Synthesis and activities of rutile IrO2 and RuO2 nanoparticles for oxygen evolution in acid and alkaline solutions. J. Phys. Chem. Lett. 3, 399–404. doi: 10.1021/jz2016507
Li, C., Tan, H., Lin, J., Luo, X., Wang, S., You, J., et al. (2018). Emerging Pt-based electrocatalysts with highly open nanoarchitectures for boosting oxygen reduction reaction. Nano Today 21, 91–105. doi: 10.1016/j.nantod.2018.06.005
Li, M., Xiong, Y., Liu, X., Bo, X., Zhang, Y., Han, C., et al. (2015). Facile synthesis of electrospun MFe2O4 (M = Co, Ni, Cu, Mn) spinel nanofibers with excellent electrocatalytic properties for oxygen evolution and hydrogen peroxide reduction. Nanoscale 7, 8920–8930. doi: 10.1039/C4NR07243J
Li, X., and Wang, J. (2019). One-dimensional and two-dimensional synergized nanostructures for high-performing energy storage and conversion. InfoMat 2, 3–32. doi: 10.1002/inf2.12040
Li, Y., and Lu, J. (2017). Metal–air batteries: will they be the future electrochemical energy storage device of choice? ACS Energy Lett. 2, 1370–1377. doi: 10.1021/acsenergylett.7b00119
Liang, H.-W., Wei, W., Wu, Z.-S., Feng, X., and Müllen, K. (2013). Mesoporous metal–nitrogen-doped carbon electrocatalysts for highly efficient oxygen reduction reaction. J. Am. Chem. Soc. 135, 16002–16005. doi: 10.1021/ja407552k
Liu, M., Zhao, Z., Duan, X., and Huang, Y. (2019a). Nanoscale structure design for high-performance Pt-based ORR catalysts. Adv. Mater. 31:1802234. doi: 10.1002/adma.201802234
Liu, Y., Dong, P., Li, M., Wu, H., Zhang, C., Han, L., et al. (2019b). Cobalt nanoparticles encapsulated in nitrogen-doped carbon nanotube as bifunctional-catalyst for rechargeable Zn-air batteries. Front. Mater. 6:85. doi: 10.3389/fmats.2019.00085
Niu, W., Li, L., Liu, X., Wang, N., Liu, J., Zhou, W., et al. (2015). Mesoporous N-doped carbons prepared with thermally removable nanoparticle templates: an efficient electrocatalyst for oxygen reduction reaction. J. Am. Chem. Soc. 137, 5555–5562. doi: 10.1021/jacs.5b02027
Pan, J., Tian, X. L., Zaman, S., Dong, Z., Liu, H., Park, H. S., et al. (2018). Recent progress on transition metal oxides as bifunctional catalysts for lithium-air and zinc-air batteries. Batter. Supercaps 2, 336–347. doi: 10.1002/batt.201800082
Park, J., Sa, Y. J., Baik, H., Kwon, T., Joo, S. H., and Lee, K. (2017). Iridium-based multimetallic nanoframe@nanoframe structure: an efficient and robust electrocatalyst toward oxygen evolution reaction. ACS Nano 11, 5500–5509. doi: 10.1021/acsnano.7b00233
Poizot, P., and Dolhem, F. (2011). Clean energy new deal for a sustainable world: from non-CO2 generating energy sources to greener electrochemical storage devices. Energy Environ. Sci. 4, 2003–2019. doi: 10.1039/c0ee00731e
Sheng, Z. H., Shao, L., Chen, J. J., Bao, W. J., Wang, F. B., and Xia, X. H. (2011). Catalyst-free synthesis of nitrogen-doped graphene via thermal annealing graphite oxide with melamine and its excellent electrocatalysis. ACS Nano 5, 4350–4358. doi: 10.1021/nn103584t
Shi, X., Ling, X., Li, L., Zhong, C., Deng, Y., Han, X., et al. (2019). Nanosheets assembled into nickel sulfide nanospheres with enriched Ni3+ active sites for efficient water-splitting and zinc–air batteries. J. Mater. Chem. A. 7, 23787–23793. doi: 10.1039/C9TA03819A
Sivanantham, A., Ganesan, P., and Shanmugam, S. (2016). Hierarchical NiCo2S4 nanowire arrays supported on Ni Foam: an efficient and durable bifunctional electrocatalyst for oxygen and hydrogen evolution reactions. Adv. Funct. Mater. 26, 4661–4672. doi: 10.1002/adfm.201600566
Stamenkovic, V. R., Strmcnik, D., Lopes, P. P., and Markovic, N. M. (2016). Energy and fuels from electrochemical interfaces. Nat. Mater. 16, 57–69. doi: 10.1038/nmat4738
Su, C.-Y., Cheng, H., Li, W., Liu, Z.-Q., Li, N., Hou, Z., et al. (2017). Atomic modulation of FeCo-nitrogen-carbon bifunctional oxygen electrodes for rechargeable and flexible all-solid-state zinc-air battery. Adv. Energy Mater. 7:1602420. doi: 10.1002/aenm.201602420
Suen, N. T., Hung, S. F., Quan, Q., Zhang, N., Xu, Y. J., and Chen, H. M. (2017). Electrocatalysis for the oxygen evolution reaction: recent development and future perspectives. Chem. Soc. Rev. 46, 337–365. doi: 10.1039/C6CS00328A
Wan, H., Li, L., Zhang, J., Liu, X., Wang, H., and Wang, H. (2017). Nickel nanowire@porous NiCo2O4 nanorods arrays grown on nickel foam as efficient pseudocapacitor electrode. Front. Energy Res. 5:33. doi: 10.3389/fenrg.2017.00033
Wang, C., Yu, Y., Niu, J., Liu, Y., Bridges, D., Liu, X., et al. (2019). Recent progress of metal–air batteries—a mini review. Appl. Sci. 9:2787. doi: 10.3390/app9142787
Wang, H. F., Tang, C., Wang, B., Li, B. Q., and Zhang, Q. (2017). Bifunctional transition metal hydroxysulfides: room-temperature sulfurization and their applications in Zn-air batteries. Adv. Mater. 29:1702327. doi: 10.1002/adma.201702327
Wang, Y., Zhang, G., Ma, M., Ma, Y., Huang, J., Chen, C., et al. (2020). Ultrasmall NiFe layered double hydroxide strongly coupled on atomically dispersed FeCo-NC nanoflowers as efficient bifunctional catalyst for rechargeable Zn-air battery. Sci. China Mater. 63, 1182–1195. doi: 10.1007/s40843-020-1276-8
Wu, G., Chen, W., Zheng, X., He, D., Luo, Y., Wang, X., et al. (2017). Hierarchical Fe-doped NiOx nanotubes assembled from ultrathin nanosheets containing trivalent nickel for oxygen evolution reaction. Nano Energy 38, 167–174. doi: 10.1016/j.nanoen.2017.05.044
Xie, Z., Zhang, C., He, X., Liang, Y., Meng, D., Wang, J., et al. (2019). Iron and nickel mixed oxides derived from Ni(II)Fe(II)-PBA for oxygen evolution electrocatalysis. Front. Chem. 7:539. doi: 10.3389/fchem.2019.00539
Xiong, M., and Ivey, D. G. (2018). Synthesis of bifunctional catalysts for metal-air batteries through direct deposition methods. Batter. Supercaps 2, 326–335. doi: 10.1002/batt.201800069
Yang, Y., Lin, Z., Gao, S., Su, J., Lun, Z., Xia, G., et al. (2016). Tuning electronic structures of nonprecious ternary alloys encapsulated in graphene layers for optimizing overall water splitting activity. ACS Catal. 7, 469–479. doi: 10.1021/acscatal.6b02573
Yu, L., Yi, Q., Yang, X., and Chen, Y. (2019). An easy synthesis of Ni-Co doped hollow C-N tubular nanocomposites as excellent cathodic catalysts of alkaline and neutral zinc-air batteries. Sci. China Mater. 62, 1251–1264. doi: 10.1007/s40843-019-9439-9
Zhang, J., Wang, T., Pohl, D., Rellinghaus, B., Dong, R., Liu, S., et al. (2016). Interface engineering of MoS2/Ni3S2 heterostructures for highly enhanced electrochemical overall-water-splitting activity. Angew. Chem. Int. Ed. 55, 6702–6707. doi: 10.1002/anie.201602237
Zhang, L., Chang, C., Hsu, C.-W., Chang, C.-W., and Lu, S.-Y. (2017). Hollow nanocubes composed of well-dispersed mixed metal-rich phosphides in N-doped carbon as highly efficient and durable electrocatalysts for the oxygen evolution reaction at high current densities. J. Mater. Chem. A. 5, 19656–19663. doi: 10.1039/C7TA04905F
Keywords: zinc-air battery, integrated electrode, NiFe nanoparticle, nitrogen-doped carbon, OER/ORR
Citation: Hu H, Ling X, Tan C, Lin J, Han X and Hu W (2020) Preparation of Ni3Fe2@NC/CC Integrated Electrode and Its Application in Zinc-Air Battery. Front. Chem. 8:575288. doi: 10.3389/fchem.2020.575288
Received: 23 June 2020; Accepted: 12 October 2020;
Published: 09 November 2020.
Edited by:
Hongbo Li, Beijing Institute of Technology, ChinaReviewed by:
Khaled Mohammad Saoud, Virginia Commonwealth University School of the Arts, QatarBao Yu Xia, Huazhong University of Science and Technology, China
Copyright © 2020 Hu, Ling, Tan, Lin, Han and Hu. This is an open-access article distributed under the terms of the Creative Commons Attribution License (CC BY). The use, distribution or reproduction in other forums is permitted, provided the original author(s) and the copyright owner(s) are credited and that the original publication in this journal is cited, in accordance with accepted academic practice. No use, distribution or reproduction is permitted which does not comply with these terms.
*Correspondence: Xiaopeng Han, eHBoYW5AdGp1LmVkdS5jbg==; Wenbin Hu, d2JodUB0anUuZWR1LmNu