- Department of Chemistry and Biochemistry, California State University Northridge, Northridge, CA, United States
In this review, we summarize the rapid progress that has been made in the study of noble gas chemistry in solid compounds under high pressure. Thanks to the recent development of first-principles crystal structure search methods, many new noble gas compounds have been predicted and some have been synthesized. Strikingly, almost all types of chemical roles and interactions are found or predicted in these high-pressure noble gas compounds, ranging from cationic and anionic noble gases to covalent bonds between noble gas atoms, and to hydrogen bond-like noble gas bonds. Besides, the recently discovered He insertion reactions reveal a unique chemical force that displays no local chemical bonding, providing evidence that research into noble gas reactions can advance the frontier of chemistry at the very basic level.
Introduction
For many years after their discovery, noble gases (NG) were known as elements that do not have any chemistry. This idea was consolidated by the atomic shell structure and the corresponding theory that all elements are destined to a complete shell while forming compounds. Therefore, the noble gases would remain chemically inert (noble) since their valence orbitals are already completely filled. This doctrine was first challenged in 1933 by Pauling who predicted the formation of KrF6 and XeF6 compounds (Pauling, 1933). It took almost another 30 years before the first noble gas compound, XePtF6 was synthesized by Bartlett (1962). Soon after, many Xenon binary compounds such as XeF4, XeF2 etc. were obtained (Chernick et al., 1962). By now, there are already a few hundred known noble gas compounds and the list continues to grow (Grochala, 2007). The most recent advancements include the first truly bonded Argon compound, HArF (Khriachtchev et al., 2000), and a striking compound AuXe4 (Sb2F11) (Seidel and Seppelt, 2000) in which Xenon, a noble gas element, bonds with Au, a noble metal, as a weak reducing and coordinating agent.
A scenario of rich chemistry for NG elements has been rolled out gradually over the past decades, especially while locking them in an extreme chemical environment. For example, many NG elements can be coerced to form charged or strongly polarized species, such as HHe+ (Hogness and Lunn, 1925), HNGO− (Li et al., 2005), and HeOLi2F2, etc. (Grochala, 2012). In contrast, a similar chemical environment is difficult to achieve in solid compounds since the charge neutrality needs to be preserved globally and locally. As a result, the chemical roles that NG elements can play in solid compounds are more limited. Lots of noble gas compounds are not formed by local chemical bonds featuring electron sharing or transfer. In many of these compounds, noble gases are either bonded to other atoms by weak interactions such as van der Waals force or inserted into the voids preexisting in some solid compounds such as clathrates, C60, etc. (Saunders et al., 1994; Guńka et al., 2015). While forming true chemical bonds in compounds, such as XeF2, XeO3, etc., noble gases act like reductants (electron donors).
On the other hand, we can drive chemical interactions to an extreme in solid compounds by applying mechanical pressures so that new chemistry can emerge. Due to both the development of first-principles computer simulations (Zhang et al., 2017; Oganov et al., 2019; Miao et al., 2020) and the diamond anvil cell (DAC) experiments (Mao et al., 2018), numerous novel compounds have been predicted and some have been synthesized. These new compounds under pressure, such as NamCln (Zhang et al., 2013), H3S (Drozdov et al., 2015), LaHn (Pickard et al., 2020), CsFn (Miao, 2013), etc., show a distinct trend of having a large range of compositions, with very different stoichiometries to the ambient condition. Although many unconventional stoichiometries are caused by the formation of homonuclear bonds or species, such as Cl–Cl in NaCl3, some compounds with atypical compositions are formed due to the change of oxidation states of their constituent elements (Miao et al., 2020). In some extreme cases, the core electrons, such as the 5p electrons of Cs, can be coerced to form chemical bonds, leading to the formation of atypical compounds, such as CsF3 and CsF5 (Miao, 2013).
For the same reason, pressure can greatly enrich noble gas chemistry. It goes far beyond the known NG compounds formed by sharing their closed-shell electrons with strong oxidants such as F. In contrast to molecular and ionic species, most of the solid NG compounds under pressure are thermodynamically stable. In this review, we will show that NG elements, under high-pressure, can (1) be oxidized by elements such as Fe that usually are not considered as oxidants, (2) become an oxidant themselves and behave like anions in compounds, (3) form strong NG–NG covalent bonds, (4) form intermolecular NG bonds that are similar to hydrogen bonds, and (5) form stable compounds that are not bound by any local chemical bonds.
Methods and the Progress of High-Pressure Chemistry
The progresses of high-pressure chemistry strongly depend on the development of experimental methods. The first leap of this field is triggered by the development of diamond anvil cell (DAC) and the corresponding heating and measurement techniques. However, high-pressure experiments are usually very difficult, expensive, and time-consuming. Recently, the density functional theory (DFT) calculations have been widely used in predicting phase diagrams of binary compounds under pressure, which gave rise to the second leap of high-pressure chemistry. These “complete ab initio” studies neither use any empirical parameters for electronic structure nor take any crystal structures and chemical bonding information as input. Instead, crystal structures are generated and the globally stable structures are searched, using various algorithms, such as random search (RS) (Pickard and Needs, 2011), genetic algorithm (GA) (Glass et al., 2006; Avery et al., 2019) and particle swarm optimization (PSO) (Wang et al., 2012). In the last decade, numerous new compounds have been predicted by this method without any experimental input and many of them have been confirmed by DAC experiments. Many of these compounds assume atypical compositions, such as NaCl3, CsF3, H3S, LaH10, etc. The change of the chemistry and the formation of a plethora of atypical compounds can be roughly grouped into two kinds: those caused by the formation of homonuclear bonds and those caused by the change of oxidation states, both of which can be found in high-pressure noble gas compounds (Miao et al., 2020).
Noble Gas Chemistry Under High Pressure
Oxidation of NG Under Pressure
Although most of noble gas chemistry is about the sharing of their closed-shell electrons, oxidizing NG is not an easy task and most of the stable NG compounds contain F, the strongest oxidant element. Several Xenon oxides exist but they are not stable (Brock and Schrobilgen, 2011; Goettel et al., 2016). Pressure can greatly extend the chemistry of NG as a reductant because the energies of their valence orbitals increase rapidly under pressure and become significantly higher than those of the valence orbitals of oxidant elements. For example, DFT/GA simulations showed that XeO, XeO2, and XeO3 become stable at pressures above 83, 102, and 114 GPa (Zhu et al., 2013). A study that combined the DAC experiment and DFT simulation work added two new compositions, Xe3O2 and Xe2O5, in which Xe adopted mixed oxidation states (Dewaele et al., 2016). Similarly, Kr–O (Zaleski-Ejgierd and Lata, 2016), Xe–N (Peng et al., 2015), and Xe–C (Bovornratanaraks et al., 2019) compounds are predicted by DFT/GA, DFT/PSO, and DFT/GA methods respectively. Probably the most striking prediction is the formation of stable Xe–Fe and Xe–Ni compounds under high pressure (Zhu et al., 2014), which has been confirmed by DAC experiments (Dewaele et al., 2017; Stavrou et al., 2018). Especially, the DFT/PSO calculations showed that XeFe3 and XeNi3 become stable at the pressures and temperatures found in the Earth's core, indicating that the iron core of the Earth might be a chemical reservoir of the missing Xe (Zhu et al., 2014). From the chemistry point of view, it is significant that Xe can be oxidized by Fe or Ni under high pressure, as shown by the large calculated charge transfer from Xe to Fe/Ni in these compounds. The alloying of Xe with transition metals such as Hg has been predicted before, but DFT calculations showed only a slight charge transfer from Xe to Hg (Grochala, 2007).
Anionic Noble Gases Under Pressure
Besides extending the range of reductant chemistry, pressure can endow a new role for NG elements. They might oxidize metals such as Li and Mg and become anions in the corresponding compounds (Li et al., 2015; Miao et al., 2015; Liu et al., 2017). The first example was predicted by the DFT/PSO method, which showed that Mg forms stable compounds with Xe, Kr, and Ar under pressures higher than 125, 250, and 250 GPa, respectively (Miao et al., 2015). Among all the calculated compositions, MgNG and Mg2NG are stable under high pressure. These compounds adopt very simple structures. For Xe and Kr, MgNG adopts a Pmm (CsCl) structure, whereas Mg2NG adopts a P4/nmm structure. In contrast, both MgAr and Mg2Ar compounds adopt hexagonal P63/mcm structures. The most important chemical feature of these compounds is the charge transfer. As calculated by Bader's Quantum Theory of Atoms in Molecules method (Bader, 1990), there are large charges transferred from Mg to NG, which also strongly depend on the pressure (Miao et al., 2015). For example, the charge transfer from Mg to Xe in MgXe under 100 GPa is 1.5 e/Mg, which is comparable to that in MgO under ambient conditions. From the band structures, these compounds are clearly metallic. The projected density of states (PDOS) reveals that the transferred electrons occupy the Xe 5d orbitals (Miao et al., 2015). Therefore, under high pressure, Xe behaves like a 5d transition metal. The electron localization function (ELF) (Silvi and Savin, 1994) calculations also show large values between Mg and NG, which is also the characteristic of intermetallic compounds. A similar phenomenon has not been found or predicted in any case without compression. The closest sign of anionic NG is a theoretical study that shows a positive electron affinity for Oganesson (Gaston et al., 2002), a synthetic element that has a half-life of about 1 ms.
The Mg-rich compounds show another unique feature in their electronic structures. The charges on Mg and NG do not add up to 0. For example, at 50 GPa, the charges on Xe and Mg are −1.03e/Xe and 1.29e/Mg. There are about 1.54e which are not located on either Mg or Xe (Miao et al., 2015). Both the charge distribution and the ELF plots show that these charges locate at the interstitial sites between Mg and NG atoms (Figures 1A,B). Therefore, Mg2NG is a high-pressure electride (HPE). Electrides are compounds in which some electrons detach from all the atoms and locate at the interstitial sites, playing the role of anions (Dawes et al., 1986). The formation of electrides under high pressure can be explained by the energy change of a local orbital constrained at an interstitial site by the surrounding atoms (Miao and Hoffmann, 2014). Although its energy increases due to the reduced volume under increasing pressure, it may change less significantly than the energies of many atomic orbitals. A series of calculations of these orbital energy changes using a He-matrix model showed that the local orbital energy of the interstitial quasi-atoms (ISQ) decreases relative to that of s and p orbitals with a rate that strongly depends on the atom and the orbital (Miao and Hoffmann, 2014). HPE can form while the energy of ISQ becomes significantly lower than the energy of valence orbitals of the atom, for example, Li and Na. Mg metal has been predicted to become an HPE under pressures higher than 800 GPa (Li et al., 2010). The insertion of NG atoms into the Mg lattice while forming Mg–Xe compounds significantly lowers the pressure of forming HPE.
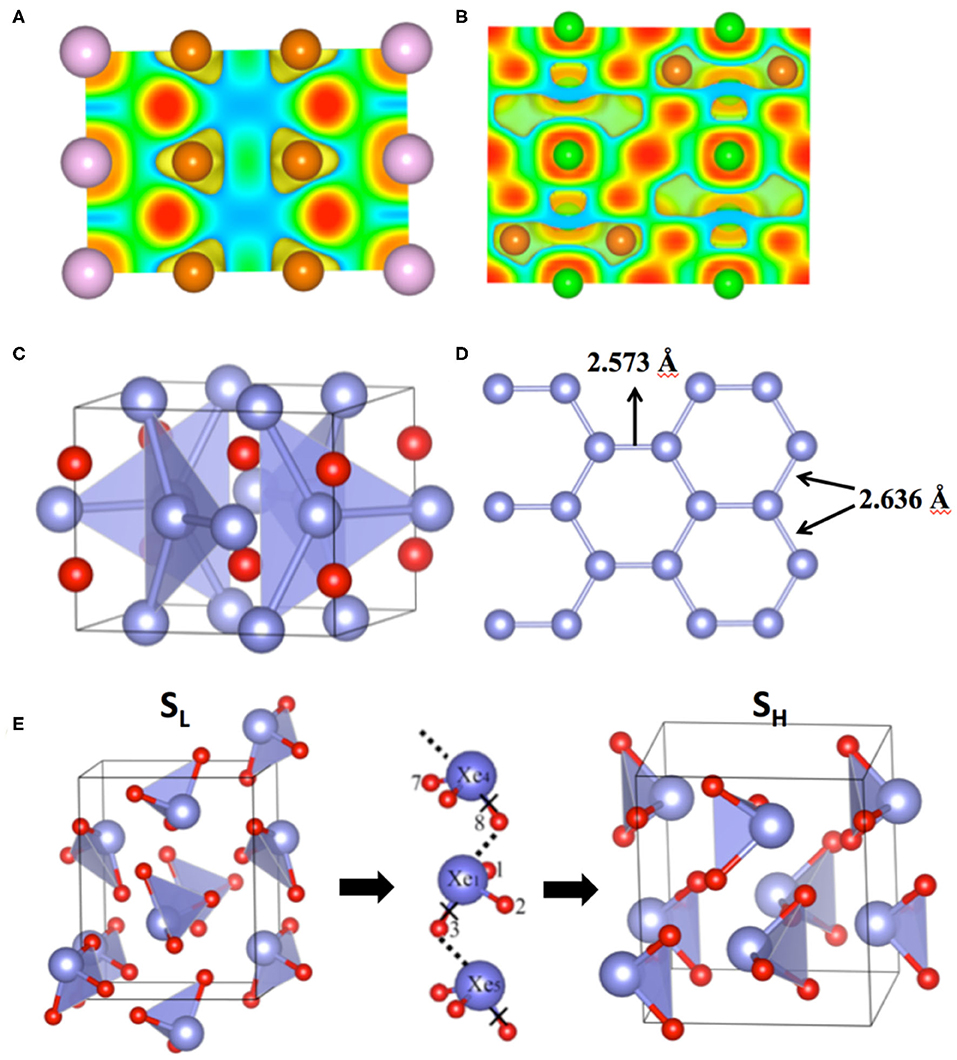
Figure 1. Electronic structure and geometry of compounds containing anionic noble gases, NG–NG covalent bonds and NG bonds. (A) Electron localization function (ELF) of Mg2Kr at 200 GPa; (B) ELF of Mg2Ar at 200 GPa; (C) Structure of Xe2F at 200 GPa; (D) The view of one set of Xe atoms in a graphene-like lattice in Xe2F. The bond lengths are slightly different due to the distortion. (E) The low pressure (SL) and the high pressure (SH) structures of XeO3 and the O path for the transition from SL to SH or vice versa. Brown, purple and green balls represent Mg, Xe and Ar atoms in (A) and (B). Blue and red balls represent Xe and F (or O) atoms in (C–E).
NG–NG Covalent Bonds in Simple Compounds
The examples of chemical bonds between NG atoms are rare. In principle, they do not form bonds because their valence orbitals are completely filled. However, while one or both NG atoms lose electrons, they may form bonds. Examples of this kind include the Xe–Xe bonds in molecules HXeXeR and RXeXeR′ (R,R′=F, Cl, Br, I) (Fernández and Frenking, 2012), and the Xe2+ cations that occur in (Sb4F21)−. (Drews and Seppelt, 1997) More recently, an example of the Xe–Xe bond was found unexpectedly in novel Xe–F compounds under pressure (Peng et al., 2016). An experimental study of XeF2 under compression showed that the XeF2 molecular crystal transformed into 2D and 3D extended solids and become metallic (Kim et al., 2010). However, the later DFT study did not agree with the proposed structure evolution of XeF2 under pressure, and therefore cannot explain the observed metallization of the compound (Kurzydlowski et al., 2011). This discrepancy was alleviated by a full-scale computation study of the Xe–F compounds with various compositions (Peng et al., 2016). The PSO based structure search revealed that XeF2 becomes unstable and decomposes to Xe2F and XeF4 at 81 GPa (Peng et al., 2016). DFT calculations using HSE functional show that XeF2 maintains its energy gap at least up to 100 GPa, whereas Xe2F is metallic. The observed insulator-metal transition of XeF2 at 70 GPa might be caused by the partial decomposition of the sample. The metallic transition was not observed in a later experiment where pressure is applied up to 80 GPa without heating the sample (Wu et al., 2017). Throughout its stable pressure range (60–200 GPa), Xe2F adopts an I4/mcm structure (Figure 1C) consisting of intercalated Xe graphitic (graphene-like) layers (Figure 1D) (Peng et al., 2016). At 200 GPa, the Xe–Xe distances are 2.573 and 2.636 Å (Figure 1D), which is close to the summation of the covalent radius of two Xe atoms. The calculated COHP and ELF prove that the two neighboring Xe atoms form covalent bonds (Peng et al., 2016). The appearance of Xe–Xe bonds in a simple binary compound is due to the enhancement of the homonuclear bond strength under pressure (Miao et al., 2020). It causes the instability of XeF2, a stoichiometric compound consisting of Xe in its typical oxidation state of +2.
Noble Gas Bonds
Another type of chemical interaction that has been missing in noble gas chemistry is the donor-acceptor weak interaction between molecules, which is similar to hydrogen bonds (Pauling, 1960) Although this type of bond is the strongest for hydrogen, especially while H atoms are bonded with strong oxidant elements such as F and O and become very electrophilic, it has been found for other elements. It was extended to halogens where they are named halogen bonds (Cavallo et al., 2016), and then to chalcogens, pnictogens, etc. (Cavallo et al., 2016). Up till recently, almost all groups of elements in the periodic table have been found to form this type of bond, except noble gases. Recently, Bauzá and Frontera (2015) studied the molecular electrostatic potential surface of XeO3 and showed that there was an unexpectedly positive potential at the position of the lone pair of Xe6+, indicating that Xe are very electrophilic while in a high oxidation state and can form a noble gas bond. Similar bonding features are also found in XeO3 and alkylnitrile adducts (Goettel et al., 2016).
Under increasing pressure, molecular crystals bound by hydrogen bonds behave very differently to molecular crystals without it. In the latter case, the lengths of the covalent intramolecular bonds, such as C–H bonds in CH4, decrease while the intermolecular distances are reduced by pressure. In contrast, the lengths of some intramolecular bonds, such as H–O in H2O, increases under compression, if the hydrogen bonds dominate the intermolecular interactions. In accordance with this change, some vibration modes are softened by the external pressure, opposing our chemical intuition that all vibration frequencies should increase while the material is compressed. Therefore, the change of bond lengths and vibration modes under pressure can be used to demonstrate the presence of hydrogen bonds. The same idea can be applied to noble gas bonds. As shown by a recent computational work of Hou et al. (2017), under increasing pressure, the Xe–O bond lengths of both SL and SH increase, and the vibration frequencies of SH decrease. Furthermore, the strong noble gas bonds between XeO3 molecules under pressure might provide transition paths for O atoms from one Xe to a neighboring Xe, a process that is essential for the structural transitions between SL and SH (Figure 1E) (Hou et al., 2015).
Forming Compounds While Keeping Nobility
Being the second most abundant element in the universe, Helium has the highest ionization energy of 24.59 eV and a negative electron affinity. Thus, He shows much less chemistry than most other elements in the periodic table. Yet, several chemical species have been predicted or synthesized by locking He in an unusual chemical environment by exquisitely designed molecular scaffolding (Hogness and Lunn, 1925; Hotokka et al., 1984; Li et al., 2005; Rzepa, 2010; Grochala, 2012). In contrast, the chemistry of He in solid compounds is almost a blank slate except the insertion of He into solid compounds with clathrate or cage structures (Saunders et al., 1994; Guńka et al., 2015). Up till very recently, there is no known reaction of He that can form a stable solid compound. The first example of such is proposed by a thorough structure search study of elements in the periodic table reacting with He under pressure and confirmed by a DAC experiment. Most of the elements were found not to react with He except Na that will form a stable Na2He compound under pressure higher than 113 GPa (Dong et al., 2017) The enthalpy of formation is as large as a 0.35 eV/atom at a pressure of 350 GPa. Such a large energy gain during the reaction excluded the possibility that Na2He is bound by weak interactions such as vdW. On the other hand, electronic structure analysis did not show any evidence that He formed chemical bonds with neighboring Na atoms, which immediately give rise to a question: how can He form a stable compound without forming chemical bonds (Miao, 2017)?
The answer to this paradox lies in the unusual behavior of electrons in Na under pressure. At pressures higher than 200 GPa, Na undergoes a structural transition and becomes transparent due to the presence of a large band-gap (Ma et al., 2009). In this double-hexagonal closed-packed structure, the valence electrons of Na detach from all the Na atoms and locate at the interstitial sites and play the role of anions. Electron analysis showed that Na2He is also an HPE (Dong et al., 2017), although Na atoms form a simple cubic lattice in Na2He. Therefore, the reaction can be viewed as the insertion of He into the Na2E ionic compound. Indeed, soon after the discovery of Na2He, the reaction of He with several other ionic compounds such as Na2O (Dong et al., 2017), Na2S (Gao et al., 2019), H2O (Liu et al., 2015), etc., have been predicted by DFT calculations. Similar to Na2He, He does not form chemical bonds with neighboring atoms in these compounds.
The driving force of He insertion reactions is electrostatic (Liu et al., 2018; Bai et al., 2019). The key point is that all the above ionic compounds involved in He insertion possess unequal numbers of cations and anions, although the overall charge is neutral. The mechanism can be explained more easily by a one-dimensional model (Figure 2A) (Liu et al., 2018). While reacting with the AB type of ionic compounds, He needs to be inserted in between two ions with opposite charges and therefore increase the electrostatic (Madelung) energy. In contrast, if the ionic compound is an A2B (or AB2) type, He atoms can choose to stay in between two ions (such as A+) with the same charge and therefore lower the Madelung energy. The two A+ ions repel each other, but are forced to stay close by external pressure. The insertion of the He in between two A+ ions alleviates this pressure effect, therefore the insertion of He in this type of ionic compounds becomes favored under increasing pressure. Thus, the reaction does not involve the formation of any local chemical bond, i.e., He can react with ionic compounds while keeping its chemical inertness (nobility). This mechanism has been demonstrated by rigorous energy analysis for He insertion into MgO (AB type) and MgF2 (AB2 type) compounds (Figures 2B–G) (Liu et al., 2018).
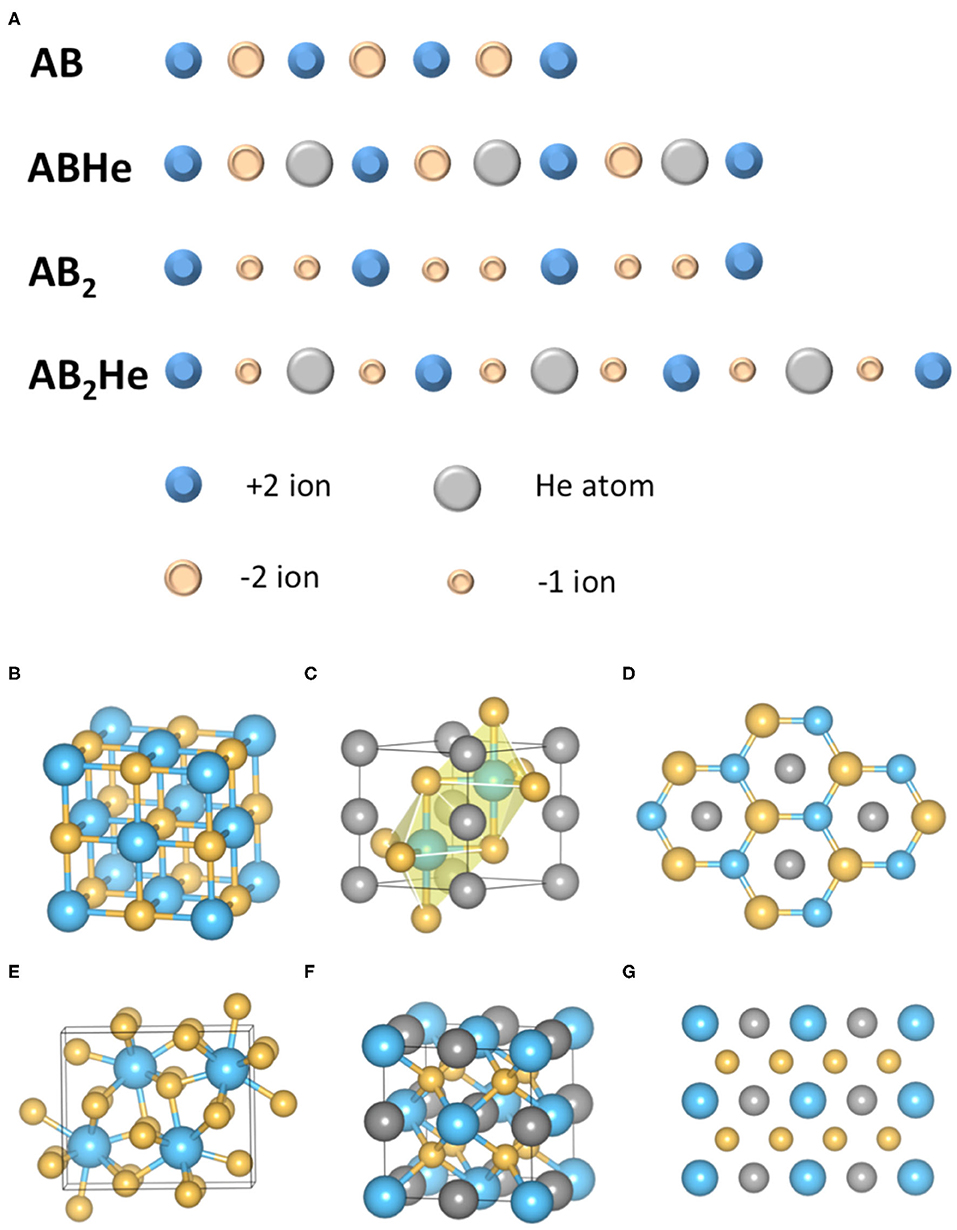
Figure 2. Mechanism of He insertion reaction with ionic compounds. (A) The schematics of He insertions into AB and AB2 types of ionic compounds. The Madelung energy increases in the former case whereas decreases in the latter case; (B) The rocksalt structure of MgO under high pressure; (C) The lowest energy structure of conceived compound MgOHe; (D) The view from (001) direction of MgOHe structure, showing that He chains are inserted between Mg and O atoms; (E) The PbCl2 structure of MgF2 under pressure; (F) The structure of stable MgF2He compound; (G) The (110) plane of MgF2He structure. The He atoms are inserted in between the neighboring F atoms, which further demonstrated the proposed mechanism. The blue, yellow and grey balls represent Mg, O (or F) and He atoms in (B–G).
Conclusions and Perspectives
Many recent simulations and experiments showed that noble gases could have very rich chemistry under high pressure. The types of chemical roles and interactions include electron donors (being oxidizing), electron acceptors (being reduced), NG–NG covalent bonds, noble gas bonds, and reliever of repulsive electrostatic interactions. A major effect of pressure is the change of the energies of the atomic orbitals. Although the energies of all local orbitals increase under higher pressure, the effect is more significant to those orbitals with lower principal quantum numbers and higher angular momenta, especially to those having no corresponding core orbitals such as 2p and 3d. As a result of this orbital energy reordering, the electrons are redistributed in different quantum orbitals under pressure. If the energy of the valence np orbital of a noble gas (5p for Xe, 4p for Kr) is close to or higher than the energies of the valence orbitals of an oxidant element such as F, O, or Fe, the noble gas will be oxidized. Conversely, if the unoccupied orbitals of the noble gases such as 5d for Xe become lower in energy than the orbitals of reductants such as Li or Mg, the noble gases will be reduced and become anionic.
The future of the exotic chemistry of noble gases as well as the high-pressure study rely on three signs of progress, the experimental methods that allow us to study the chemistry of materials under higher pressure (>200 GPa), the computer power and the simulation algorithms that can enable us to explore the structures and stability of more complicated materials such as ternary and quaternary compounds, the conceptual framework that can help us in understanding and predicting the change of chemistry under pressure without full-scale calculations. Although the recent high-pressure studies have greatly advanced noble gas chemistry, many important questions still remain unanswered. What are the oxidation and reduction limits of noble gases? Can He or Ne be oxidized or reduced under pressure? Can high-pressure noble gas compounds become superconducting or topological? Can high-pressure noble gas bonding be recovered after releasing the pressure? The developments of theoretical, simulation, and experimental methods might help to answer these questions and extend the noble gas chemistry to an unexpected territory.
Author Contributions
The author confirms being the sole contributor of this work and has approved it for publication.
Funding
The author acknowledges the support of NSF CAREER award 1848141, ACS PRF 59249-UNI6, and computational resources provided by XSEDE (TG-DMR130005).
Conflict of Interest
The author declares that the research was conducted in the absence of any commercial or financial relationships that could be construed as a potential conflict of interest.
References
Avery, P., Toher, C., Curtarolo, S., and Zurek, E. (2019). XtalOpt Version R12: an open-source evolutionary algorithm for crystal structure prediction. Comput. Phys. Commun. 237, 274–275. doi: 10.1016/j.cpc.2018.11.016
Bai, Y., Liu, Z., Botana, J., Yan, D., Lin, H.-Q., Sun, J., et al. (2019). Electrostatic force driven helium insertion into ammonia and water crystals under pressure. Commun Chem. 2, 1–7. doi: 10.1038/s42004-019-0204-6
Bartlett, N. (1962). Xenon Hexafluoroplatinate (V) Xe+PtF6. In: Proceedings of the Chemical Society of London (London).
Bauzá, A., and Frontera, A. (2015). Aerogen bonding interaction: a new supramolecular force? Angew. Chem. Int. Ed. 54, 7340–7343. doi: 10.1002/anie.201502571
Bovornratanaraks, T., Tsuppayakorn-aek, P., Luo, W., and Ahuja, R. (2019). Ground-state structure of semiconducting and superconducting phases in xenon carbides at high pressure. Sci. Rep. 9, 1–6. doi: 10.1038/s41598-019-39176-4
Brock, D. S., and Schrobilgen, G. J. (2011). Synthesis of the missing oxide of xenon, XeO2, and its implications for earth's missing xenon. J. Am. Chem. Soc. 133, 6265–6269. doi: 10.1021/ja110618g
Cavallo, G., Metrangolo, P., Milani, R., Pilati, T., Priimagi, A., Resnati, G., et al. (2016). The halogen bond. Chem. Rev. 116, 2478–2601. doi: 10.1021/acs.chemrev.5b00484
Chernick, C. L., Claassen, H. H., Fields, P. R., Hyman, H. H., Malm, J. G., Manning, W. M., et al. (1962). Fluorine compounds of xenon and radon. Science. 138, 136–138. doi: 10.1126/science.138.3537.136
Dawes, S. B., Ward, D. L., Huang, R. H., and Dye, J. L. (1986). First electride crystal structure. J. Am. Chem. Soc. 108, 3534–3535. doi: 10.1021/ja00272a073
Dewaele, A., Pépin, C. M., Geneste, G., and Garbarino, G. (2017). Reaction between nickel or iron and xenon under high pressure. High Press. Res. 37, 137–146. doi: 10.1080/08957959.2016.1267165
Dewaele, A., Worth, N., Pickard, C. J., Needs, R. J., Pascarelli, S., Mathon, O., et al. (2016). Synthesis and stability of xenon oxides Xe2O5 and Xe3O2 under pressure. Nat. Chem. 8, 784–790. doi: 10.1038/nchem.2528
Dong, X., Oganov, A. R., Goncharov, A. F., Stavrou, E., Lobanov, S., Saleh, G., et al. (2017). A stable compound of helium and sodium at high pressure. Nat. Chem. 9, 440–445. doi: 10.1038/nchem.2716
Drews, T., and Seppelt, K. (1997). The Xe ion-preparation and structure. Angew. Chem. Int. Ed. Eng. 36, 273–274. doi: 10.1002/anie.199702731
Drozdov, A. P., Eremets, M. I., Troyan, I. A., Ksenofontov, V., and Shylin, S. I. (2015). Conventional superconductivity at 203 kelvin at high pressures in the sulfur hydride system. Nature. 525, 73–76. doi: 10.1038/nature14964
Fernández, I., and Frenking, G. (2012). Neutral noble gas compounds exhibiting a Xe–Xe bond: structure, stability and bonding situation. Phys. Chem. Chem. Phys. 14, 14869–14877. doi: 10.1039/c2cp41244f
Gao, H., Sun, J., Pickard, C. J., and Needs, R. J. (2019). Prediction of pressure-induced stabilization of noble-gas-atom compounds with alkali oxides and alkali sulfides. Phys. Rev. Mater. 3, 015002. doi: 10.1103/PhysRevMaterials.3.015002
Gaston, N., Schwerdtfeger, P., and Nazarewicz, W. (2002). Ionization potentials of internal conversion electrons for the superheavy elements 112, 114, 116, and 118. Phys. Rev. A. 66, 062505. doi: 10.1103/PhysRevA.66.062505
Glass, C. W., Oganov, A. R., and Hansen, N. (2006). USPEX - evolutionary crystal structure prediction. Comput. Phys. Commun. 175, 713–720. doi: 10.1016/j.cpc.2006.07.020
Goettel, J. T., Matsumoto, K., Mercier, H. P. A., and Schrobilgen, G. J. (2016). Syntheses and structures of xenon trioxide alkylnitrile adducts. Angew. Chem. Int. Ed. 55, 13780–13783. doi: 10.1002/anie.201607583
Grochala, W. (2007). Atypical compounds of gases, which have been called “noble.” Chem. Soc. Rev. 36, 1632–1655. doi: 10.1039/b702109g
Grochala, W. (2012). A metastable He-O bond inside a ferroelectric molecular cavity: (HeO)(LiF)(2). Phys. Chem. Chem. Phys. 14, 14860–14868. doi: 10.1039/c2cp42321a
Guńka, P. A., Dziubek, K. F., Gładysiak, A., Dranka, M., Piechota, J., Hanfland, M., et al. (2015). Compressed arsenolite As4O6 and its helium clathrate As4O6·2He. Cryst. Growth Des. 15, 3740–3745. doi: 10.1021/acs.cgd.5b00390
Hogness, T. R., and Lunn, E. G. (1925). The ionization of hydrogen by electron impact as interpreted by positive ray analysis. Phys. Rev. 26, 44–55. doi: 10.1103/PhysRev.26.44
Hotokka, M., Kindstedt, T., Pyykkö, P., and Roos, B. O. (1984). On bonding in transition-metal helide ions. Mol. Phys. 52, 23–32. doi: 10.1080/00268978400101031
Hou, C., Wang, X., Botana, J., and Miao, M. (2017). Noble gas bond and the behaviour of XeO3 under pressure. Phys. Chem. Chem. Phys. 19, 27463–27467. doi: 10.1039/C7CP05385A
Hou, P., Tian, F., Li, D., Zhao, Z., Duan, D., Zhang, H., et al. (2015). Ab initio study of germanium-hydride compounds under high pressure. RSC Adv. 5, 19432–19438. doi: 10.1039/C4RA13183E
Khriachtchev, L., Pettersson, M., Runeberg, N., Lundell, J., and Rasanen, M. (2000). A stable argon compound. Nature. 406, 874–876. doi: 10.1038/35022551
Kim, M., Debessai, M., and Yoo, C. S. (2010). Two- and three-dimensional extended solids and metallization of compressed XeF2. Nat. Chem. 2, 784–788. doi: 10.1038/nchem.724
Kurzydlowski, D., Zaleski-Ejgierd, P., Grochala, W., and Hoffmann, R. (2011). Freezing in resonance structures for better packing: XeF2 becomes (XeF+)(F-) at large compression. Inorg. Chem. 50, 3832–3840. doi: 10.1021/ic200371a
Li, P., Gao, G., Wang, Y., and Ma, Y. (2010). Crystal structures and exotic behavior of magnesium under pressure. J. Phys. Chem. C. 114, 21745–21749. doi: 10.1021/jp108136r
Li, T. H., Mou, C. H., Chen, H. R., and Hu, W. P. (2005). Theoretical prediction of noble gas containing anions FNgO- (Ng = He, Ar, and Kr). J. Am. Chem. Soc. 127, 9241–9245. doi: 10.1021/ja051276f
Li, X., Hermann, A., Peng, F., Lv, J., Wang, Y., Wang, H., et al. (2015). Stable lithium argon compounds under high pressure. Sci. Rep. 5, 16675. doi: 10.1038/srep16675
Liu, H. Y., Yao, Y. S., and Klug, D. D. (2015). Stable structures of He and H2O at high pressure. Phys. Rev. B. 91:014102. doi: 10.1103/PhysRevB.91.014102
Liu, Z., Botana, J., Hermann, A., Valdez, S., Zurek, E., Yan, D., et al. (2018). Reactivity of He with ionic compounds under high pressure. Nat. Commun. 9, 951. doi: 10.1038/s41467-018-03284-y
Liu, Z., Botana, J., Miao, M. S., and Yan, D. D. (2017). Unexpected Xe Anions in XeLin intermetallic compounds. Europhys Lett. 117, 26002. doi: 10.1209/0295-5075/117/26002
Ma, Y., Eremets, M., Oganov, A. R., Xie, Y., Trojan, I., Medvedev, S., et al. (2009). Transparent dense sodium. Nature. 458, 182–185. doi: 10.1038/nature07786
Mao, H. K., Chen, X. J., Ding, Y., Li, B., and Wang, L. (2018). Solids, liquids, and gases under high pressure. Rev. Mod. Phys. 90, 015007. doi: 10.1103/RevModPhys.90.015007
Miao, M. (2017). Helium chemistry: react with nobility. Nat. Chem. 9, 409–410. doi: 10.1038/nchem.2768
Miao, M., Sun, Y., Zurek, E., and Haiqing, L. (2020). Chemistry under high pressure. Nat. Rev. Chem. 4, 508–527. doi: 10.1038/s41570-020-0213-0
Miao, M.-S., and Hoffmann, R. (2014). High Pressure electrides: a predictive chemical and physical theory. Acc. Chem. Res. 47, 1311–1317. doi: 10.1021/ar4002922
Miao, M. S. (2013). Caesium in high oxidation states and as a P-block element. Nat. Chem. 5, 846–852. doi: 10.1038/nchem.1754
Miao, M. S., Wang, X. L., Brgoch, J., Spera, F., Jackson, M. G., Kresse, G., et al. (2015). Anionic chemistry of noble gases: formation of Mg-NG (NG = Xe, Kr, Ar) compounds under pressure. J. Am. Chem. Soc. 137, 14122–14128. doi: 10.1021/jacs.5b08162
Oganov, A. R., Pickard, C. J., Zhu, Q., and Needs, R. J. (2019). Structure prediction drives materials discovery. Nature Reviews Materials. 4, 331–348. doi: 10.1038/s41578-019-0101-8
Pauling, L. (1933). The formulas of antimonic acid and the antimonates. J. Am. Chem. Soc. 55, 1895–1900. doi: 10.1021/ja01332a016
Peng, F., Botana, J., Wang, Y., Ma, Y., and Miao, M. (2016). unexpected trend in stability of Xe-F compounds under pressure driven by Xe-Xe covalent bonds. J. Phys. Chem. Lett. 7, 4562–4567. doi: 10.1021/acs.jpclett.6b01922
Peng, F., Wang, Y. C., Wang, H., Zhang, Y. W., and Ma, Y. M. (2015). Stable xenon nitride at high pressures. Phys. Rev. B. 92, 094104. doi: 10.1103/PhysRevB.92.094104
Pickard, C. J., Errea, I., and Eremets, M. I. (2020). Superconducting hydrides under pressure. Annu. Rev. Condens. Matter Phys. 11, 57–76. doi: 10.1146/annurev-conmatphys-031218-013413
Pickard, C. J., and Needs, R. J. (2011). Ab initio random structure searching. J. Phys. Condens. Matter. 23, 053201. doi: 10.1088/0953-8984/23/5/053201
Rzepa, H. S. (2010). The rational design of helium bonds. Nat. Chem. 2, 390–393. doi: 10.1038/nchem.596
Saunders, M., Jimenez-Vazquez, H. A., Cross, R. J., Mroczkowski, S., Gross, M. L., Giblin, D. E., et al. (1994). Incorporation of helium, neon, argon, krypton,and xenon into fullerenes using high pressure. J. Am. Chem. Soc. 116, 2193–2194. doi: 10.1021/ja00084a089
Seidel, S., and Seppelt, K. (2000). Xenon as a complex ligand: the tetra xenono gold(II) cation in (Sb2)2. Science. 290, 117–118. doi: 10.1126/science.290.5489.117
Silvi, B., and Savin, A. (1994). Classification of chemical bonds based on topological analysis of electron localization functions. Nature. 371, 683–686. doi: 10.1038/371683a0
Stavrou, E., Yao, Y., Goncharov, A. F., Lobanov, S. S., Zaug, J. M., Liu, H., et al. (2018). Synthesis of xenon and iron-nickel intermetallic compounds at earth's core thermodynamic conditions. Phys. Rev. Lett. 120, 096001. doi: 10.1103/PhysRevLett.120.096001
Wang, Y., Lv, J., Zhu, L., and Ma, Y. (2012). CALYPSO: a method for crystal structure prediction. Comput. Phys. Commun. 183, 2063–2070. doi: 10.1016/j.cpc.2012.05.008
Wu, G., Huang, X., Huang, Y., Pan, L., Li, F., Li, X., et al. (2017). Confirmation of the structural phase transitions in XeF2 under high pressure. J. Phys. Chem. C. 121, 6264–6271. doi: 10.1021/acs.jpcc.6b11558
Zaleski-Ejgierd, P., and Lata, P. M. (2016). Krypton oxides under pressure. Sci. Rep. 6, 18938. doi: 10.1038/srep18938
Zhang, L., Wang, Y., Lv, J., and Ma, Y. (2017). Materials Discovery at high pressures. Nat. Rev. Mater. 2. doi: 10.1038/natrevmats.2017.5
Zhang, W., Oganov, A. R., Goncharov, A. F., Zhu, Q., Boulfelfel, S. E., Lyakhov, A. O., et al. (2013). Unexpected stable stoichiometries of sodium chlorides. Science. 342, 1502–1505.
Zhu, L., Liu, H., Pickard, C. J., Zou, G., and Ma, Y. (2014). Reactions of xenon with iron and nickel are predicted in the earth's inner core. Nat. Chem. 6, 644–648. doi: 10.1038/nchem.1925
Keywords: noble gas anions, NG–NG bonds, noble gas bond, chemistry without chemical bond, DFT—density functional theory, high pressure
Citation: Miao M (2020) Noble Gases in Solid Compounds Show a Rich Display of Chemistry With Enough Pressure. Front. Chem. 8:570492. doi: 10.3389/fchem.2020.570492
Received: 08 June 2020; Accepted: 16 September 2020;
Published: 05 November 2020.
Edited by:
Sudip Pan, University of Marburg, GermanyReviewed by:
Wojciech Grochala, University of Warsaw, PolandXiang-Feng Zhou, Yanshan University, China
Copyright © 2020 Miao. This is an open-access article distributed under the terms of the Creative Commons Attribution License (CC BY). The use, distribution or reproduction in other forums is permitted, provided the original author(s) and the copyright owner(s) are credited and that the original publication in this journal is cited, in accordance with accepted academic practice. No use, distribution or reproduction is permitted which does not comply with these terms.
*Correspondence: Maosheng Miao, bW1pYW9AY3N1bi5lZHU=