- 1Sonophotochemistry Research Group, Faculty of Science and Natural Resources, Universiti Malaysia Sabah, Kota Kinabalu, Sabah
- 2Water Research Unit, Faculty of Science and Natural Resources, Universiti Malaysia Sabah, Kota Kinabalu, Sabah
- 3Industrial Chemistry Programme, Faculty of Science and Natural Resources, Universiti Malaysia Sabah, Kota Kinabalu, Sabah
- 4Chancellery Office, Universiti Malaysia Sabah, Kota Kinabalu, Sabah
- 5Catalysis Science and Technology Research Centre, Faculty of Science, Universiti Putra Malaysia, Serdang, Malaysia
- 6Centre of Foundation, Language and Malaysian Studies, International University of Malaya-Wales, Kuala Lumpur, Malaysia
Over the last decade, interest in the utilization of solar energy for photocatalysis treatment processes has taken centre-stage. Researchers had focused on doping TiO2 with SiO2 to obtain an efficient degradation rate of various types of target pollutants both under UV and visible-light irradiation. In order to further improve this degradation effect, some researchers resorted to incorporate plasmonic metal nanoparticles such as silver and gold into the combined TiO2-SiO2 to fully optimize the TiO2-SiO2’s potential in the visible-light region. This article focuses on the challenges in utilizing TiO2 in the visible-light region, the contribution of SiO2 in enhancing photocatalytic activities of the TiO2-SiO2 photocatalyst, and the ability of plasmonic metal nanoparticles (Ag and Au) to edge the TiO2-SiO2 photocatalyst toward an efficient solar photocatalyst.
Introduction
The first breakthrough in producing the photocatalytic effect of TiO2 was reported by Fujishima-Honda in 1972, in which, photosplitting of water in the presence of TiO2 was achieved. The team documented the generation of oxygen gas bubbles at the electrode containing TiO2 placed under an electrical contact with a piece of platinum metal. While both were immersed in water and exposed to light, hydrogen gas was detected at the platinum electrode. The explanation for this effect was that TiO2, under ultraviolet irradiation with a wavelength lower than 380 nm, produces an electron-hole pairs according to the following equation:
The electron-hole pairs diffuse evenly on the surface of the TiO2 particle, to react and decompose oxygen and water present in the atmosphere in order to produce HO•, hydroxyl radicals, and O−2, superoxide ions, according to the following equations:
These oxidants will disintegrate and restructure the pollutants through redox reactions taking place on the surface of catalyst, into H2O, CO2, and mineral acids (Zangeneh et al., 2015). The mineral acids are generated due to presence of heteroatoms, i.e., S, N, and Cl in the organic compounds (Gardin et al., 2010). TiO2, is a commonly used photocatalyst due to its availability, its efficient photoactivity, highest chemical stability, harmless nature, lowest cost, and ability in mineralizing various organic contaminants including dyes, insecticides, aromatics, alkanes, haloalkanes, alcohols, and surfactants (Gardin et al., 2010; Abdullah et al., 2016).
TiO2-based photocatalysis has long been utilized as one of the methods to remediate wastewater. The main obstruction for the application of TiO2 under solar energy is its high recombination rate of photoexcited electron-hole pairs and wide band gap which is 3.2 eV (Liu et al., 2013). Due to its wide band gap, it requires photon energy equals or higher than 3.2 eV, in order to induce the photoexcitation of electrons and holes. Unfortunately, majority of photons in visible region have photon energy less than 3.0 eV (based on Figure 1). In order to overcome these restrictions, TiO2 is prepared as a composite catalyst in which the newly added material enables the utilization of TiO2 in the visible-light region. For instance, doping CuBiS2, a p-type semiconductor (with a band-gap value of 2.19–2.62 eV) onto TiO2, enabled the direct utilization of TiO2 photocatalytic activity in the visible-light region (Abdullah & Kuo, 2015).
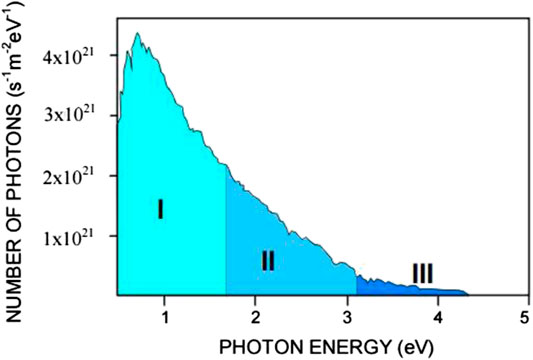
FIGURE 1. The solar energy spectrum based on the number of photons received per second per unit area of 1 m2 vs. the photon energy detected on a clear sunny day with the sun at 60° above the horizon. I: IR region, II: visible region, and III: UV region cited by Choi, 2007 which was originally adapted from Oriel-Instruments (Book of Photon Tools, Oriel-Instruments, 1999).
Another hindrance in utilizing TiO2 photocatalysis on an industrial scale is the requirement of a large amount of energy to power the UV lamps, resulting in its high operating costs (Chan et al., 2012). In order to overcome these drawbacks, employing sunlight energy to power the photoexcitation of the electrons and holes in the TiO2 semiconductor would be most ideal. The tapping of solar energy to drive the remediation of wastewater by photocatalysis processes will enable the classification of conventional photocatalysis methods as renewable energy with green technology characteristics.
Due to these benefits, many investigators are currently working on extending the application of TiO2 photocatalysis into visible-light region. In order to achieve this, one of the methods focused on is the synthesizing of SiO2-modified TiO2 photocatalysts which have shown remarkable efficiency under visible-light region.
TiO2-SiO2 Photocatalysis
Effect of SiO2 on Specific Surface Area of TiO2-SiO2 Composite
SiO2 is typically used as a catalyst support or dopant dispersed within the TiO2 lattice (Liga et al., 2013). This doping affects the TiO2’s fundamental properties and thus influences the photocatalytic activities too. The structure modification of TiO2 by SiO2 drastically increases the specific surface area of the TiO2-based photocatalyst. The high surface area of TiO2-SiO2 is due to its high porosity. Structures with high porosity have large internal surface area per weight, and it is this property which provides high accessibility and diffusivity in order to allow molecules to penetrate through pores, resulting in higher degradation of pollutants on the catalyst’s surface (Jeon et al., 2015).
The morphological improvement by SiO2 on the TiO2’s surface is evident from the literature. Balachandran et al. (2014) reported that the BET specific surface area increased from 65 m2 g−1 for TiO2 to 75 m2 g−1 for TiO2-SiO2 while the mean pore size calculated from BET isotherms was 10 nm for TiO2 and 15 nm for TiO2-SiO2. Bellardita et al. (2010) reported specific surface area of TiO2-Cabot SiO2, TiO2-Axim SiO2, and TiO2-Fly Ash SiO2 as 177, 49, and 29 m2 g−1, respectively. Fatimah et al. (2015) reported TiO2-SiO2 produced by using rice husk ash as the precursor for SiO2 with a specific surface area of 91.91 m2/g with a pore volume of 15.18 cc/g compared to that of rice husk ash with a specific surface area of 25.09 m2g−1 and pore volume of 11.60 cc g−1. These results were reportedly higher than that of TiO2-SiO2 synthesized by using tetraethyl orthosilicate (TEOS) as the SiO2 precursor, resulting in catalyst material with a specific surface area of 59.22 m2/g and pore volume of 14.92 cc/g. Table 1 shows the BET surface area and crystallite size values of TiO2-SiO2 photocatalyst reported by Ramamoorthy et al., 2016.
Data in Table 1 clearly indicate that the high surface area of TiO2-SiO2 is attributed to the high surface area of SiO2 itself. SiO2 can be synthesized easily via sol gel with a large surface area and pore volume (Ren et al., 2010; Bahadur et al., 2012) and later can be added into TiO2 sol, generating composite photocatalyst with increased surface area and pore volume. The enhanced surface area of TiO2-SiO2 definitely facilitates in achieving higher photocatalytic activity under solar irradiation.
Effect of SiO2 on Crystalline Size of TiO2-SiO2 Composite
Deposition of SiO2 onto TiO2 reduces the overall particle size of TiO2-SiO2 composite particles. Based on the spectra from the UV-Vis spectrophotometer, Balachandran et al. (2014) reported absorption peak of maximum absorbance of TiO2 and TiO2-SiO2 at 372 and 352 nm, respectively. The blue shift indicates decrease in particle size due to quantum confinement effect. As shown in Table 1, Ramamoorthy et al. (2016) reported the reduction in crystallite size of TiO2-SiO2 (9–12 nm) compared to TiO2 (18 nm). In addition, using data from the SEM and TEM analysis, Balachandran et al. (2014) reported that pure TiO2 showed irregular morphological structure due to the agglomeration of its particles and has an average diameter of 15–20 nm. Meanwhile, TiO2-SiO2 showed regular morphology with an average particle size of 7–10 nm. This proves that the SiO2-modified TiO2 photocatalyst consists of smaller particles but with larger surface area.
The reduced size of TiO2-SiO2 particles reduces the pathway in which the photoinduced electrons and holes are used to migrate to the active sites on the TiO2 surface. This increases the efficiency of the redox reactions by electrons and holes while reducing the recombination rate of photoinduced electrons and holes, thus making TiO2-SiO2 a better photocatalyst as compared to TiO2.
Effect of SiO2 on Surface Acidity of TiO2-SiO2 Composite
Many previous researchers have reported high surface acidity of TiO2-SiO2 composite which aids in enhancing the photo decomposition of pollutant molecules. According to Wei et al. (2014), TiO2 exhibits Lewis acidity and SiO2 does not exhibit any acidity while TiO2-SiO2 exhibits both Bronsted and Lewis acidity. For TiO2-SiO2 with TiO2 as the main component, the Lewis acid sites are dominant while the Bronsted acid sites are dominant for TiO2-SiO2 with SiO2 as the major component (Fateh et al., 2013). It should be noted that the Ti/Si atomic ratio can be manipulated to control the acidity of the TiO2-SiO2. TiO2-SiO2 with atomic ratio of four (Ti:Si = 4) exhibits highest Lewis acidity while TiO2-SiO2 with atomic ratio of one (Ti:Si = 1) exhibited highest Bronsted acidity (Wei et al., 2014).
The Ti-O-Si bond, a strong acidic bond, in the TiO2-SiO2 composite photocatalyst, results in a charge imbalance due to the different coordination numbers of the Ti and Si metal center. To offset the negative imbalanced charges over Ti-O, a great deal of protons is extracted from H2O molecules generating HO− groups, which in turn enhances the surface acidity. The decomposition rate of pollutant molecules is enhanced due to higher amount of hydroxyl groups being on the surface of TiO2-SiO2, representing a better photocatalytic performance in visible region compared to TiO2 (Kibombo et al., 2012; Xu et al., 2015).
The increased surface acidity attracts and adsorbs more hydroxyl groups which later act as hole-scavengers and readily oxidize the adsorbed H2O molecules. These surface hole-scavenger active sites effectively increase the charge separation and reduce the recombination of photoinduced electrons and holes (Kibombo et al., 2012).
Effect of SiO2 on Band Gap of TiO2-SiO2 Composite
One of the main drawbacks of TiO2 is the fast recombination of photoinduced electrons and holes which decreases the efficiency of its photocatalytic activity. Deposition of SiO2 onto TiO2 caused an increase in the band-gap value of the TiO2-SiO2 composite, which is due to the quantum-size effect resulting in an increase in the band-gap value and the interface interaction between the oxide phases, either an SiO2 matrix or SiO2 support effect. The interface interaction leads to a formation of Ti-O-Si bonds strongly modifying the electronic structure of the Ti atoms (Panayotov and Yates, 2003).
As an example, the band-gap value of TiO2 and TiO2-SiO2 as reported by Balachandran et al. (2014) was 3.3 and 3.54 eV, respectively. Bellardita et al. (2010) documented the band-gap value of pure TiO2, TiO2-Cabot SiO2, and TiO2/Axim SiO2 as 3.00, 3.02, and 2.98 eV, respectively. The increased band gap in TiO2-SiO2 indicates that the electrons and holes possess stronger reduction (Kibombo et al., 2012) and oxidation abilities and these abilities enhance the photocatalytic activity of the TiO2-SiO2 photocatalyst in visible region.
Effect of SiO2 on Thermal Stability of TiO2-SiO2 Composite
Addition of SiO2 onto TiO2 increases the overall thermal stability of TiO2-SiO2, thus preventing the conversion from anatase TiO2 into rutile TiO2 crystalline structure (Kibombo et al., 2012). Table 2 shows the specific surface and pore volume of CTS-1 (Ti:Si = 1), CTS-4 (Ti:Si = 4), and TiO2 as a function of the calcination temperature reported by Wei et al. (2014).

TABLE 2. Specific surface area and pore volume of CTS-1, CTS-4, and TiO2 in relation to calcination temperature (source: Wei et al. (2014)).
When the calcination temperature increased from 500 to 950°C, the specific surface area decreased from 437.4 to 176.3 m2 g−1 for CTS-1 and from 309.5 to 121.2 m2 g−1 for CTS-4. When the calcination temperature was increased from 500 to 950°C, the pore volume of CTS-1 changed from 1.39 to 0.52 ml g−1 and the pore volume of CTS-4 changed from 0.55 to 0.30 ml g−1. Meanwhile, for TiO2, both specific surface area and pore volume were undetectable after calcination at 650°C or higher due to sintering of particles and collapse of interstitial pores. However, both CTS-1 and CTS-4 are less affected by the increase in calcination temperature due to enhanced thermal stability of TiO2 with the addition of SiO2 (Wei et al., 2014).
Photocatalytic Activity of TiO2-SiO2 Composite
Binary mixed oxide, TiO2-SiO2, has been widely documented as a better photocatalyst compared to TiO2 alone due to simultaneous roles played by the TiO2 and SiO2 as photocatalyst and adsorbent, respectively, according to the reaction mechanism shown in Figure 2. The doping of SiO2 onto TiO2 enhances the adsorption of pollutant molecules to be near the photoactive center of TiO2, resulting in more pollutant molecules being broken down, thus producing high degradation rate of pollutant molecules under visible-light region. Due to the presence of more effective adsorption sites in the TiO2-SiO2 composite system, the photogenerated holes can reach the sites before recombination with electrons thus further enhancing the photocatalytic activity of TiO2.
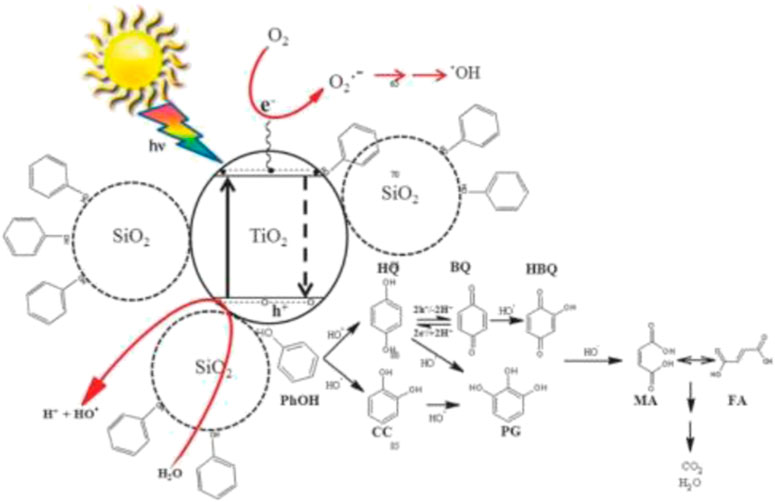
FIGURE 2. Photocatalytic degradation of phenol over TiO2-SiO2 photocatalyst (source: Kibombo et al. (2012)).
Ramamoorthy et al. (2016) reported that 30% of the TiO2 incorporated in TiO2-SiO2 (200 mg) exhibited 51% degradation of acid orange dye (300 ppm) as compared to only 19% by TiO2 (200 mg) after 10 h of visible-light irradiation (150 W tungsten filament lamp). Mungondori et al. (2015) reported an absorption band of 366 nm for TiO2 and 397 nm for TiO2-SiO2 analyzed through shift in absorption band edge. This proves that incorporation of SiO2 into TiO2 shifts the absorption band of TiO2 toward the visible-light region.
When the molar ratio of TiO2 in TiO2-SiO2 was increased, the total surface area of TiO2-SiO2 decreased causing low photocatalytic activity of the composite catalyst (Ramamoorthy et al., 2016). In a study reported by Jeon et al. (2015), at high molar ratio of TiO2 (molar ratio of TiO2 precursor to SiO2 precursor of 0.05) in the TiO2-SiO2, dense thin film with mostly blocked pores was observed. This indicated that at higher concentration of TiO2 precursor relative to SiO2 precursor, the size of the pores became smaller if not completely blocked. In contrast, at high SiO2 precursor molar ratio relative to TiO2 precursor, the amount of vertically perforated pores increased and formed large micron-sized cracks due to the inability in enduring thermal shrinkage stress as the film thickness increased. Thickness of the film has been reported to decrease with increase in molar ratio of SiO2 in TiO2/SiO2 film (Fateh et al., 2013).
When too small amount of SiO2 (<2%) or too large (>5%) is used to modify TiO2, it causes lower photocatalytic degradation of TiO2-SiO2 as compared to commercial P25 TiO2. In addition, from the XRD analysis, it was reported that doping a small amount of SiO2 on TiO2 will not effectively prevent the rutile phase transformation, which also contributes to a lower photocatalytic activity while a high amount of SiO2 doping will influence the optical absorption of TiO2 which is unfavourable in photocatalytic reactions (Kang et al., 2009). Table 3 presents the gist of the some of the previous studies on TiO2-SiO2 photocatalyst.
Challenges in TiO2-SiO2 in Solar Photocatalysis
Modification of TiO2 with SiO2 alone is insufficient to maximize the utilization of the TiO2 semiconductor in a solar-energy-powered wastewater remediation process. In order to fully utilize the photocatalytic activity of the TiO2-SiO2 in the visible region, there is a need for third material to be added to the TiO2-SiO2 in which the third material donates the electrons excited via absorption of photon from sunlight irradiation. Thus, the third material needs to have a low band-gap energy (Eg < 3) in order to utilize the energy directly from the sunlight. Xu et al. (2015) utilized Fe2O3, which has a band-gap energy of 2.2 eV, to extend the application of TiO2 in the visible-light region by coupling with Fe2O3.
Wang et al. (2016) reported on utilizing Fe2O3 coupled TiO2-SiO2 photocatalyst irradiated under room light to degrade the methyl orange dye. Based on the diffuse reflectance spectra analysis, the absorption edge of SiO2-TiO2 was approximately 400 nm. However, the absorption edge of SiO2-TiO2-Fe2O3 and SiO2-Fe2O3-TiO2 is in the visible-light region as shown in Figures 3, 4. This shift directly enables and enhances the photocatalytic activity of TiO2-based catalyst in the visible-light region due to the narrowing of the band-gap value. Compared to SiO2-TiO2-Fe2O2, SiO2-Fe2O3-TiO2 exhibited a higher photocatalytic activity in the visible region due to strong interaction between Fe2O3 and TiO2 and due to the formation of shallow trapping sites for photoinduced electrons and holes.
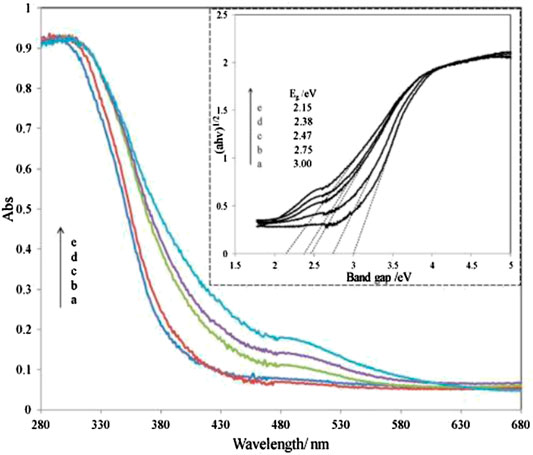
FIGURE 3. UV-Vis diffuse reflectance spectra of various SiO2-TiO2-Fe2O3 combinations. Inset: the plots of (αhv)1/2 vs. photon energy of different catalysts. Ratio percentage of Fe/Ti amount: a, 0%; b, 1%; c, 3%; d, 5%; e, 8% (source: Wang et al. (2016)).
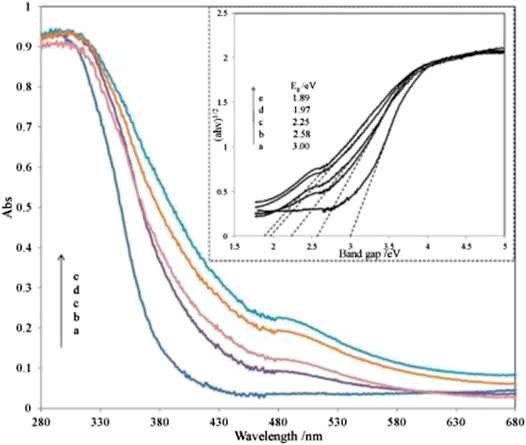
FIGURE 4. UV-Vis diffuse reflectance spectra of various SiO2-Fe2O3-TiO2 combinations. Inset: the plots of (αhv)1/2 vs. the photon energy of different catalysts. Ratio percentage of Fe/Ti amount: a, 0%; b, 1%; c, 3%; d, 5%; e, 8% (source: Wang et al. (2016)).
The codoped SiO2-Fe2O3(5%)-TiO2 by N and B exhibited high photocatalytic activity of methyl orange under weak room light irradiation (4 W fluorescent lamp with rare earth phosphor) due to synergistic effect of both N doping and Fe2O3 coupling which enhanced the visible-light absorbance and reduced the band-gap energy of TiO2-based catalyst and at the same time, and both N and B doping on TiO2 caused high separation efficiency for photoinduced electrons and holes (Wang et al., 2016).
Deposition of Plasmonic Metal Nanoparticles
Another option to maximize the application of TiO2-SiO2 photocatalyst in the visible-light region is by incorporating plasmonic metals such as Ag and Au into TiO2 in order to utilize their ability in absorbing strongly in visible region. Their ability in absorbing of solar photons is attributed to the Localized Surface Plasmon Resonance (LSPR) (Hao et al., 2016). LSPR is defined as collective motions of conduction electrons induced by light irradiation (Chen et al., 2012). Some of the previous attempts to deposit plasmonic metal onto the TiO2-SiO2 composite are listed in Table 4.
Plasmonic nanoparticles (such as Ag and Au) exhibit a property identified as surface plasmon resonance (SPR) especially in the visible region of the electromagnetic spectrum (Cho and Krishnan, 2013; Abdullah and Kuo, 2015). Many previous research studies deposited Ag/Au nanoparticles onto TiO2-SiO2 composite photocatalyst due to their ability to enhance photocatalytic activities by trapping the generated photoelectron and reducing the recombination rate of generated electrons and holes by acting as an electron trap (Chen et al., 2012; Abdullah & Kuo, 2015). The Ag/Au nanoparticles enhance the efficiency of TiO2-SiO2 photocatalyst by absorbing the solar photons and transferring the energetic electron, formed via SPR excitation, into the TiO2 (Hamal and Klabunde, 2010; Linic et al., 2011). This mechanism is demonstrated in Figure 5, 6.
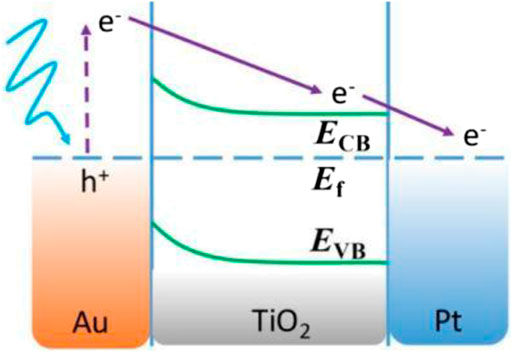
FIGURE 5. Schematic representations of excited electron generated in AuNS and transfer to the TiO2 CB, where ECB, Ef, and EVB represent the energies of the conduction band, Fermi level, and valence band, respectively (source: Shi et al. (2016)).
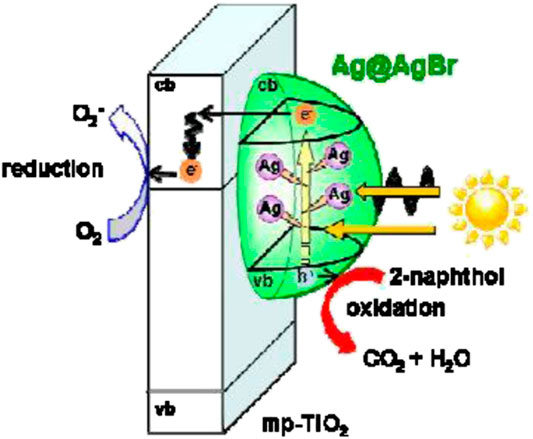
FIGURE 6. Reaction mechanism of the Ag@AgBr/mp-TiO2 photocatalyst under visible-light irradiation (source: Hayashido et al., 2016).
Evidence of higher efficiency achieved by plasmonic metal deposited TiO2-SiO2 was reported by Liu et al. (2013). Liu showed that under 4 h of visible irradiation, the TiO2-SiO2-Ag photocatalyst exhibited an almost complete degradation of rhodamine B (10ppm) as compared to 80% by TiO2-Ag and only 30% by neat TiO2. In comparison, Hayashido et al. (2016) documented that Ag@AgBr/mp-TiO2 is an efficient photocatalyst in the visible-light region (λ > 400 nm) due to the visible-light activity of Ag@AgBr.
Addition of Ag into TiO2 modifies the lattice parameters of TiO2 by generating oxygen vacancies which act as active sites for photocatalysis process, reduces recombination rates of photoexcited electrons and holes due to the formation of Schottky barrier between Ag and TiO2, reduces band-gap value, and generates defect site Ti3+ (Gupta et al., 2006; Chen et al., 2007; Hamal and Klabunde, 2010). Hamal and Klabunde (2010) documented that 5% AgCl-SiO2, 5% AgBr-SiO2, and 5% AgI-SiO2 exhibited band-gap absorption in visible region as shown in Figure 7 and were able to degrade rhodamine B dye (concentration of 2 × 10–5 M) in the liquid phase and acetaldehyde in the gas phase under visible region (λ > 420 nm). Among these three, the 5% AgI-SiO2 was found to be the best photocatalyst due to its low band gap and high surface area as well as high stability.
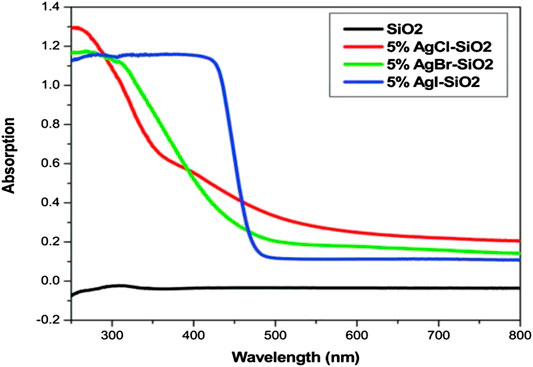
FIGURE 7. UV-Vis spectrophotometer absorption spectra of 5% silver halide supported on silica (Hamal and Klabunde (2010)).
When molar ratio of TiO2 in the TiO2-SiO2 photocatalyst structure is increased, the total surface area of TiO2-SiO2 decreases causing low photocatalytic activity of the composite catalyst (Ramamoorthy et al., 2016). In a study reported by Jeon et al. (2015), at high molar ratio of TiO2 (molar ratio of TiO2 precursor to SiO2 precursor of 0.05) in the TiO2-SiO2 photocatalyst, a dense thin film with mostly blocked pores was observed. This indicates that at higher concentration of the TiO2 precursor relative to the SiO2 precursor, the size of the pores becomes smaller and may be completely blocked. In contrast at high SiO2 precursor molar ratio relative to TiO2 precursor, the amount of vertically perforated pores increases and forms large micron-sized cracks due to the inability in enduring thermal shrinkage stress due to the high film thickness. Thickness of the film has been reported to decrease with the increase in molar ratio of SiO2 in TiO2/SiO2 film (Fateh et al., 2013).
When the amount of SiO2 used to modify the TiO2 is too small (<2%) or too large (>5%), it causes lower photocatalytic degradation of TiO2-SiO2 as compared to commercial P25 TiO2. In addition, from the XRD analysis, it was reported that doping small amount of SiO2 on TiO2 will not effectively prevent rutile phase transformation, which also produces lower photocatalytic activity while high mount of SiO2 doping will influence the optical absorption of TiO2 which is not favourable in photocatalytic reactions (Kang et al., 2009).
Lee et al. (2017a) employed the citrate reduction method to prepare Au and polyol method to prepare Ag nanoparticles, respectively. After that, modified Stober process was employed to coat Au and Ag surfaces with SiO2. The prepared composites were later deposited onto TiO2 (Degussa P25) by employing maleic acid as an anchoring agent. Lee et al. (2017b) reported that Ag@SiO2-doped TiO2 nanoparticles are significantly more effective in photocatalytic activity than Ag-doped TiO2. Ag25@SiO2/TiO2 exhibited the highest activity in decomposing aqueous salicylic acid and aniline under UV-visible light irradiation; which was 3.8 and 2.5 times, respectively, that of the bare TiO2. The authors attributed the high photocatalytic efficiency of Ag25@SiO2/TiO2 to strong LSPR effect of Ag.
Zhang et al. (2018) found that SiO2-Ag/TiO2 (SAT) showed enhanced visible-light activity and UV light activity compared to SiO2-TiO2/Ag (STA) for degrading tetracycline and traditional dyes. According to them, SiO2 serves as an efficient support for the Ag nanoparticle immobilization; meanwhile, TiO2 retains the hierarchical structure and prevents agglomeration of Ag nanoparticles during photocatalytic reaction. They attributed the excellent photocatalytic activity of SAT to improve the transport path of photogenerated electrons, diminished recombination probability of electron-hole pairs, and reduced threat of oxidation and corrosion. It should be noted that the SAT retained its photocatalytic efficiency even after five consecutive runs.
Conclusion
Plasmonic metal particle-incorporated TiO2-SiO2 composite plays an essential role as a solar photocatalyst which can transform solar energy into chemical energy for application in photocatalysis. Various methods and strategies were presented in this work that highlighted this incorporation, yielding different results. By taking advantage of three synergistic effects that can influence the photocatalytic activity of TiO2, ability to absorb solar photons by plasmonic metal nanoparticles (Ag or Au), and high adsorption activity by SiO2, it is possible to utilize the renewable solar energy for water and wastewater remediation effectively. With greater focus on this composite photocatalyst, the next few years will bring major advancement in utilizing the Ag/Au-incorporated TiO2-SiO2 in water and wastewater treatment plants at an industrial scale.
Author Contributions
CJ supervised the student, visualised, wrote, and edited the manuscript. YH wrote and edited the manuscript. BM made funding acquisition and edited the manuscript. MS made funding acquisition and edited the manuscript. LE conducted the research and investigation process and wrote the original draft.
Funding
This research was supported by the Research and Innovation Management Center of University Malaysia Sabah (grant no. SBK0354-2017), and it is gratefully acknowledged.
Conflict of Interest
The authors declare that the research was conducted in the absence of any commercial or financial relationships that could be construed as a potential conflict of interest.
References
Abdullah, H., Kuo, D. H., Lee, J. Y., and Wu, C. M. (2016). Recyclability of thin nylon film-supported p-CuBiS2/n-TiO2 heterojunction-based nanocomposites for visible light photocatalytic degradation of organic dye. Appl. Phys. A. 122 (8), 750. doi:10.1007/s00339-016-0276-4
Abdullah, H., and Kuo, D. H. (2015). Photocatalytic performance of Ag and CuBiS2 nanoparticle-coated SiO2@ TiO2 composite sphere under visible and ultraviolet light irradiation for azo dye degradation with the assistance of numerous nano p–n diodes. J. Phys. Chem. C. 119 (24), 13632–13641. doi:10.1021/acs.jpcc.5b01970
Bahadur, J., Sen, D., Mazumder, S., Sastry, P. U., Paul, B., Bhatt, H., et al. (2012). One-step fabrication of thermally stable TiO2/SiO2 nanocomposite microspheres by evaporation-induced self-assembly. Langmuir 28 (31), 11343–11353. doi:10.1021/la3022886
Balachandran, K., Venckatesh, R., Sivaraj, R., and Rajiv, P. (2014). TiO2 nanoparticles versus TiO2–SiO2 nanocomposites: a comparative study of photo catalysis on acid red 88. Spectrochim. Acta Mol. Biomol. Spectrosc. 128, 468–474. doi:10.1016/j.saa.2014.02.127
Bellardita, M., Addamo, M., Di Paola, A., Marcì, G., Palmisano, L., Cassar, L., et al. (2010). Photocatalytic activity of TiO2/SiO2 systems. J. Hazard Mater. 174 (1–3), 707–713. doi:10.1016/j.jhazmat.2009.09.108
Chan, P. Y., El-Din, M. G., and Bolton, J. R. (2012). A solar-driven UV/Chlorine advanced oxidation process. Water Res. 46 (17), 5672–5682. doi:10.1016/j.watres.2012.07.047
Chen, H. W., Ku, Y., and Kuo, Y. L. (2007). Photodegradation of o‐cresol with Ag deposited on TiO2 under visible and UV light irradiation. Chem. Eng. Technol. 30 (9), 1242–1247. doi:10.1002/ceat.200700196
Chen, J. J., Wu, J. C., Wu, P. C., and Tsai, D. P. (2012). Improved photocatalytic activity of shell-isolated plasmonic photocatalyst Au@ SiO2/TiO2 by promoted LSPR. J. Phys. Chem. C. 116 (50), 26535–26542. doi:10.1021/jp309901y
S. H. Cho, and S. Krishnan (Editiors) (2013). Cancer nanotechnology: principles and applications in radiation oncology. (Boca Raton, FL: CRC Press), 296.
Choi, K. S. (2007). Band gap tuning of zinc oxide films for solar energy conversionA CASPiE Module. West Lafayette, Ind: Purdue University, 38.
Fateh, R., Dillert, R., and Bahnemann, D. (2013). Preparation and characterization of transparent hydrophilic photocatalytic TiO2/SiO2 thin films on polycarbonate. Langmuir 29 (11), 3730–3739. doi:10.1021/la400191x
Fatimah, I., Said, A., and Hasanah, U. A. (2015). Preparation of TiO2-SiO2 using rice husk ash as silica source and the kinetics study as photocatalyst in methyl violet decolorization. Bull. Chem. React. Eng. Catal. 10 (1), 43–49. doi:10.9767/bcrec.10.1.7218.43-49
Fu, N., Ren, X.-C., and Wan, J.-X. (2019). Preparation of Ag-coated SiO2@TiO2 core-shell nanocomposites and their photocatalytic applications towards phenol and methylene blue degradation. J. Nanomater. 2019, 8. doi:10.1155/2019/8175803
Gardin, S., Signorini, R., Pistore, A., Giustina, G. D., Brusatin, G., Guglielmi, M., et al. (2010). Photocatalytic performance of hybrid SiO2−TiO2 films. J. Phys. Chem. C. 114 (17), 7646–7652. doi:10.1021/jp911495h
Gupta, A. K., Pal, A., and Sahoo, C. (2006). Photocatalytic degradation of a mixture of Crystal Violet (Basic Violet 3) and Methyl Red dye in aqueous suspensions using Ag+ doped TiO2. Dyes Pigments 69 (3), 224–232. doi:10.1016/j.dyepig.2005.04.001
Hamal, D. B., and Klabunde, K. J. (2010). “Heterogeneous photocatalysis over high-surface-area silica-supported silver halide photocatalysts for environmental remediation,”in Nanoscale materials in chemistry: environmental applications. (Washington: American Chemical Society), 191–205.
Hao, C. H., Guo, X. N., Pan, Y. T., Chen, S., Jiao, Z. F., Yang, H., et al. (2016). Visible-light-driven selective photocatalytic hydrogenation of cinnamaldehyde over Au/SiC catalysts. J. Am. Chem. Soc. 138 (30), 9361–9364. doi:10.1021/jacs.6b04175
Hayashido, Y., Naya, S. I., and Tada, H. (2016). Local electric field-enhanced plasmonic photocatalyst: formation of Ag cluster-incorporated AgBr nanoparticles on TiO2. J. Phys. Chem. C. 120 (35), 19663–19669. doi:10.1021/acs.jpcc.6b04894
Hu, C., Tang, Y., Jiang, Z., Hao, Z., Tang, H., and Wong, P. K. (2003). Characterization and photocatalytic activity of noble-metal-supported surface TiO2/SiO2. Appl. Catal. Gen. 253 (2), 389–396. doi:10.1016/S0926-860X(03)00545-3
Jeon, H., Lee, C. S., Patel, R., and Kim, J. H. (2015). Well-organized meso-macroporous TiO2/SiO2 film derived from amphiphilic rubbery comb copolymer. ACS Appl. Mater. Interfaces. 7 (14), 7767–7775. doi:10.1021/acsami.5b01010
Kang, C., Jing, L., Guo, T., Cui, H., Zhou, J., and Fu, H. (2009). Mesoporous SiO2-modified nanocrystalline TiO2 with high anatase thermal stability and large surface area as efficient photocatalyst. J. Phys. Chem. C. 113 (3), 1006–1013. doi:10.1021/jp807552u
Kibombo, H. S., Peng, R., Rasalingam, S., and Koodali, R. T. (2012). Versatility of heterogeneous photocatalysis: synthetic methodologies epitomizing the role of silica support in TiO2 based mixed oxides. Catal. Sci. Technol. 2 (9), 1737–1766. doi:10.1039/C2CY20247F
Lee, J. E., Bera, S., Choi, Y. S., and Lee, W. I. (2017a). Size-dependent plasmonic effects of M and M@ SiO2 (M= Au or Ag) deposited on TiO2 in photocatalytic oxidation reactions. Appl. Catal. B Environ. 214, 15–22. doi:10.1016/j.apcatb.2017.05.025
Lee, R., Kumaresan, Y., Yoon, S. Y., Um, S. H., Kwon, I. K., and Jung, G. Y. (2017b). Design of gold nanoparticles-decorated SiO2@TiO2 core/shell nanostructures for visible light-activated photocatalysis. RSC Adv. 7 (13), 7469–7475. doi:10.1039/C6RA27591E
Liga, M. V., Maguire-Boyle, S. J., Jafry, H. R., Barron, A. R., and Li, Q. (2013). Silica decorated TiO2 for virus inactivation in drinking water–simple synthesis method and mechanisms of enhanced inactivation kinetics. Environ. Sci. Technol. 47 (12), 6463–6470. doi:10.1021/es400196p
Linic, S., Christopher, P., and Ingram, D. B. (2011). Plasmonic-metal nanostructures for efficient conversion of solar to chemical energy. Nat. Mater. 10 (12), 911–921. doi:10.1038/nmat3151
Liu, C., Yang, D., Jiao, Y., Tian, Y., Wang, Y., and Jiang, Z. (2013). Biomimetic synthesis of TiO2–SiO2–Ag nanocomposites with enhanced visible-light photocatalytic activity. ACS Appl. Mater. Interfaces 5 (9), 3824–3832. doi:10.1021/am4004733
Matos, J., Llano, B., Montaña, R., Poon, P. S., and Hidalgo, M. C. (2018). Design of Ag/and Pt/TiO2-SiO2 nanomaterials for the photocatalytic degradation of phenol under solar irradiation. Environ. Sci. Pollut. Control Ser. 25 (19), 18894–18913. doi:10.1007/s11356-018-2102-3
Mungondori, H. H., Tichagwa, L., and Green, E. (2015). Synthesis and glass immobilization of carbon and nitrogen doped TiO2-SiO2 and its effect on E. coli ATCC 25922 bacteria. Br. J. Appl. Sci. Technol. 5 (5), 447. doi:10.9734/bjast/2015/11049
Panayotov, D., and Yates, J. T. (2003). Bifunctional hydrogen bonding of 2-chloroethyl ethyl sulfide on TiO2−SiO2 powders. J. Phys. Chem. B. 107 (38), 10560–10564. doi:10.1021/jp0304273
Ramamoorthy, V., Kannan, K., Joseph, J., Infant, A., Kanagaraj, T., and Thiripuranthagan, S. (2016). Photocatalytic degradation of acid orange dye using silver impregnated TiO2/SiO2 composite catalysts. J. Nanosci. Nanotechnol. 16 (9), 9980–9986. doi:10.1166/jnn.2016.12071
Ren, Y., Chen, M., Zhang, Y., and Wu, L. (2010). Fabrication of rattle-type TiO2/SiO2 core/shell particles with both high photoactivity and UV-shielding property. Langmuir 26 (13), 11391–11396. doi:10.1021/la1008413
Shi, X., Lou, Z., Zhang, P., Fujitsuka, M., and Majima, T. (2016). 3D-Array of Au–TiO2 yolk–shell as plasmonic photocatalyst boosting multi-scattering with enhanced hydrogen evolution. ACS Appl. Mater. Interfaces 8 (46), 31738–31745. doi:10.1021/acsami.6b12940
Singh, R., Bapat, R., Qin, L., Feng, H., and Polshettiwar, V. (2016). Atomic layer deposited (ALD) TiO2 on fibrous nano-silica (KCC-1) for photocatalysis: nanoparticle formation and size quantization effect. ACS Catal. 6 (5), 2770–2784. doi:10.1021/acscatal.6b00418
Wang, T., Zhu, Y. C., Xu, Z. Y., Wu, L. G., Wei, Y. Y., Chen, T. N., et al. (2016). Fabrication of weak-room-light-driven TiO2-based catalysts through adsorbed-layer nanoreactor synthesis: enhancing catalytic performance by regulating catalyst structure. J. Phys. Chem. C. 120 (22), 12293–12304. doi:10.1021/acs.jpcc.6b03721
Wei, Q., Li, Y., Zhang, T., Tao, X., Zhou, Y., and Chung, K., et al. (2014). TiO2–SiO2-composite-supported catalysts for residue fluid catalytic cracking diesel hydrotreating. Energy Fuels 28 (12), 7343–7351. doi:10.1021/ef500799t
Xu, Z., Huang, C., Wang, L., Pan, X., Qin, L., Guo, X., et al. (2015). Sulfate functionalized Fe2O3 nanoparticles on TiO2 nanotube as efficient visible light-active photo-Fenton catalyst. Ind. Eng. Chem. Res. 54 (16), 4593–4602. doi:10.1021/acs.iecr.5b00335
Zangeneh, H., Zinatizadeh, A. A. L., Habibi, M., Akia, M., and Isa, M. H. (2015). Photocatalytic oxidation of organic dyes and pollutants in wastewater using different modified titanium dioxides: a comparative review. J. Ind. Eng. Chem. 26, 1–36. doi:10.1016/j.jiec.2014.10.043
Keywords: TiO2-SiO2, plasmonic metal nanoparticles, visible region, photocatalysis, dye
Citation: Joseph CG, Taufiq-Yap YH, Musta B, Sarjadi MS and Elilarasi L (2021) Application of Plasmonic Metal Nanoparticles in TiO2-SiO2 Composite as an Efficient Solar-Activated Photocatalyst: A Review Paper. Front. Chem. 8:568063. doi: 10.3389/fchem.2020.568063
Received: 31 May 2020; Accepted: 18 December 2020;
Published: 29 January 2021.
Edited by:
Sivakumar Ponnusamy, Arignar Anna Government Arts College, IndiaReviewed by:
Senthil Subramaniyam, Erode Sengunthar Engineering College, IndiaShanmugam Palanisamy, Anna University, India
Geetha Arumugam, Anna University, India
Copyright © 2021 Joseph, Taufiq-Yap, Musta, Sarjadi and Elilarasi. This is an open-access article distributed under the terms of the Creative Commons Attribution License (CC BY). The use, distribution or reproduction in other forums is permitted, provided the original author(s) and the copyright owner(s) are credited and that the original publication in this journal is cited, in accordance with accepted academic practice. No use, distribution or reproduction is permitted which does not comply with these terms.
*Correspondence: Collin G. Joseph, Y29sbGluQHVtcy5lZHUubXk=