- 1Central Metallurgical Research and Development Institute, Cairo, Egypt
- 2Department of Environmental Engineering and Earth Sciences, Clemson University, Clemson, SC, United States
- 3Department of Engineering and Science Education, Clemson University, Clemson, SC, United States
- 4Interdisciplinary Group for Biotechnological Innovation and Ecosocial Change-BioNovo, Universidad del Valle, Cali, Colombia
For the past few decades, a plethora of nanoparticles have been produced through various methods and utilized to advance technologies for environmental applications, including water treatment, detection of persistent pollutants, and soil/water remediation, amongst many others. The field of materials science and engineering is increasingly interested in increasing the sustainability of the processes involved in the production of nanoparticles, which motivates the exploration of alternative inputs for nanoparticle production as well as the implementation of green synthesis techniques. Herein, we start by overviewing the general aspects of nanoparticle synthesis from industrial, electric/electronic, and plastic waste. We expand on critical aspects of waste identification as a viable input for the treatment and recovery of metal- and carbon-based nanoparticles. We follow-up by discussing different governing mechanisms involved in the production of nanoparticles, and point to potential inferences throughout the synthesis processes. Next, we provide some examples of waste-derived nanoparticles utilized in a proof-of-concept demonstration of technologies for applications in water quality and safety. We conclude by discussing current challenges from the toxicological and life-cycle perspectives that must be taken into consideration before scale-up manufacturing and implementation of waste-derived nanoparticles.
Introduction
According to the Environment Program of the United Nations, nearly 11.2 billion tons of solid waste is generated every year, which is a significant source of environmental degradation and negative health impacts, particularly in low-income countries, where more than 90% of waste is openly dumped or burned (UNEP, 2020). In Southeast Asian countries with insufficient waste management capacity, such as Vietnam, Malaysia, the Philippines, and Thailand, waste-related issues are further exacerbated by the massive amounts of plastic, electric, and electronic waste imported from industrialized countries (Kaza et al., 2018; Dell, 2019; Sukanan, 2020). This situation has motivated the development, implementation, and strengthening of different policy strategies including (i) international environmental agreements such as the Basel Convention on the control of transboundary movements of hazardous wastes and their disposal (Basel Action Network, 2019), (ii) source reduction, or complete bans on single-use plastics (UNEP, 2018), and (iii) shifts in political agendas toward the adoption of circular economy models for meeting environmental and resource-oriented goals in several countries (Bourguignon, 2014; Berg et al., 2018). However, there are significant technical, economic, environmental, and social challenges for realizing closed-loop management of engineered materials on a large scale. For example, many plastic and metal recycling processes involve the use of hazardous substances for extraction and purification, which results in new health risks for humans and the environment (Kral et al., 2019). Besides, the practical viability of strategies for recycling and repurposing materials is directly linked to the economic value of the end-products. Therefore, using “clean manufacturing” processes to yield “value-added” commodities from waste materials emerges as a desirable synergy for achieving both circularity and sustainability goals (Ferronato and Torretta, 2019).
Over the past few years, a plethora of engineered nanomaterials have demonstrated great promise for advanced applications ranging from energy transformation and storage (Hussein, 2015; Verma et al., 2016; Sonawane et al., 2017; Yang et al., 2019), pollution monitoring (Baruah and Dutta, 2009), intelligent packaging (Pereira de Abreu et al., 2012), precision agriculture and controlled delivery of food ingredients (Bindraban et al., 2015; Peters et al., 2016), membrane technology (Goh et al., 2015); water treatment (Westerhoff et al., 2013); drug delivery and diagnostics (Turcheniuk and Mochalin, 2017), bone and tissue engineering (Shadjou and Hasanzadeh, 2016), amongst others. In an effort to reduce negative environmental impacts and health risks associated with the production, use, and disposal of novel nanomaterials, the fields of materials engineering and nanotechnology are increasingly concerned with sustainability approaches, frameworks, and metrics (Dhingra et al., 2010; Mata et al., 2015; Falinski et al., 2018). For example, a Boolean search of the keywords “green synthesis” AND “nanomaterials” on the academic engine Web of Science (Clarivate™) returns a record count of 569 articles published on 2019 alone, which is 3-fold higher number than the year 2015, and 12-fold higher than the year 2009. This growing trend is expected to continue over the next few years, consistent with global trends on sustainable development.
Herein, we provide an overview of the current status, challenges, and future directions for the utilization of industrial wastes (e.g., large batteries, rubber tires, wastewater), e-waste (e.g., copper cables, printed circuit boards, electronic equipment), and plastic wastes (e.g., polyethylene, polypropylene, polyvinyl alcohol) as suitable inputs for production of nanoparticles (NPs) as added-value products. In addition, we review some proof-of-concept examples for the incorporation of waste-derived NPs in advanced technologies for pollution monitoring, treatment, and remediation of water (Figure 1). We conclude with some remarks from the sustainability viewpoint, that emphasize the critical aspects necessary for scale-up manufacturing and deployment of waste-derived nanomaterials.
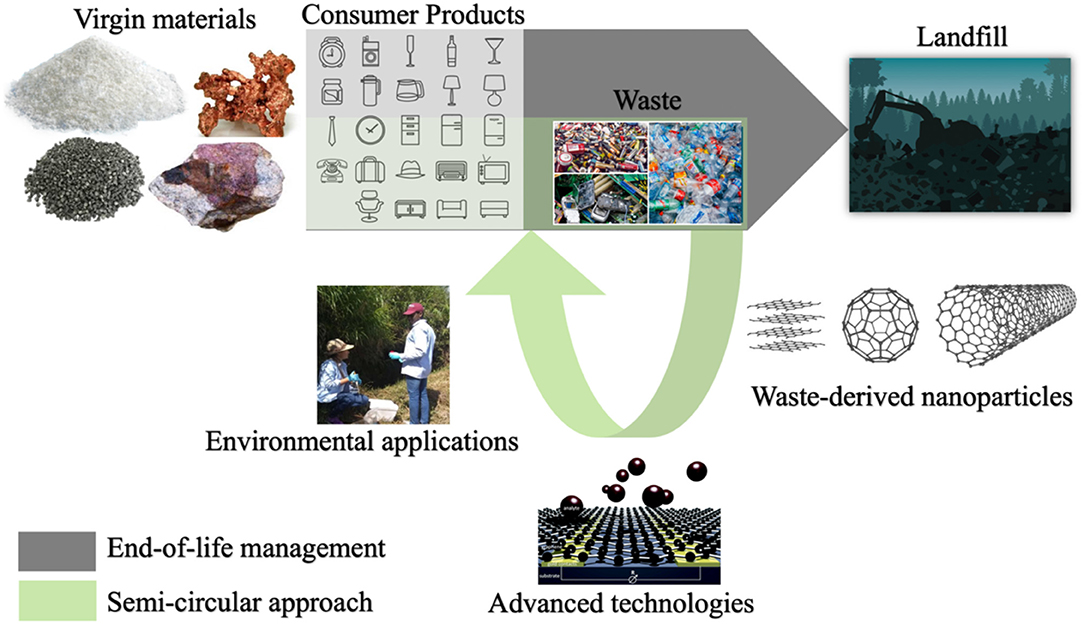
Figure 1. Schematic representation of the concept discussed in the review article. industrial, electric, electronic and plastic wastes are suitable inputs for sustainable production of engineered nanoparticles, which can be further used in the development of advanced technologies for environmental applications. Thus, disrupting the linear end-of-life approach to waste management and increasing sustainability by closing the loop with the fabrication of value-added materials and technologies from waste. This schematic was created using public domain images obtained from a open source database.
Waste as Starting Materials for the Production of Nanoparticles
Depending on the source of emission, highly problematic waste materials produced in massive amounts can be classified into wastes generated by industries, and wastes generated by consumers. Both industrial and consumer wastes can be utilized as inputs for processes that yield value-added products. From the industrial sector, large batteries, rubber tires, wastewater, and biosolids are prominent sources of carbon, lead, zinc, copper, and palladium. End-of-life consumer products result in overwhelming amounts of e-waste and plastic waste. E-waste can be a significant source of recoverable precious and semi-precious metals. Depending on the type of polymer and the degree of purity, plastic waste can be either recycled and reused as packaging material or, it can be used as raw material for other applications, including the production of construction materials, paper, fiber composites, new polymers, and carbon nanoparticles. In this section, we examine the recent research on the mechanisms and processes used for the recovery of valuable components from the different waste streams.
Industrial Waste
Up to this point in time, several industrial wastes such as batteries, tires, wastewater, and sludges have been studied as potential low-cost and ubiquitous starting materials for nanoparticles synthesis (Samaddar et al., 2018).
Lead Batteries
Over 50 million units of waste lead batteries are produced annually by China alone; considering the toxicological effects of lead exposure, along with its bioaccumulation capacity, recycling these batteries is a responsible approach while avoiding ecological and public health problems (Zhou et al., 2017). Lead pastes are made of metal oxide, dioxide, and sulfate forms that are difficult to recover and reuse because of their insolubility. However, pyrometallurgical processes can be employed to retrieve lead from spent lead-acid battery paste. For example, Zhou et al. (2017) designed a synthesis technique to make Pb nanopowder from simulated spent lead paste by the pyrolytic route. The authors produced submicron particles of lead oxide by a thermal process coadjuvated by poly (N-vinyl-2- pyrrolidone), which helps decrease the particle size (Zhou et al., 2017). While the technique overcomes difficulties associated with the insolubility of lead paste in aqueous media, pyrometallurgical processes may have unfavorable consequences due to the high energy requirements as well as the undesirable emissions of air pollutants such as sulfur dioxide and lead dust.
Zinc-Manganese Batteries
Similarly, a large percent of transportable batteries in industrialized countries are made of zinc-manganese (Zn-Mn) composites. China alone produces more than 15 billion units every year (Xiang et al., 2015). Exposure to toxic concentrations of Zn can result in impaired calcium uptake and metabolic deficiencies in humans and other organisms (Valko et al., 2005). Thus, recovering Zn from recycled Zn-Mn batteries is a convenient approach for enabling reuse of the metal in other applications while limiting its uncontrolled dissemination in the environment. Synthesis of Zn nanomaterials such as NPs, nanofibers, and flaky NPs using recycled Zn-Mn batteries as starting material, has been actively investigated in recent years. Xiang et al. (2015) demonstrated a facile and highly efficient method for separating zinc from spent batteries. Samples of recycled zinc-cathodes were put in a corundum crucible and subjected to a vacuum pressure of 1 Pa, heating temperature of 800°C, and condensing temperature 200°C. The simple technique can attain zinc separation efficiency up to 99.68%. By following the separation process with a nitrogen gas flow into the heating chamber, structured nanoparticles can be obtained with different morphologies including hexagonal prisms, fibers, and sheets (Xiang et al., 2015).
Rubber Tires
Worldwide, roughly 1,000 million scrap tires are generated every year (GoldsteinResearch, 2020). A significant portion is landfilled or openly burned, contributing to air pollution and environmental degradation (Moghaddasi et al., 2013). For that reason, the disposal of scrap rubber in landfills is banned in most developed countries (Turgut and Yesilata, 2008). Thus, there is interest in finding sustainable solutions for managing rubber tire waste. Generally, tires are composed of 45–47% natural rubber, 21–22% carbon black, 12–25% metals, 5–10% textile, 6–7% additives and 1–2% zinc, ~1% sulfur (Evans and Evans, 2006; Samaddar et al., 2018). The synthesis of Zn NPs from used tires constitutes a suitable alternative with value-added potential. Moghaddasi et al. (2013) obtained NPs from recycled tires by applying ball milling for five consecutive hours. The method yielded rubber ash particles smaller than 500 nm; when aided with silicon waste, the particle size was further reduced to <50 nm. The effectiveness of rubber ash and ground tire rubber particles as a zinc source for plant nutrition was comparable to a commercial ZnSO4 fertilizer (Moghaddasi et al., 2013).
As waste tires are composed, principally, of carbon (representing about 81.2 wt%) they are considered a promising source of carbon for various potential applications. Maroufi et al. (2017) synthesized high-value carbon nanoparticles (CNPs) from waste tire rubber (WTR), using a high-temperature approach. The transformation of WTRs was carried out at 1,550°C over various reaction times (5 s to 20 min). The total energy utilization for the process was acceptable as the decomposition of WTR and the formation of CNPs occurs quickly, and completely. Within 5 min, all the impurities, such as sulfur, zinc, and oxygen, have been removed (Maroufi et al., 2017). Another facile and low-cost method for the preparation of carbon black nanoparticles from waste tires was reported by Gómez-Hernández et al. (2019). Briefly, thermal transformation under the high temperature (1,000°C) and self-pressurized conditions produced carbon nanoparticles (~22 nm) with high yield (about 81%) under the optimized conditions. The chain-like agglomerated nanoparticles showed good thermal stability and conductivity and the chemical analysis indicated that it was partly oxidized (C, 84.9%; O, 4.9%; S, 10.21%) (Gómez-Hernández et al., 2019). Many studies have been reported advising to choose an unconventional hydrocarbon waste as low-cost starting materials such as tires and plastics for the synthesis of CNTs (Sathiskumar and Karthikeyan, 2019). Multiwalled carbon nanotubes were synthesized from low boiling point hydrocarbon tire pyrolysis oil derived from waste tire material mixed with ferrocene as the catalyst on a quartz substrate with a flow rate of 20 ml/min at 950°C (Parasuram et al., 2018).
Wastewater and Bio-sludge
Wastewater effluents and industrial sludges have been identified as rich sources of valuable metals and metal oxides; therefore, these waste effluents have been extensively studied for the recovery of materials such as PbO, Mo, Ni, Co, Cu, Ag, SiO2, rare earth elements, and Pt. While the concentration of trace elements is highly variable among waste effluents, there are associations with the specific activities performed in the emitting source, as well as the mineralogical diversity found within a single effluent source. For example, the U.S. Geological Survey has identified waste effluents from Zn ore mining as major sources of Ga and In. Through wastewater and biosolid mining, Ga and In could be recovered and used for the fabrication of integrated circuits, light-emitting diodes, photodetectors, semiconductors, and solar cells (Smith et al., 2015). An elemental analysis of waste stream samples (e.g., mining influenced waters) from different historical metal mining sites found concentrations of Ag (Mdn. ~10 ppm), Au (Mdn. ~0.3 ppm), Pd (Mdn. ~30 ppm), Pt (Mdn. ~1 ppm), Re (Mdn. ~ 0.01 ppm), Os (Mdn. ~0.02 ppm), Ir (Mdn. ~0.001 ppm), La (Mdn. ~20 ppm), Ce (Mdn. ~40 ppm), Nd (Mdn. ~20 ppm), Sm (Mdn. ~4 ppm), Gd (Mdn. ~4 ppm), Yb (Mdn. ~2 ppm), Th (Mdn. ~4 ppm), Zn (Mdn. ~1,000 ppm), Cu (Mdn. ~150 ppm), Ga (Mdn. ~15 ppm), In (Mdn. ~ 1 ppm), Sb (Mdn. ~10 ppm), and Te (Mdn. ~1.5 ppm). Although the recovery of some of these metals may not be economically attractive by itself, the removal and recovery of trace elements from waste streams may still offset treatment and disposal costs and reduce environmental and public health liability (Smith et al., 2015).
Urbina et al. (2019) demonstrated an innovative approach for metal recovery from aqueous waste such as those found in bioremediation or biomining processes. The method uses metal-binding peptides to functionalize fungal mycelia. In silico models have been developed for predicting binding affinity between metals and natural ligand-binding motifs. These models are useful because they enable the prediction of binding parameters based on open access protein databases (e.g., The Protein Data Bank https://www.rcsb.org) to describe the geometric features a cognate metal in a metalloprotein. As a proof-of-concept, the authors achieved binding affinity and specificity for Cu that show a high correlation between the natural motifs and those derived in silico (Urbina et al., 2019). Another study identified different morphologies of silica nano-fillers (100–500 nm) present in polymer waste (Tran et al., 2017). Various metals have also been recovered from pickling wastes, spent catalysts, furnace slag, fly ash, e-waste, and metalized plastic waste (Khaloo et al., 2013; Bennett et al., 2016; Chaukura et al., 2016; Chen et al., 2016; El-Nasr et al., 2020; Elsayed et al., 2020). Aside from being a good source for the recovery of metals, various industrial effluents and bio-wastes have been used for the synthesis of carbon-based nanomaterials (Deng et al., 2016; Zhang et al., 2016). Deng et al. provided a comprehensive review highlighting the current status and prospects of green synthesis of carbon nanocomposites from bio-sludges and industrial wastes through microbiologically driven pathways (Deng et al., 2016).
Electric and Electronic Wastes
The overwhelming generation of end-of-life electric and electronic products and equipment (e-waste or WEEE) in the United States and Europe is causing severe environmental and public health issues in the e-waste receiving nations, which are primarily located in West Africa and Asia (Baldé et al., 2017; Tansel, 2017). For the past decades, there has been a steady increase in the development of efficient recycling techniques for e-waste led by the US and China, especially with regards to the recovery of metallic components (Bhat et al., 2012; Wibowo et al., 2014; Barletta et al., 2015; Park et al., 2017; Zeng et al., 2017). However, e-waste recycling is increasingly challenging because electronic devices are made of complex mixtures of various metals, plastics, glass, and even newly engineered nanomaterials (Abdelbasir et al., 2018b,c). Moreover, electronic components are continually being miniaturized, which diminishes the efficiency of conventional mechanical separation processes available in developing countries (Baldé et al., 2017). Despite the practical difficulties, the high economic value of precious metals, such as gold, silver, and palladium, continues to drive e-waste mining.
As shown in Table 1, Printed circuit boards and mobile phones contain the highest amounts of precious metals and a considerable portion of base metals. Printed circuit boards (PCBs) are an integral component of all electronics. On average, PCBs represent 3% of the total mass of waste electric and electronic equipment, but in the case of small appliances, it can account for almost 8% of the weight (Dalrymple et al., 2007; Luda, 2011; Abdelbasir et al., 2018c). In 2014 the value of metals in e-waste was roughly estimated at 52 billion U.S. dollars (Xu et al., 2016). The estimated overall composition of PCBs is 40% metals, 30% organics, and 30% persistent noxious materials (Abdelbasir et al., 2018b). Detailed elemental analysis of polymers present in PCB has revealed the following composition: 5.52 wt% C, 2.18 wt% H, 0.73 wt% N, and 7.86 wt% Br (Blazsó et al., 2002). Copper represents the highest amount of metals in PCBs, and it has the second-highest pecuniary value. The organics are generally made of a variety of polymers such as polyvinyl chloride (PVC), polycarbonate (PC), acrylonitrile butadiene styrene (ABS), polytetrafluoroethylene (PTFE), polyethylene (PE), and polypropylene (PP) (Stevens and Goosey, 2009). The toxicity of the materials found in PCBs is a recurrent concern in the characterization and treatment of waste PCBs. The lead-tin soldering material and the brominated flame retardants (BFRs) contained in PCBs are the most harmful constituents. Additionally, copper catalyzes the formation of dioxins when the flame retardants are incinerated.
Conventional industrial waste management processes involve transport of e-waste over long distances, to only recover a small fraction of materials; primarily precious metals such as Au and Ag, and semi-precious metals, like Cu, Ni, Al, Zn, Pb, and Sn, leaving significant amounts of residual waste and toxic components (Mankhand et al., 2012). Generally, industrial recycling approaches start with energy-intensive mechanical operations such as bulk crushing, grinding, and separation based on density or magnetic properties (Long et al., 2010); chemical treatments are later applied for extraction and purification of copper and other precious metals (Quan et al., 2010; Mankhand et al., 2012).
Overall, the metal content in e-waste is much higher than in land-mined ores (Li et al., 2007; Duan et al., 2009; Zhao et al., 2012). For example, e-waste has a weight composition of 10–20% Cu, whereas the maximum concentration of Cu in virgin ore is 3%. Thus, recovering metallic elements from e-waste, rather than mining for ores in natural deposits, would significantly cut the environmental impacts associated with both the mining of metals and the accumulation of e-waste. Enormous energy savings have been estimated from the recovery of metals from e-waste in contrast with the conventional extraction from mined ores. Specific examples of comparative cost-benefits are 95% for Al, 85% for Cu, 75% for Zn, and 90% for Ni (Cui and Forssberg, 2003; Shokri et al., 2017). Conventional approaches for recovering metals from e-waste begin with the conversion of the metallic components found in e-waste to oxide or sulfite forms, followed by the iterative raising of the pure form of the elements. Recently, efficient processes such as pyro- and hydro- metallurgy of e-waste captured significant attention. In pyrometallurgy, crushed e-waste is placed into a molten bath where the plastic degrades while releasing energy, and the refractories are ejected as slag. This slag is subsequently processed for the extraction of base or precious metals (Cayumil et al., 2014). Alternatively, hydrometallurgy processing is more predictable and controllable, but also very costly since it requires recovery and treatment of spent liquids and preparation of fresh solutions. Furthermore, the presence of chlorides in hydrometallurgically-treated e-waste is likely detrimental to the environment (Mankhand et al., 2012).
While the high yields of precious and semiprecious metals from WEEE provide the economic incentive for recycling it, there is still a need for more efficient and sustainable approaches to WEEE management that use a more substantial fraction of the waste materials (not just the metal parts) and results in the generation of value-added products (Abdelbasir et al., 2018c).
Plastic Wastes
The broad utilization of non-biodegradable plastics has arguably brought about the most significant and problematic class of waste materials in the world. Plastic polymers have been intensely used for about a century to fabricate an immense number of products. In fact, the production of synthetic plastics has increased vastly during the last decades (Gu and Ozbakkaloglu, 2016). Traditional plastics were designed to be durable and withstand variations in external environmental conditions, which is precisely the reason why they persist and accumulate in the environment (Pol and Thiyagarajan, 2010). On a global scale, plastics are now the major component in solid waste (Ritchie and Roser, 2020). The generation of plastic wastes (PW) accounts for over 150 million metric tons per year (Aboulkas et al., 2010).
Plastic debris and microplastics have been found in alarming concentrations in the ocean. These particles produce negative impacts not only on aquatic life and birds but also on humans via contamination of the trophic chain (Bouwmeester et al., 2015). Aside from the problems associated with their slow degradation, PWs are harmful because they contain pigments with several highly noxious trace elements that can migrate out of the polymeric matrix and into the environment (Gondal and Siddiqui, 2007). As a result, environmental pollution from synthetic PWs is now considered one of the most devastating and likely irreversible effects of contemporary anthropogenic activities (Zheng et al., 2005).
In many countries, separation at source (i.e., segregation of materials at the point of disposal) and recycling are main collective efforts initiated at the household level, which are aimed at reducing the need for producing new plastics from virgin materials and fossil fuels. Main synthetic polymers in household items include polystyrene (PS), polypropylene (PP), polyethylene terephthalate (PET), high-density polyethylene (HDPE), low-density polyethylene (LDPE), linear low-density polyethylene (LLDPE), polyvinyl chloride (PVC) and nylon. Table 2 depicts the properties of common plastic polymers. Considering the vast amount of solid waste generated worldwide, stakeholders are looking for avenues to use PWs as starting material for energy recovery or valued-added products manufacturing (Ackerman, 2000). From the thermodynamic perspective, energy recovery is less favorable as the energy content of plastic (42.6 MJ/l) is at least an order of magnitude lower than conventional fuels such as heating oil (443.5 MJ/kg) (Kumar et al., 2011; Banu et al., 2020). Additionally, using PW as an energy source can be seriously detrimental for public health since harmful dioxins are released during PW burning. Finally, the concept of using PWs as an alternative feedstock to generate syn-gas or oil for the later conversion into liquid fuels such as diesel and gasoline may incentivize the unnecessary perpetuation of dependence on carbon fuels, which ultimately leads to the release of greenhouse gases into the atmosphere, and all its encompassing effects on the planet.
On the other hand, turning PW into useful products is particularly challenging because of the chemical heterogenicity in the different waste streams. For instance, many packaging materials are made of compact composites of several thin layers of different polymers and often are lined with aluminum. These composite plastics have to be landfilled because they are difficult to separate by conventional mechanical and/or chemical recovery processes (Al-Salem et al., 2009; Astrup et al., 2009; Singh et al., 2017). Recent approaches for valorizing mixed PWs include mixing them with materials such as wood, cement, and ash to turn them into construction materials (Sardot et al., 2012; Sofi et al., 2019), printable paper-like composites (Fan et al., 2020), fiber composites (Keskisaari and Kärki, 2017), new polymers (Rahimi and Garciá, 2017), and polar waxes (Marek et al., 2015). Nanomaterials have also been prepared from single-polymer PW. For example, ultrafine nano-channeled carbon tubes and multi-walled carbon nanotubes (MWCNTs) have been synthesized from polyethylene terephthalate waste using an arc discharge method (Berkmans et al., 2014). Table 3 summarizes the advantages and challenges of some of the potential value-added applications of PWs.
In summary, the depicted mechanisms show promising potential for reducing the amount of landfilled materials and noxious elements dispersed in the environment. It is worth noting that the majority of the reviewed processes are still in the early stages of research and development, thus the economics of scale have not yet been fully determined. Nonetheless, the proposed processes serve as a proof-of-concept demonstration of ways to divert from the business-as-usual approach to waste management. Perhaps, offsetting treatment and disposal costs, and reducing environmental and public health liabilities may be sufficient incentives to promote these alternative approaches.
Synthesis of Nanoparticles from Recycled Materials
Metal and Metal Oxide Nanoparticles
Various procedures have been used for the synthesis of several types of nanomaterials from wastes after suitable pretreatment either physically or chemically or combinations of both. The most common physical pretreatment methods are grinding and milling. Chemical pretreatment is applied to separate any contaminants present in the waste sample by heating or treating with reagents such as strong acids (e.g., H2SO4, HNO3, HCl) (Samaddar et al., 2018).
The most popular synthesis method for metallic NPs is reduction with sodium borohydride. Generally, the sodium borohydride solution is freshly prepared and rapidly added to the solution of waste materials. NPs produced via reaction are washed repetitively with ultrapure water and absolute alcohol for the removal of excess sodium borohydride. This method was implemented by Fang et al. to prepare Fe NPs from steel pickling waste materials released from a steel plant (Fang et al., 2011).
Another important and widely used method is the solvent thermal method which involves chemical reactions in solvents contained in closed autoclave reactor and heated to a critical temperature (Dubin et al., 2010). When water is used as a solvent the process is known as “hydrothermal.” As an example, hematite NPs (α-Fe2O3) were synthesized via a solvent thermal method using ethanol as a solvent and heating the solution of the starting materials mixture (FeCl3, and NaOH) at 150°C for 2 h to gain NPs (Tang et al., 2011). Another technique that uses voltage to promote chemical reactions in aqueous solutions for the synthesis of nanoparticles is electrodeposition. This method has been applied to produce nanowires, nanoporous materials, and nanocylinders. In addition to these mentioned techniques, green synthesis methods based on the use of environmentally friendly and biocompatible materials such as plants and microorganisms have recently arisen (Jeevanandam et al., 2016).
Metal nanoparticles (e.g., Pb, Hg, Cu, Fe, Au, Ag, Pd, Pt, and Rh) and polymers can be recovered from electronic wastes such as computer circuit boards, cellular phones, laptops, automobiles, and supercapacitors (Xiu and Zhang, 2012; Singh and Lee, 2016; Vermisoglou et al., 2016). To date, most efforts have focused on the recovery of metal nanoparticles from spent batteries. However, other forms of e-waste are also good sources of a variety of valuable materials. For example, Cu nanoparticles were prepared from acidic CuCl2 waste etchants generated in the manufacturing process for printed circuit boards by microemulsion processes (Mdlovu et al., 2018). Likewise, aqueous solutions of CuSO4 from PCBs were chemically reduced to create organically stabilized Cu nanoparticles (Tatariants et al., 2018). Waste printed circuit boards (WPCBs) have also been recycled to obtain metals such as Cu, Pb, Fe, Au, and Hg (Calgaro et al., 2015; Chen et al., 2015a,b; Chu et al., 2015; Fogarasi et al., 2015; Hadi et al., 2015; Cui and Anderson, 2016). The metals recovered from WPCBs can be used to create nanoparticles. Cu from WPCBs has acted as a starting material in the synthesis of Cu nanoparticles (Yousef et al., 2018; El-Nasr et al., 2020). Figure 2 portrays the morphological features of Cu nanoparticles obtained from WPCBs using the approach by El-Nasr et al. (2020).
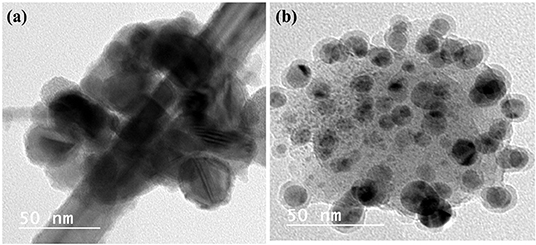
Figure 2. High resolution TEM images of CuNPs obtained from WPCBs. These NPs were prepared from (a) ammoniacal ammonium chloride and (b) ammoniacal ammonium carbonate leachant by ascorbic acid in presence of cetyltrimethylammonium bromide (CTAB) as modifier. These images were reproduced from Seif El-Nasr et al. (2020) under the reproduction license number 4854300796766 provided by Elsevier and Copyright Clearance Center.
WPCBs can also be used to create nanoparticles directly. Cu2O/TiO2 photocatalysts were recovered using electrokinetic processes (Xiu and Zhang, 2009). Cu2O nanoparticles of different sizes were also created from WPCBs using supercritical water oxidation methods (SCWO) and electrokinetic processes (Xiu and Zhang, 2012). Copper–tin (Cu–Sn) nanoparticles were fabricated from the WPCBs of spent computers using selective thermal transformation while also separating toxic lead (Pb) and antimony (Sb) (Shokri et al., 2017). Lead (Pb) nanoparticles were synthesized from solders of WPCBs via evaporation under vacuum with forced flow inert gas condensation (Zhan et al., 2016). Various other forms of e-waste can be used to create nanoparticles such as purified carbon nanotubes, Cu2O nanoparticles, and Cu2O/TiO2 catalysts. For example, Ag was completely recovered from incinerated organic solar cells (Søndergaard et al., 2016).
Wastes other than e-wastes can also be utilized. Ag, Cu, and bimetallic Ag/Cu nanoparticles were synthesized from a leachate solution (Figure 3). This solution was derived from a solution of leached metalized acrylonitrile butadiene styrene (ABS) from plastic wastes combined with nitric acid and ascorbic acid in the presence of chitosan at 60°C (Elsayed et al., 2020).
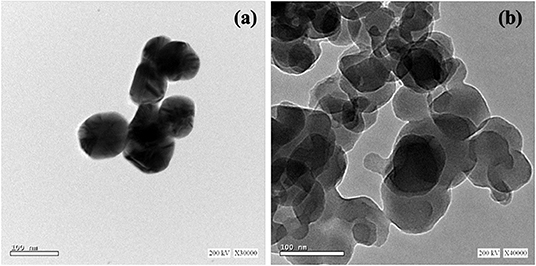
Figure 3. TEM images of (a) Ag and (b) Cu NPs synthesized from metalized plastic waste. The NPs were prepared with leachant solution of metalized acrylonitrile butadiene styrene (ABS) using ascorbic acid as reductant and chitosan as stabilizer according to the methods described by Elsayed et al. (2020). These original images were provided by the authors of the manuscript and have not been previously published.
Researchers also utilize methods to reuse nanoparticles. For example, functionalized nanoparticles were successfully recycled to capture toxins from spiked blood plasma samples by applying a glycine buffer to free up the nanoparticles after their initial use (Hassanain et al., 2017). In another study, zinc oxide (ZnO) nanoparticles were synthesized from spent Zn-C battery via thermal technique at 900°C under an argon atmosphere using a horizontal quartz tube furnace. The produced nanoparticles were spherically shaped within a size range of 50 nm (Figure 4) (Farzana et al., 2018).
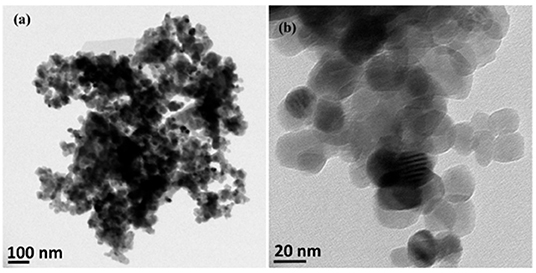
Figure 4. Low resolution (a) and high resolution (b) TEM images of ZnO NPs recovered from spent Zn-C batteries via thermal route. This figure was reproduced from Farzana et al. (2018) under the terms and conditions of the Creative Commons Attribution (CC BY) license (http://creativecommons.org/licenses/by/4.0/).
According to Dutta et al. recycling waste batteries and electronics to recover nanomaterials has ushered in a new era in nanotechnology and environmental research (Dutta et al., 2018).
Carbon Nanotubes
Carbon nanotubes (CNTs) synthesis from waste plastics is carried out in diverse systems, such as autoclaves, quartz tubes, crucibles, and muffle furnaces (Bazargan and McKay, 2012). Each method aims to disintegrate solid-state polymers into their carbon precursors via pyrolysis (Zhuo and Levendis, 2014). Polyolefin (~85.7wt % carbon content) is a polymeric material created from olefin monomer (CnH2n), and it is present in the large majority of plastic waste (Gong et al., 2012). Two waste polyolefins, polypropylene (PP) and polyethylene (PE) can be used for carbon materials synthesis. Other plastics integrated with additional elements such as polyvinyl alcohol (PVA) and polyethylene terephthalate (PET), can also be used for CNTs synthesis (Deng et al., 2016).
Gong et al. developed a new layer-by-layer assembling mechanism. In this method, waste PP was catalyzed via activated carbon and Ni2O3 in a quartz tube reactor. Activated carbon was effective in breaking PP into light hydrocarbons. Activated carbon also provided highly efficient catalytic conversion with Ni by encouraging dehydrogenation and aromatization with the formation of different aromatic groups. CNTs growth was accomplished based on benzene rings. The carboxylic moieties of activated carbon also enabled synergistic catalysis with Ni2O3. The highest carbon yield (~50 wt%) was reached at 820°C with a proportion of raw materials of PP:10Ni2O3:8AC (wt%) (Gong et al., 2012). Considering the high energy demand of this method, the recovery of Ni2O3 could be an alternative for more sustainable scale-up manufacturing.
Zhou et al. reported another combustion method for PE decomposition. This method generates light hydrocarbon in-situ during an exothermic process. This method utilized a stainless-steel wire mesh as a catalyst and a substrate. A ceramic filter was placed before the stainless-steel wire mesh to eliminate the soot that could deactivate the mesh that served as a catalyst. The yield of CNTs was higher than 10 wt% (Zhuo et al., 2010).
In another synthesis process, Zhang et al. mixed PP (~2 g), maleated PP (~0.5 g), and Ni catalysis powders (~0.5 g) in an autoclave. The resulting mixture was then heated for 12 h to a temperature of 700°C using an electric stove. It was then left to cool to ambient temperature. Carbon solid spheres were the only product obtained in absence of Ni from the reaction system which indicated that Ni powder catalyzed the decomposition of PP. MA-PP contributed to two actions in the growth process of CNTs, first improving the dispersion of Ni in PP, and then forming a homogenous system between carbon atoms and Ni catalysts. Ni particles were separated to form carbon-surrounded Ni particles and a high surface packing density of Ni particles enabled the nanotubes to grow along a consistent direction. This method of production of 160 nm CNTs had a yield of 80% (Zhang et al., 2008).
Bajad et al. attained approximately 45.8% yield in the production of MWNTs. These MWNTs were derived from PP waste catalyzed by Ni/Mo/MgO using a combustion technique (Bajad et al., 2015). The powdered catalysts and PP were placed in a covered silicon crucible and heated to 800°C in a muffle furnace. The Ni/Mo ratio was found to affect the yield and size of nanotubes. The HRTEM images exposed that the Ni/Mo ratio controlled both the yield and morphology of CNTs. Increasing the Mo content resulted in large diameter CNTs while lower Mo content gave higher yield with short-radius CNTs. The authors optimized the Ni/Mo mole ratio using response surface methodology (RSM) which proposed that 394% of carbon product could be yielded at a Ni/Mo mole ratio of 22.04. While 514% of CNTs would yield over Ni4Mo0.2MgO1 catalyst at 800°C, 5 g polymer weight, 150 mg catalyst weight, and combustion time of 10 min.
Table 4 summarizes the different methods used in the production of CNTs from plastic polymers commonly found in solid waste.
Graphene
Graphene is a single-atom-thick sheet of sp2 hybridized carbon atoms packed into a honeycomb lattice structure. Prominent properties of this material are high surface area, high electrical conductivity, good chemical stability, and strong mechanical strength (Nair et al., 2008; Rao et al., 2009; Luo et al., 2012; Novoselov et al., 2012). Graphene revolutionized the health, energy, and environment sectors (Liu et al., 2013a; Quesnel et al., 2015; Surwade et al., 2015; Yang et al., 2016a).
Its electrical, optical, and mechanical properties (Bonaccorso et al., 2010; Soldano et al., 2010; Marinho et al., 2012) make graphene eligible for any application. There are two essential sources for the preparation of graphene: graphite and organic molecules (Strudwick et al., 2015). Typical methods for graphene preparation include the bottom-up approach, the top-down approach, epitaxial growth on silicon carbide (Mishra et al., 2016), mechanical cleavage (Bonaccorso et al., 2012), and chemical reduction of graphene oxide (Abdolhosseinzadeh et al., 2015). The bottom-up approach utilizes chemical vapor deposition (CVD) on metallic films (Strudwick et al., 2015). The top-down approach utilizes liquid exfoliation of graphite crystal (Coleman, 2013). Table 5 summarizes the recent approaches for graphene synthesis from waste materials.
Graphene can be synthesized from various waste plastics using a variety of methods. Gong et al. were able to create high yields of graphene flakes. Their method used waste polypropylene (PP) catalyzed by organically modified montmorillonite. A uniform mixture of PP (~89 wt %), talcum (~11 wt %), and modified montmorillonite, was placed in a crucible and heated to 700°C for 15 min to obtain the carbonized char. After cooling the carbonized char was immersed in HF and HNO3. HF dissolved the impurities and HNO3 oxidized the amorphous carbon. After a repeated process of centrifuging and isolating from solution, graphene flakes were obtained (Gong et al., 2014b).
Manukyan et al. developed an energy-saving combustion method to prepare graphene sheets using waste polytetrafluoroethylene (PTFE) and silicon carbide (SiC). The process mechanism was similar to epitaxial growth on SiC, in which Si was removed by C2F4 through an exothermal reaction (Manukyan et al., 2013).
Ruan et al. developed a green synthesis method in which raw wastes including waste polystyrene, grass blades, waste food, and grass, were transformed into high-quality single-layered graphene. In this method, 10 mg of start materials were placed on a slightly bent piece of Cu foil held by a quartz boat in a CVD quartz tube. After low-pressure annealing at a temperature of approximately 1,050°C for 15 min in an inert atmosphere (Ar and H2), graphene growth was observed on the back of the Cu foil (Ruan et al., 2011).
Another CVD based graphene synthesis method used solid waste plastics roughly composed of 86% polyethylene and 14% polystyrene (Sharma et al., 2014). The method used two furnaces. In the first furnace, nearly 3 mg of plastic waste was put into a ceramic boat and kept at a temperature of approximately 500°C. In the second furnace, Cu foil was placed inside as substrate and kept at a temperature of approximately 1,020°C. Following pyrolysis, the degraded carbonaceous compounds were placed into the next furnace in a gas mixture (Ar/H2: 98/2.5 sc cm). The products then interacted with a Cu substrate causing graphene growth. In this method, the injection rate of disintegrated products was crucial for graphene crystal formation. Large hexagonal single-layered graphene was produced at a low rate of pyrolysis and injection. On the other hand, under a high injection rate, a bilayer or multilayer graphene was formed (Sharma et al., 2014).
Environmental Applications of Waste-Derived Nanoparticles
The environmental applications of waste-derived nanoparticles will be discussed in this section and are presented in Table 6.
Wastewater Treatment and Water Remediation
Adsorption
Industrial wastewater is contaminated with a mixture of pollutants unique to the industries that create them. For example, the textile industry produces waste streams contaminated with a variety of dyes. Water contaminated with these dyes must be treated to ensure public and environmental health (Arslan et al., 2016). Several research groups are attempting to find novel nanotechnology-enabled treatments for industrial wastewater using recycled nanoparticles, which helps meet circularity and sustainability goals. Arifin et al. (2017) developed iron oxide nanoparticles from mill scale and applied them to dye removal. In their process, iron oxide particles were removed from the unwanted components of mill scaling using magnetic separation techniques. The particles were then processed using conventional and high energy ball milling and treated with hexadecyltrimethylammonium bromide to prevent aggregation. In dye wastewater, the adsorption by the modified iron nanoparticles was above 99% with optimum adsorption of 99.93% with 53.76 nm particles (Arifin et al., 2017).
Giri et al. (2011) developed a method for creating magnetite (Fe3O4) nanoparticles from iron ore tailings. The formation of these particles was confirmed through powder X-ray diffraction (XRD), ultraviolet-visible spectrophotometry (UV–Vis) and Fourier-transform infrared spectroscopy (FT-IR) spectra. These particles were fast and effective in the removal of methylene blue and Congo red dyes. In optimal conditions, the monolayer adsorption capacities were 70.4 mg g−1 for methylene blue and 172.4 mg g−1 for Congo red. These nanoparticles compared well with particles created from reagent grade materials and as such could be a value-added product with possible applications in large scale wastewater treatment (Giri et al., 2011).
El Essawy et al. (2017) explored another means of removing dye from water using recycled Polyethylene terephthalate (PET) as a starting material for NP production. Through thermal dissociation, PET creates graphene. The graphene was characterized using SEM, TEM, Raman, BET, TGA, and FT-IR. The produced graphene showed high micropore volume and surface area. Potential for adsorption was assessed with methylene blue and acid blue 25 dyes. This graphene showed good adsorption of methylene blue with optimal adsorption at a pH of 12. The methylene blue dye reached equilibrium within 30 min. The adsorption of the acid blue 25 dye was optimal in acidic solutions and the adsorbed dye reached an equilibrium in approximately 50 min. The PET-based graphene showed effectiveness in removing both dyes from solutions (El Essawy et al., 2017).
Wang et al. developed engineered biochar functionalized with waste eggshell particles. The biochar is composed of three types of biomass pretreated with eggshell waste and prepared through slow pyrolysis. The eggshell particles are prepared using a method shown to make colloidal and nanosized eggshell particles (Hassan et al., 2014). Using characterization tools such as scanning electron microscopy eggshell particles were found on the surface and within the pore networks of the biochar. When studied the biochar treated with eggshells was more effective at lead (Pb2+) adsorption than pristine biochar due to the presence of CaCO3 from the eggshells (Wang et al., 2018).
Thai et al. (2019) reviewed the synthesis of highly porous aerogels from recycled materials such as paper, cotton textiles, and plastic bottles. Paper-derived aerogels were made through a simple process that required sonication of a solution of recycled cellulose with plyamide-epichlorohydrin, followed by freeze-drying at −98°C. Similarly, textile-derived aerogels were prepared by blending scraps of cotton cloth with DI water, followed by sonication in a solution of plyamide-epichlorohydrin. The resulting dispersion was freeze-dried at −98°C and cured at 120°C. Aerogels from recycled PET bottles were obtained by immersing PET fibers in a solution of NaOH heated at 80°C; the fibers were subsequently rinsed with DI water and then mixed with a solution of polyvinyl alcohol, glutaraldehyde, and HCl. Next, the mixture was sonicated, heated at 80°C, and freeze-dried. To confer superhydrophobicity to these aerogels, a coating of methyltrimethoxysilane was added at the end of the fabrication process. The ultra-low density and high absorption capacity of these waste-derived aerogels make them especially attractive for remediation applications such as the clean-up of oil spills in water bodies. For example, aerogels from cotton can achieve an absorption capacity above 100 grams of motor oil per gram of aerogel, which significantly outperforms most commercial sorbents (Thai et al., 2019).
Zhang et al. (2020) proposed and tested another method for treating waste dyewater using iron derived from the manufacturing of saccharin. In this process, NiFe2O4 nanoparticles that are used as a catalyst, are first extracted from the saccharin wastewater, which is challenging to treat. A mesoporous magnet NiFe2O4/ ZnCuCr-LDH composite is then created using a hydrothermal method. The created NiFe2O4/ ZnCuCr-LDH composites had a removal efficiency for Congo red of over 97% in cases where the initial concentration of the dye was between 100 and 450 mg l−1. The process treats the saccharin wastewater for iron contamination and reuses the waste iron to create a magnetic composite for the treatment of dye water (Zhang et al., 2020).
Rovani et al. and Peres et al. proposed methods of creating silicon nanoparticles from agricultural waste for the absorption of dyes. Rovani et al. created high purity silica nanoparticles (SiO2NPs) from sugar cane waste ash. The nanoparticles were tested for their capacity as absorbent material for the removal of acid orange 8 (AO8) dye. The silicon nanoparticles had an absorption capacity of 230 mg/g and the nanoparticles were able to be reused for up to five cycles (Rovani et al., 2018). Peres et al. synthesized silica nanoparticles from corn husk waste through a standard synthesis and a microwave synthesis method. These silicon nanoparticles were applied for the absorption of methyl blue dye. The microwave silica nanoparticles show higher values for surface area, poor volume, pore diameter, porosity, and purity compared to the traditionally synthesized silica nanoparticles. The microwave silica nanoparticles had an absorption capacity of 679.9 mg/g and a removal percentage of 80%. The thermodynamic results for the absorbent revealed a favorable spontaneous exothermic reaction for both nano-silica particles (Peres et al., 2018).
Photocatalytic Degradation
Nayak et al. (2019) synthesized a nanocomposite of ZnO and CuO using electronic waste, specifically printed circuit boards. These composites were formed by applying nitric acid to the memory slots and processing the leached liquid using an alkaline hydrothermal treatment. The composites were characterized using high-resolution transmission electron microscopy (HR-TEM), diffuse reflectance ultraviolet-visible spectrophotometry (UV-DRS), and UV–Vis. The nanocomposites were composed of CuO cores with ZnO nanostructures precipitated onto the cores. The nanoparticles proved to be effective photocatalyst for methyl orange dye degradation in the presence of visible light and hydrogen peroxide. These nanoparticles show promise as photo-Fenton catalysts for organic pollutants (Nayak et al., 2019).
Wu et al. explored another strategy for the photocatalytic degradation of pollutants in wastewater. Wu et al. prepared a metals-doped ZnO (M-ZnO) nanomaterial using fabric filter dust. The dopants used (Fe, Mg, Ca, and Al) and the zinc were all obtained from fabric filter dust without the addition of chemicals as elemental sources. The doped M-ZnO nanoparticles were prepared through sulfolysis combining co-precipitation processes. The nanoparticle acts as a favorable photocatalyst for the breakdown of organic substances, specifically phenol, under visible light irradiation (Wu et al., 2014).
Decomposition
Lee et al. studied the removal of nitrobenzene using synthesized zero-valent iron nanoparticles. The iron oxide to synthesize the nanoparticles comes from the pickling line of a steel plant. These particles are approximately 500 nm. The particles showed a reaction activity much higher than commercial zero-valent iron. Lee et al. proposed that in presence of the synthesized zero-valent iron nitrobenzene deoxidizes to nitrosobenzene. The nitrosobenzene is then reduced to aniline which is more biodegradable than nitrobenzene. When combined with biological processes these nanoparticles show promise in decomposing nitrobenzene in wastewater (Lee et al., 2015).
Monitoring of Pollutants in Water
Laboratory tests utilized to identify pollutants in water are cost-prohibitive in some communities because they require trained personnel and expensive equipment to run. To address this problem, researchers are developing low-cost sensors to monitor pollutants using nanoparticles. Some researchers take it a step further using recycled nanoparticles. Lu et al. (2012) developed an optical sensor that uses recycled carbon nanoparticles from waste pomelo peels. Pomelo (Citrus maximus) has a grapefruit-like flavor with a much thicker peel and is a popular fruit from Brazil to Southeast Asia. The particles, derived from the hydrothermal processing of the pomelo peels, need no further chemical modification. The carbon particles have a reported quantum yield of approximately 6.9%. These particles were tested for use in the detection of mercury (Hg2+) based on the mercury-induced fluorescence quenching of the carbon particles. The particles' selectivity and sensitivity were excellent, and the particles had a limit of detection as low as 0.23 nM. The carbon nanoparticles have also been successful in mercury detection in lake water samples (Lu et al., 2012).
Abdelbasir et al. (2018a) developed an electrochemical sensor for environmental protection applications. The sensor was fabricated by anchoring copper nanoparticles to laser-scribed graphene electrodes. The nano-cuprous oxide was synthesized from electrical waste. The unique structure of the nanoparticles made them stable, linker-free, and size-tunable. The anchoring of the particles to the graphene electrode reduced internal/charge transfer resistance enhancing electrochemical performance. The effectiveness of the sensors in detecting dopamine and mercury was evaluated. In the case of dopamine, the sensor shows a linear calibration curve between 300 nM and 5 uM, a limit of detection of 200 nM, a sensitivity of 30 nA μM−1 cm−2, and a response time of 2.4 ± 0.7 s. In the case of mercury, the sensor shows a linear calibration curve between 0.02 and 2.5 ppm, a limit of detection of 25 ppb, a sensitivity of 10 nA ppm−1, and a response time of fewer than 3 min. The proposed approach presented to fabricate these sensors is scalable as wires are used in a variety of commonplace goods and industries (Abdelbasir et al., 2018a).
Capture of Air Pollutants
Zeng et al. tested a means of deriving porous silica nanoparticles (PSNs) from rice husks with a simple template-free method. This method is inexpensive, fast, simple, and energy saving. (NH4)2SiF6 salt formed during the synthetic process serve as a porogen. Thus, providing control of the porosity of the PSNs without the need for extra pore-directing templates and post-heat-treatment by varying the molar ratios of HF/Si and NH4OH/Si. The PSNs were evaluated as support for polyethyleneimine (PEI). The PEI/silica composite reached an adsorption capacity of 159 mg/g at 75°C under 15% CO2. According to Zeng et al. this is superior to waste silica precursors reported in the literature. The sorbent also showed high stability during 20 cycles of absorption and desorption. This implies that it has potential in post combustions CO2 capture. The silica used could also be sourced from other waste streams such as bottom ash and fly ash (Zeng and Bai, 2016).
Sustainability Considerations and Concluding Remarks
Throughout this review, different possibilities for using waste materials as inputs for the production of NPs have been exemplified and discussed in the context of research. While the concept of turning waste into advanced technologies for environmental applications may, at first glance, look like an attractive full-circle approach, there are significant knowledge gaps about engineered nanomaterials that should be addressed before transitioning these ideas from the lab to the real world; particularly regarding energy use, generation of secondary wastes, fate and transport behavior, exposure routes in different environments, and toxicity levels in diverse organisms (Kumar et al., 2014). As viable processes emerge from the proof-of-concept stage, careful cost-benefit analyses must be conducted. Though there is no simple path for overcoming the negative implications of the current “end-of-life” waste management systems, many experts have highlighted the importance of conducting life cycle assessments and risk analysis early on during the development of new technologies and processes, and also, to account for aging transformations and potential release of nanomaterials and byproducts in the environment. Thus, minimizing the potential for the future unveiling of unintended consequences (Dhingra et al., 2010; Grillo et al., 2015; Mitrano et al., 2015). However, performing the pertinent life cycle assessment and risk analyses implies having access to a sufficiently large body of data on emissions and environmental concentrations on engineered NPs. Hence, most data available in the literature have been obtained through modeling and simulation of the release of NPs from containing products during consumer manipulation; thus, empirical information on release coefficients throughout all stages of the life cycle (including production, usage, and disposal) is a limiting factor (Aitken et al., 2006; Gottschalk and Nowack, 2011).
At the production stage, the twelve principles of green chemistry provide a suitable framework for manufacturing NPs and NP-enabled technologies. These principles are intended to guide the design of chemical routes that yield enhanced sustainability outcomes (Gilbertson et al., 2015; Benelli, 2019). Nevertheless, the fact that NPs can be produced through sustainable approaches does not mean that the fabricated NPs are intrinsically safe and that their use and release in the environment will not cause harm. In the last decade, some studies have shown that engineered NPs that have already been used in different applications, including food manufacturing and packaging, could dramatically disrupt epigenetic mechanisms, and there are several questions regarding their ability to induce diseases (Smolkova et al., 2015). Perhaps, fullerenes are amongst the better-known class of engineered NPs. Several in vitro and in vivo studies have reported on the interactions and toxicity of carbon nanomaterials with various living systems (Liu et al., 2013b; Lalwani et al., 2016). Kagan et al. (2005) discussed how the very same properties that make CNTs so desirable for technological applications are also associated with inflammatory reactions and fibrogenic events that impair lung function in mice making their respiratory system more prone to infections (Kagan et al., 2005). The effects of inhalation of CNTs have been compared to those of asbestos fibers, which are known cytotoxic and carcinogenic particles (Sanchez et al., 2009).
In conclusion, to realize the potential benefits from the implementation of waste-derived NPs, a complex web of influencing factors must be understood, and decisions should err on the side of precaution and ethics. Scale and scope of the applications of these NPs, as well as strategies to enable closed-loop management of the engineered nanomaterials, should be defined on a regional basis and management policies should be informed by health and environmental science.
Author Contributions
DV outlined and lead the drafting and editing of the manuscript. SA and KM performed an extensive literature search and contributed to the writing of sections and the construction of figures and tables. CL provided professional advice and participated in the revision of the final document. All authors read and approved the final manuscript.
Funding
The authors would like to acknowledge the support by the National Science Foundation (GRFPF award 2014124, for KM), the DUPC2-IHE program at Delft University (P: 1084731:111; Ref: 2019/061/108473/EWH), and the Open Access Publishing Initiative of Clemson University.
Conflict of Interest
The authors declare that the research was conducted in the absence of any commercial or financial relationships that could be construed as a potential conflict of interest.
References
Abdelbasir, S. M., El-Sheikh, S. M., Morgan, V. L., Schmidt, H., Casso-Hartmann, L. M., Vanegas, D. C., et al. (2018a). Graphene-anchored cuprous oxide nanoparticles from waste electric cables for electrochemical sensing. ACS Sustain. Chem. Eng. 6, 12176–12186. doi: 10.1021/acssuschemeng.8b02510
Abdelbasir, S. M., El-Sheltawy, C. T., and Abdo, D. M. (2018b). Green processes for electronic waste recycling: a review. J. Sustain. Metall. 4, 295–311. doi: 10.1007/s40831-018-0175-3
Abdelbasir, S. M., Hassan, S. S. M., Kamel, A. H., and El-Nasr, R. S. (2018c). Status of electronic waste recycling techniques: a review. Environ. Sci. Pollut. Res. 25, 16533–16547. doi: 10.1007/s11356-018-2136-6
Abdolhosseinzadeh, S., Asgharzadeh, H., and Kim, H. S. (2015). Fast and fully-scalable synthesis of reduced graphene oxide. Sci. Rep. 5, 1–7. doi: 10.1038/srep10160
Aboul-Enein, A. A., Awadallah, A. E., Abdel-Rahman, A. A. H., and Haggar, A. M. (2018). Synthesis of multi-walled carbon nanotubes via pyrolysis of plastic waste using a two-stage process. Fullerenes Nanotub. Carbon Nanostructures 26, 443–450. doi: 10.1080/1536383X.2018.1447929
Aboulkas, A., El harfi, K., and El Bouadili, A. (2010). Thermal degradation behaviors of polyethylene and polypropylene. Part I: pyrolysis kinetics and mechanisms. Energy Convers. Manag. 51, 1363–1369. doi: 10.1016/j.enconman.2009.12.017
Ackerman, F. (2000). Waste management and climate change. Local Environ. 5, 223–229. doi: 10.1080/13549830050009373
Aitken, R. J., Chaudhry, M. Q., Boxall, A. B. A., and Hull, M. (2006). Manufacture and use of nanomaterials: current status in the UK and global trends. Occup. Med. (Chic. Ill). 56, 300–306. doi: 10.1093/occmed/kql051
Al-Salem, S. M., Lettieri, P., and Baeyens, J. (2009). Recycling and recovery routes of plastic solid waste (PSW): a review. Waste Manag. 29, 2625–2643. doi: 10.1016/j.wasman.2009.06.004
Arifin, S. A. R. A., Ismail, I., Halim Abdullah, A., Nabilah Shafiee, F., Nazlan, R., and Riati Ibrahim, I. (2017). Iron oxide nanoparticles derived from mill scale waste as potential scavenging agent in dye wastewater treatment for batik industry. Solid State Phenom. 268, 393–398. doi: 10.4028/www.scientific.net/SSP.268.393
Arslan, S., Eyvaz, M., Gürbulak, E., and Yüksel, E. (2016). “A review of state-of-the-art technologies in dye-containing wastewater treatment – the textile industry case,” in Textile Wastewater Treatment. 1–29. doi: 10.5772/64140
Astrup, T., Fruergaard, T., and Christensen, T. H. (2009). Recycling of plastic: accounting of greenhouse gases and global warming contributions. Waste Manag. Res. 27, 763–772. doi: 10.1177/0734242X09345868
Bajad, G. S., Tiwari, S. K., and Vijayakumar, R. P. (2015). Synthesis and characterization of CNTs using polypropylene waste as precursor. Mater. Sci. Eng. B Solid-State Mater. Adv. Technol. 194, 68–77. doi: 10.1016/j.mseb.2015.01.004
Baldé, C. P., Forti, V., Gray, V., Kuehr, R., and Stegmann, P. (2017). Quantities, Flows, and Resources The Global E-waste. Available online at: www.unu.edu (accessed May 10, 2020).
Banu, J. R., Sharmila, V. G., Ushani, U., Amudha, V., and Kumar, G. (2020). Impervious and influence in the liquid fuel production from municipal plastic waste through thermo-chemical biomass conversion technologies - A review. Sci. Total Environ. 718:137287. doi: 10.1016/j.scitotenv.2020.137287
Barletta, I., Johansson, B., Cullbrand, K., Björkman, M., and Reimers, J. (2015). “Fostering sustainable electronic waste management through intelligent sorting equipment,” in IEEE International Conference on Automation Science and Engineering (CASE) (Gothenburg), 459–461. doi: 10.1109/CoASE.2015.7294122
Baruah, S., and Dutta, J. (2009). Nanotechnology applications in pollution sensing and degradation in agriculture. Environ. Chem. Lett. 7, 191–204. doi: 10.1007/s10311-009-0228-8
Basel Action Network (2019). Norwegian Proposal' Puts Brakes on Global Plastic Waste ‘Tsunami. 2020. Available online at: https://www.ban.org/news/2019/5/10/basel-convention-agrees-to-control-plastic-waste-trade (accessed May 10 2020).
Bazargan, A., and McKay, G. (2012). A review - Synthesis of carbon nanotubes from plastic wastes. Chem. Eng. J. 195–196, 377–391. doi: 10.1016/j.cej.2012.03.077
Bennett, J. A., Wilson, K., and Lee, A. F. (2016). Catalytic applications of waste derived materials. J. Mater. Chem. A 4, 3617–3637. doi: 10.1039/C5TA09613H
Berg, A., Antikainen, R., Hartikainen, E., Kauppi, S., Kautto, P., Lazarevic, D., et al. (2018). Circular Economy for Sustainable Development. Helsinki: Finnish Environment Institute.
Berkmans, J. A., Jagannatham, M., Priyanka, S., and Haridoss, P. (2014). Synthesis of branched, nano channeled, ultrafine and nano carbon tubes from PET wastes using the arc discharge method. Waste Manag. 34, 2139–2145. doi: 10.1016/j.wasman.2014.07.004
Bhat, V., Rao, P., and Patil, Y. (2012). Development of an integrated model to recover precious metals from electronic scrap - a novel strategy for E-Waste management. Procedia. Soc. Behav. Sci. 37, 397–406. doi: 10.1016/j.sbspro.2012.03.305
Bindraban, P. S., Dimkpa, C., Nagarajan, L., Roy, A., and Rabbinge, R. (2015). Revisiting fertilisers and fertilisation strategies for improved nutrient uptake by plants. Biol. Fertil. Soils 51, 897–911. doi: 10.1007/s00374-015-1039-7
Blazsó, M., Czégény, Z., and Csoma, C. (2002). Pyrolysis and debromination of flame retarded polymers of electronic scrap studied by analytical pyrolysis. J. Anal. Appl. Pyrolysis 64, 249–261. doi: 10.1016/S0165-2370(02)00035-9
Bonaccorso, F., Lombardo, A., Hasan, T., Sun, Z., Colombo, L., and Ferrari, A. C. (2012). Production and processing of graphene and 2d crystals. Mater. Today 15, 564–589. doi: 10.1016/S1369-7021(13)70014-2
Bonaccorso, F., Sun, Z., Hasan, T., and Ferrari, A. C. (2010). Graphene photonics and optoelectronics. Nat. Publ. Gr. 4, 611–622. doi: 10.1038/nphoton.2010.186
Bourguignon, D. (2014). Turning Waste Into a Resource - Moving Towards a “Circular Economy” - Think Tank. Brief. Eur. Parliam. Available online at: https://www.europarl.europa.eu/thinktank/en/document.html?reference=EPRS_BRI(2014)545704 (accessed May 10, 2020).
Bouwmeester, H., Hollman, P. C. H., and Peters, R. J. B. (2015). Potential health impact of environmentally released micro- and nanoplastics in the human food production chain: experiences from nanotoxicology. Environ. Sci. Technol. 49, 8932–8947. doi: 10.1021/acs.est.5b01090
Calgaro, C. O., Schlemmer, D. F., Da Silva, M. D. C. R., Maziero, E. V., Tanabe, E. H., and Bertuol, D. A. (2015). Fast copper extraction from printed circuit boards using supercritical carbon dioxide. Waste Manag. 45, 289–297. doi: 10.1016/j.wasman.2015.05.017
Cayumil, R., Khanna, R., Ikram-Ul-Haq, M., Rajarao, R., Hill, A., and Sahajwalla, V. (2014). Generation of copper rich metallic phases from waste printed circuit boards. Waste Manag. 34, 1783–1792. doi: 10.1016/j.wasman.2014.05.004
Chaukura, N., Gwenzi, W., Bunhu, T., Ruziwa, D. T., and Pumure, I. (2016). Potential uses and value-added products derived from waste polystyrene in developing countries: a review. Resour. Conserv. Recycl. 107, 157–165. doi: 10.1016/j.resconrec.2015.10.031
Chen, D., Li, Q., Shao, L., Zhang, F., and Qian, G. (2016). Recovery and application of heavy metals from pickling waste liquor (PWL) and electroplating wastewater (EPW) by the combination process of ferrite nanoparticles. Desalin. Water Treat. 57, 29264–29273. doi: 10.1080/19443994.2016.1172984
Chen, M., Huang, J., Ogunseitan, O. A., Zhu, N., and Wang, Y. (2015a). Comparative study on copper leaching from waste printed circuit boards by typical ionic liquid acids. Waste Manag. 41, 142–147. doi: 10.1016/j.wasman.2015.03.037
Chen, M., Zhang, S., Huang, J., and Chen, H. (2015b). Lead during the leaching process of copper from waste printed circuit boards by five typical ionic liquid acids. J. Clean. Prod. 95, 142–147. doi: 10.1016/j.jclepro.2015.02.045
Chu, Y., Chen, M., Chen, S., Wang, B., Fu, K., and Chen, H. (2015). Micro-copper powders recovered from waste printed circuit boards by electrolysis. Hydrometallurgy 156, 152–157. doi: 10.1016/j.hydromet.2015.06.006
Coleman, J. N. (2013). Liquid exfoliation of defect-free graphene. Acc. Chem. Res. 46, 14–22. doi: 10.1021/ar300009f
Cui, H., and Anderson, C. G. (2016). Literature review of hydrometallurgical recycling of printed circuit boards (PCBs). J. Adv. Chem. Eng. 6, 1–11. doi: 10.4172/2090-4568.1000142
Cui, J., and Forssberg, E. (2003). Mechanical recycling of waste electric and electronic equipment: a review. J. Hazard. Mater. 99, 243–263. doi: 10.1016/S0304-3894(03)00061-X
Cui, L., Wang, X., Chen, N., Ji, B., and Qu, L. (2017). Trash to treasure: converting plastic waste into a useful graphene foil. Nanoscale 9, 9089–9094. doi: 10.1039/C7NR03580B
Dalrymple, I., Wright, N., Kellner, R., Bains, N., Geraghty, K., Goosey, M., et al. (2007). An integrated approach to electronic waste (WEEE) recycling. Circuit World 33, 52–58. doi: 10.1108/03056120710750256
Dell, J. (2019). 157,000 Shipping Containers of U.S. Plastic Waste Exported to Countries with Poor Waste Management in 2018 — Plastic Pollution Coalition. Available online at: https://www.plasticpollutioncoalition.org/blog/2019/3/6/157000-shipping-containers-of-us-plastic-waste-exported-to-countries-with-poor-waste-management-in-2018 (accessed May 10, 2020).
Deng, J., You, Y., Sahajwalla, V., and Joshi, R. K. (2016). Transforming waste into carbon-based nanomaterials. Carbon N. Y. 96, 105–115. doi: 10.1016/j.carbon.2015.09.033
Dhingra, R., Naidu, S., Upreti, G., and Sawhney, R. (2010). Sustainable nanotechnology: through green methods and life-cycle thinking. Sustainability 2, 3323–3338. doi: 10.3390/su2103323
Duan, C., Wen, X., Shi, C., Zhao, Y., Wen, B., and He, Y. (2009). Recovery of metals from waste printed circuit boards by a mechanical method using a water medium. J. Hazard. Mater. 166, 478–482. doi: 10.1016/j.jhazmat.2008.11.060
Dubin, S., Gilje, S., Wang, K., Tung, V. C., Cha, K., Hall, A. S., et al. (2010). A one-step, solvothermal reduction method for producing reduced graphene oxide dispersions in organic solvents. ACS Nano 4, 3845–3852. doi: 10.1021/nn100511a
Dutta, T., Kim, K. H., Deep, A., Szulejko, J. E., Vellingiri, K., Kumar, S., et al. (2018). Recovery of nanomaterials from battery and electronic wastes: a new paradigm of environmental waste management. Renew. Sustain. Energy Rev. 82, 3694–3704. doi: 10.1016/j.rser.2017.10.094
El Essawy, N. A., Ali, S. M., Farag, H. A., Konsowa, A. H., Elnouby, M., and Hamad, H. A. (2017). Green synthesis of graphene from recycled PET bottle wastes for use in the adsorption of dyes in aqueous solution. Ecotoxicol. Environ. Saf. 145, 57–68. doi: 10.1016/j.ecoenv.2017.07.014
El-Nasr, S. R., Abdelbasir, S. M., Kamel, A. H., and Hassan, S. S. M. (2020). Environmentally friendly synthesis of copper nanoparticles from waste printed circuit boards. Sep. Purif. Technol. 230, 115860. doi: 10.1016/j.seppur.2019.115860
Elsayed, D. M., Abdelbasir, S. M., Abdel-Ghafar, H. M., Salah, B. A., and Sayed, S. A. (2020). Silver and copper nanostructured particles recovered from metalized plastic waste for antibacterial applications. J. Environ. Chem. Eng. 8:103826. doi: 10.1016/j.jece.2020.103826
Evans, A., and Evans, R. (2006). The Composition of a Tyre: Typical Components Creating markets for recycled resources. Waste Resour. Action Program. 1–5. Available online at: https://nanopdf.com/download/the-composition-of-a-tyre-typical-components_pdf (accessed May 10, 2020).
Falinski, M. M., Plata, D. L., Chopra, S. S., Theis, T. L., Gilbertson, L. M., and Zimmerman, J. B. (2018). A framework for sustainable nanomaterial selection and design based on performance, hazard, and economic considerations. Nat. Nanotechnol. 13, 708–714. doi: 10.1038/s41565-018-0120-4
Fan, C., An, H., Du, J., and Luo, Y. (2020). High-performance printable paper-like composites derived from plastic flexible film wastes. Polym. Int. 69, 184–191. doi: 10.1002/pi.5935
Fang, Z., Qiu, X., Chen, J., and Qiu, X. (2011). Degradation of the polybrominated diphenyl ethers by nanoscale zero-valent metallic particles prepared from steel pickling waste liquor. Desalination 267, 34–41. doi: 10.1016/j.desal.2010.09.003
Farzana, R., Rajarao, R., Behera, P. R., Hassan, K., and Sahajwalla, V. (2018). Zinc oxide nanoparticles from waste Zn-C battery via thermal route: characterization and properties. Nanomaterials 8:717. doi: 10.3390/nano8090717
Ferronato, N., and Torretta, V. (2019). Waste mismanagement in developing countries: a review of global issues. Int. J. Environ. Res. Public Health 16:1060. doi: 10.3390/ijerph16061060
Fogarasi, S., Imre-Lucaci, F., Egedy, A., Imre-Lucaci, Á., and Ilea, P. (2015). Eco-friendly copper recovery process from waste printed circuit boards using Fe3+/Fe2+ redox system. Waste Manag. 40, 136–143. doi: 10.1016/j.wasman.2015.02.030
Gilbertson, L. M., Zimmerman, J. B., Plata, D. L., Hutchison, J. E., and Anastas, P. T. (2015). Designing nanomaterials to maximize performance and minimize undesirable implications guided by the Principles of Green Chemistry. Chem. Soc. Rev. 44, 5758–5777. doi: 10.1039/C4CS00445K
Giri, S. K., Das, N. N., and Pradhan, G. C. (2011). Synthesis and characterization of magnetite nanoparticles using waste iron ore tailings for adsorptive removal of dyes from aqueous solution. Colloids Surfaces A Physicochem. Eng. Asp. 389, 43–49. doi: 10.1016/j.colsurfa.2011.08.052
Goh, P. S., Ng, B. C., Lau, W. J., and Ismail, A. F. (2015). Inorganic nanomaterials in polymeric ultrafiltration membranes for water treatment. Sep. Purif. Rev. 44, 216–249. doi: 10.1080/15422119.2014.926274
GoldsteinResearch (2020). Global Tire Recycling Market Report - Edition 2020. Available online at: https://www.goldsteinresearch.com/report/global-tire-recycling-industry-market-trends-analysis (accessed May 10, 2020).
Gómez-Hernández, R., Panecatl-Bernal, Y., and Méndez-Rojas, M. Á. (2019). High yield and simple one-step production of carbon black nanoparticles from waste tires. Heliyon 5:e02139. doi: 10.1016/j.heliyon.2019.e02139
Gondal, M. A., and Siddiqui, M. N. (2007). Identification of different kinds of plastics using laser-induced breakdown spectroscopy for waste management. J. Environ. Sci. Heal. Part A Toxic/Hazardous Subst. Environ. Eng. 42, 1989–1997. doi: 10.1080/10934520701628973
Gong, J., Liu, J., Jiang, Z., Feng, J., Chen, X., Wang, L., et al. (2014a). Striking influence of chain structure of polyethylene on the formation of cup-stacked carbon nanotubes/carbon nanofibers under the combined catalysis of CuBr and NiO. Appl. Catal. B Environ. 147, 592–601. doi: 10.1016/j.apcatb.2013.09.044
Gong, J., Liu, J., Wan, D., Chen, X., Wen, X., Mijowska, E., et al. (2012). Catalytic carbonization of polypropylene by the combined catalysis of activated carbon with Ni2O3 into carbon nanotubes and its mechanism. Appl. Catal. A Gen. 449, 112–120. doi: 10.1016/j.apcata.2012.09.028
Gong, J., Liu, J., Wen, X., Jiang, Z., Chen, X., Mijowska, E., et al. (2014b). Upcycling waste polypropylene into graphene flakes on organically modified montmorillonite. Ind. Eng. Chem. Res. 53, 4173–4181. doi: 10.1021/ie4043246
Gottschalk, F., and Nowack, B. (2011). The release of engineered nanomaterials to the environment. J. Environ. Monit. 13, 1145–1155. doi: 10.1039/c0em00547a
Grillo, R., Rosa, A. H., and Fraceto, L. F. (2015). Engineered nanoparticles and organic matter: a review of the state-of-the-art. Chemosphere 119, 608–619. doi: 10.1016/j.chemosphere.2014.07.049
Gu, L., and Ozbakkaloglu, T. (2016). Use of recycled plastics in concrete: a critical review. Waste Manag. 51, 19–42. doi: 10.1016/j.wasman.2016.03.005
Hadi, P., Xu, M., Lin, C. S. K., Hui, C. W., and McKay, G. (2015). Waste printed circuit board recycling techniques and product utilization. J. Hazard. Mater. 283, 234–243. doi: 10.1016/j.jhazmat.2014.09.032
Hassan, T. A., Rangari, V. K., and Jeelani, S. (2014). Value-added biopolymer nanocomposites from waste eggshell-based CaCO 3 nanoparticles as fillers. ACS Sustain. Chem. Eng. 2, 706–717. doi: 10.1021/sc400405v
Hassanain, W. A., Izake, E. L., Schmidt, M. S., and Ayoko, G. A. (2017). Gold nanomaterials for the selective capturing and SERS diagnosis of toxins in aqueous and biological fluids. Biosens. Bioelectron. 91, 664–672. doi: 10.1016/j.bios.2017.01.032
Hsu, E., Barmak, K., West, A. C., and Park, A. H. A. (2019). Advancements in the treatment and processing of electronic waste with sustainability: a review of metal extraction and recovery technologies. Green Chem. 21, 919–936. doi: 10.1039/C8GC03688H
Hussein, A. K. (2015). Applications of nanotechnology in renewable energies - A comprehensive overview and understanding. Renew. Sustain. Energy Rev. 42, 460–476. doi: 10.1016/j.rser.2014.10.027
Jeevanandam, J., Chan, Y. S., and Danquah, M. K. (2016). Biosynthesis of metal and metal oxide nanoparticles. Chem. Bio. Eng. Rev. 3, 55–67. doi: 10.1002/cben.201500018
Kagan, V. E., Bayir, H., and Shvedova, A. A. (2005). Nanomedicine and nanotoxicology: two sides of the same coin. Nanomed. Nanotechnol. Biol. Med. 1, 313–316. doi: 10.1016/j.nano.2005.10.003
Kaza, S., Yao, L., Bhada-Tata, P., Van Woerden, F., and Ionkova, K. (2018). What a Waste 2.0: a Global Snapshot of Solid Waste Management to 2050. Washington, DC: World Bank. doi: 10.1596/978-1-4648-1329-0
Keskisaari, A., and Kärki, T. (2017). Raw material potential of recyclable materials for fiber composites: a review study. J. Mater. Cycles Waste Manag. 19, 1136–1143. doi: 10.1007/s10163-016-0511-2
Khaloo, S. S., Torabbeigi, M., Jazani, R. K., Douraghi, M., and Ghalavand, Z. (2013). Laboratory waste minimization by recovery of silver as nano-silver colloidal dispersion from waste silver chloride. J. Mater. Cycles Waste Manag. 15, 342–347. doi: 10.1007/s10163-013-0123-z
Ko, S., Kwon, Y. J., Lee, J. U., and Jeon, Y. P. (2020). Preparation of synthetic graphite from waste PET plastic. J. Ind. Eng. Chem. 83, 449–458. doi: 10.1016/j.jiec.2019.12.018
Kral, U., Morf, L. S., Vyzinkarova, D., and Brunner, P. H. (2019). Cycles and sinks: two key elements of a circular economy. J. Mater. Cycles Waste Manag. 21, 1–9. doi: 10.1007/s10163-018-0786-6
Kumar, A., Kumar, P., Anandan, A., Fernandes, T. F., Ayoko, G. A., and Biskos, G. (2014). Engineered nanomaterials: knowledge gaps in fate, exposure, toxicity, and future directions. J. Nanomater.. 130198. doi: 10.1155/2014/130198
Kumar, S., Panda, A. K., and Singh, R. K. (2011). A review on tertiary recycling of high-density polyethylene to fuel. Resour. Conserv. Recycl. 55, 893–910. doi: 10.1016/j.resconrec.2011.05.005
Lalwani, G., D'Agati, M., Khan, A. M., and Sitharaman, B. (2016). Toxicology of graphene-based nanomaterials. Adv. Drug Deliv. Rev. 105, 109–144. doi: 10.1016/j.addr.2016.04.028
Lee, H., Kim, B.-H., Park, Y.-K., Kim, S.-J., and Jung, S.-C. (2015). Application of recycled zero-valent iron nanoparticle. J. Nanomater. 2015:392537. doi: 10.1155/2015/392537
Li, J., Lu, H., Guo, J., Xu, Z., and Zhou, Y. (2007). Recycle technology for recovering resources and products from waste printed circuit boards. Environ. Sci. Technol. 41, 1995–2000. doi: 10.1021/es0618245
Liu, J., Cui, L., and Losic, D. (2013a). Graphene and graphene oxide as new nanocarriers for drug delivery applications. Acta Biomater. 9, 9243–9257. doi: 10.1016/j.actbio.2013.08.016
Liu, Y., Zhao, Y., Sun, B., and Chen, C. (2013b). Understanding the toxicity of carbon nanotubes. Acc. Chem. Res. 46, 702–713. doi: 10.1021/ar300028m
Long, L., Sun, S., Zhong, S., Dai, W., Liu, J., and Song, W. (2010). A novel approach to recycling of glass fibers from nonmetal materials of waste printed circuit boards. J. Hazard. Mater. 177, 626–638. doi: 10.1016/j.jhazmat.2009.12.078
Lu, W., Qin, X., Liu, S., Chang, G., Zhang, Y., Luo, Y., et al. (2012). Economical, green synthesis of fluorescent carbon nanoparticles and their use as probes for sensitive and selective detection of mercury(II) ions. Anal. Chem. 84, 5351–5357. doi: 10.1021/ac3007939
Luda, P. M. (2011). Recycling of printed circuit boards. Integr. Waste Managem. Vol. II (InTech) 285–298.
Luo, B., Liu, S., and Zhi, L. (2012). Chemical approaches toward graphene-based nanomaterials and their applications in energy-related areas. Small 8, 630–646. doi: 10.1002/smll.201101396
Mankhand, T. R., Singh, K. K., Gupta, K., and Das, S. (2012). Pyrolysis of printed circuit boards. Int. J. M etallurgical Eng. 1, 102–107. doi: 10.5923/j.ijmee.20120106.01
Manukyan, K. V., Rouvimov, S., Wolf, E. E., and Mukasyan, A. S. (2013). Combustion synthesis of graphene materials. Carbon N. Y. 62, 302–311. doi: 10.1016/j.carbon.2013.06.014
Marek, A. A., Zawadiak, J., Piotrowski, T., and Hefczyc, B. (2015). A new efficient method for the processing of post-consumer polypropylene and other polyolefin wastes into polar waxes. Waste Manag. 46, 62–67. doi: 10.1016/j.wasman.2015.08.042
Marinho, B., Ghislandi, M., Tkalya, E., Koning, C. E., and de With, G. (2012). Electrical conductivity of compacts of graphene, multi-wall carbon nanotubes, carbon black, and graphite powder. Powder Technol. 221, 351–358. doi: 10.1016/j.powtec.2012.01.024
Maroufi, S., Mohannad, M., and Veena, S. (2017). Nano-carbons from waste tyre rubber: an insight into structure and morphology. Waste Managem. 69, 110–116. doi: 10.1016/j.wasman.2017.08.020
Mata, M. T., Martins, A. A., Costa, A. V. C., and Sikdar, K. S. (2015). Nanotechnology and Sustainability - Current Status and Future Challenges. Life Cycle Anal. Nanoparticles. Risk Assessment Sustain. DEStech Publ. 271–306. Available online at: https://www.researchgate.net/profile/Antonio_Martins2/publication/280836162_Nanotechnology_and_Sustainability-_Current_Status_and_Future_Challenges/links/55c8a54b08aea2d9bdc9158c.pdf
Mdlovu, N. V., Chiang, C. L., Lin, K. S., and Jeng, R. C. (2018). Recycling copper nanoparticles from printed circuit board waste etchants via a microemulsion process. J. Clean. Prod. 185, 781–796. doi: 10.1016/j.jclepro.2018.03.087
Mishra, N., Boeckl, J., Motta, N., and Iacopi, F. (2016). Graphene growth on silicon carbide: a review. Phys. Status Solidi 213, 2277–2289. doi: 10.1002/pssa.201600091
Mishra, N., Das, G., Ansaldo, A., Genovese, A., Malerba, M., Povia, M., et al. (2012). Pyrolysis of waste polypropylene for the synthesis of carbon nanotubes. J. Anal. Appl. Pyrolysis 94, 91–98. doi: 10.1016/j.jaap.2011.11.012
Mitrano, D. M., Motellier, S., Clavaguera, S., and Nowack, B. (2015). Review of nanomaterial aging and transformations through the life cycle of nano-enhanced products. Environ. Int. 77, 132–147. doi: 10.1016/j.envint.2015.01.013
Moghaddasi, S., Khoshgoftarmanesh, A. H., Karimzadeh, F., and Chaney, R. L. (2013). Preparation of nano-particles from waste tire rubber and evaluation of their effectiveness as zinc source for cucumber in nutrient solution culture. Sci. Hortic. (Amsterdam). 160, 398–403. doi: 10.1016/j.scienta.2013.06.028
Nair, R. R., Blake, P., Grigorenko, A. N., Novoselov, K. S., Booth, T. J., Stauber, T., et al. (2008). Fine structure constant defines visual transparency of graphene. Science 80, 320–1308. doi: 10.1126/science.1156965
Nath, D. C. D., and Sahajwalla, V. (2011a). Growth mechanism of carbon nanotubes produced by pyrolysis of a composite film of poly (vinyl alcohol) and fly ash. Appl. Phys. A Mater. Sci. Process. 104, 539–544. doi: 10.1007/s00339-011-6405-1
Nath, D. C. D., and Sahajwalla, V. (2011b). Application of fly ash as a catalyst for synthesis of carbon nanotube ribbons. J. Hazard. Mater. 192, 691–697. doi: 10.1016/j.jhazmat.2011.05.072
Nath, D. C. D., and Sahajwalla, V. (2012). Analysis of carbon nanotubes produced by pyrolysis of composite film of poly (Vinyl Alcohol) and modified fly ash. Mater. Sci. Appl. 3, 103–109. doi: 10.4236/msa.2012.32016
Nayak, P., Kumar, S., Sinha, I., and Singh, K. K. (2019). ZnO/CuO nanocomposites from recycled printed circuit board: preparation and photocatalytic properties. Environ. Sci. Pollut. Res. 26, 16279–16288. doi: 10.1007/s11356-019-04986-6
Novoselov, K. S., Fal'Ko, V. I., Colombo, L., Gellert, P. R., Schwab, M. G., and Kim, K. (2012). A roadmap for graphene. Nature 490, 192–200. doi: 10.1038/nature11458
Parasuram, B., Sundaram, S., Sathiskumar, C., and Karthikeyan, S. (2018). Synthesis of multi-walled carbon nanotubes using tire pyrolysis oil as a carbon precursor by spray pyrolysis method. Inorg. Nano-Metal Chem. 48, 103–106. doi: 10.1080/24701556.2017.1357578
Park, E. K., Jung, B. B., Choi, W. Z., and Oh, S. K. (2017). A basic study on sorting of black plastics of waste electrical and electronic equipment (WEEE). J. Korean Inst. Resour. Recycl. 26, 69–77. doi: 10.7844/kirr.2017.26.1.69
Pereira de Abreu, D. A., Cruz, J. M., and Losada, P. P. (2012). Active and intelligent packaging for the food industry. Food Rev. Int. 28, 146–187. doi: 10.1080/87559129.2011.595022
Peres, E. C., Slaviero, J. C., Cunha, A. M., Hosseini-Bandegharaei, A., and Dotto, G. L. (2018). Microwave synthesis of silica nanoparticles and its application for methylene blue adsorption. J. Environ. Chem. Eng. 6, 649–659. doi: 10.1016/j.jece.2017.12.062
Peters, R. J. B., Bouwmeester, H., Gottardo, S., Amenta, V., Arena, M., Brandhoff, P., et al. (2016). Nanomaterials for products and application in agriculture, feed and food. Trends Food Sci. Technol. 54, 155–164. doi: 10.1016/j.tifs.2016.06.008
Pol, V. G., and Thiyagarajan, P. (2010). Remediating plastic waste into carbon nanotubes. J. Environ. Monit. 12, 455–459. doi: 10.1039/B914648B
Quan, C., Li, A., and Gao, N. (2010). Synthesis of carbon nanotubes and porous carbons from printed circuit board waste pyrolysis oil. J. Hazard. Mater. 179, 911–917. doi: 10.1016/j.jhazmat.2010.03.092
Quesnel, E., Roux, F., Emieux, F., Faucherand, P., Kymakis, E., Volonakis, G., et al. (2015). Graphene-based technologies for energy applications, challenges and perspectives. 2D Mater. 2:030204. doi: 10.1088/2053-1583/2/3/030204
Rahimi, A. R., and Garciá, J. M. (2017). Chemical recycling of waste plastics for new materials production. Nat. Rev. Chem. 1, 1–11. doi: 10.1038/s41570-017-0046
Rao, C. N. R., Sood, A. K., Subrahmanyam, K. S., and Govindaraj, A. (2009). Graphene: the new two-dimensional nanomaterial. Angew. Chemie Int. Ed. 48, 7752–7777. doi: 10.1002/anie.200901678
Ritchie, H., and Roser, M. (2020). Plastic Pollution. Available online at: https://ourworldindata.org/plastic-pollution
Rovani, S., Santos, J. J., Corio, P., and Fungaro, D. A. (2018). Highly pure silica nanoparticles with high adsorption capacity obtained from sugarcane waste ash. ACS Omega 3, 2618–2627. doi: 10.1021/acsomega.8b00092
Roy, I., Sarkar, G., Mondal, S., Rana, D., Bhattacharyya, A., Saha, N. R., et al. (2016). Synthesis and characterization of graphene from waste dry cell battery for electronic applications. RSC Adv. 6, 10557–10564. doi: 10.1039/C5RA21112C
Ruan, G., Sun, Z., Peng, Z., and Tour, J. M. (2011). Growth of graphene from food, insects, and waste. ACS Nano 5, 7601–7607. doi: 10.1021/nn202625c
Samaddar, P., Ok, Y. S., Kim, K. H., Kwon, E. E., and Tsang, D. C. W. (2018). Synthesis of nanomaterials from various wastes and their new age applications. J. Clean. Prod. 197, 1190–1209. doi: 10.1016/j.jclepro.2018.06.262
Sanchez, V. C., Pietruska, J. R., Miselis, N. R., Hurt, R. H., and Kane, A. B. (2009). Biopersistence and potential adverse health impacts of fibrous nanomaterials: what have we learned from asbestos? Wiley Interdiscip. Rev. Nanomed. Nanobiotechnol. 1, 511–529. doi: 10.1002/wnan.41
Sardot, T., Smith, G., and McDonald, A. (2012). Valorizing mixed plastic wastes from cardboard recycling by amendment with wood, cement and ash. J. Reinf. Plast. Compos. 31, 1488–1497. doi: 10.1177/0731684412459984
Sathiskumar, C., and Karthikeyan, S. (2019). Recycling of waste tires and its energy storage application of by-products –a review. Sustain. Mater. Technol. 22. doi: 10.1016/j.susmat.2019.e00125
Shadjou, N., and Hasanzadeh, M. (2016). Graphene and its nanostructure derivatives for use in bone tissue engineering: recent advances. J. Biomed. Mater. Res. Part A 104, 1250–1275. doi: 10.1002/jbm.a.35645
Sharma, S., Kalita, G., Hirano, R., Shinde, S. M., Papon, R., Ohtani, H., et al. (2014). Synthesis of graphene crystals from solid waste plastic by chemical vapor deposition. Carbon N. Y. 72, 66–73. doi: 10.1016/j.carbon.2014.01.051
Shokri, A., Pahlevani, F., Levick, K., Cole, I., and Sahajwalla, V. (2017). Synthesis of copper-tin nanoparticles from old computer printed circuit boards. J. Clean. Prod. 142, 2586–2592. doi: 10.1016/j.jclepro.2016.11.017
Singh, J., and Lee, B. K. (2016). Recovery of precious metals from low-grade automobile shredder residue: a novel approach for the recovery of nanozero-valent copper particles. Waste Manag. 48, 353–365. doi: 10.1016/j.wasman.2015.10.019
Singh, N., Hui, D., Singh, R., Ahuja, I. P. S., Feo, L., and Fraternali, F. (2017). Recycling of plastic solid waste: a state of art review and future applications. Compos. Part B Eng. 115, 409–422. doi: 10.1016/j.compositesb.2016.09.013
Smith, K. S., Hageman, P. L., Plumlee, G. S., Budahn, J. R., and Bleiwas, D. I. (2015). “Potential metal recovery from waste streams,” in International Applied Geochemistry Symposium (IAGS) (Tucson, AZ).
Smolkova, B., El Yamani, N., Collins, A. R., Gutleb, A. C., and Dusinska, M. (2015). Nanoparticles in food: epigenetic changes induced by nanomaterials and possible impact on health. Food Chem. Toxicol. 77, 64–73. doi: 10.1016/j.fct.2014.12.015
Sofi, M., Sabri, Y., Zhou, Z., and Mendis, P. (2019). Transforming municipal solid waste into construction materials. Sustain 11:2611. doi: 10.3390/su11092661
Soldano, C., Mahmood, A., and Dujardin, E. (2010). Production, properties and potential of graphene. Carbon N. Y. 48, 2127–2150. doi: 10.1016/j.carbon.2010.01.058
Sonawane, J. M., Yadav, A., Ghosh, P. C., and Adeloju, S. B. (2017). Recent advances in the development and utilization of modern anode materials for high performance microbial fuel cells. Biosens. Bioelectron. 90, 558–576. doi: 10.1016/j.bios.2016.10.014
Søndergaard, R. R., Zimmermann, Y. S., Espinosa, N., Lenz, M., and Krebs, F. (2016). Incineration of organic solar cells: efficient end of life management by quantitative silver recovery. Energy Environ. Sci. 9, 857–861. doi: 10.1039/C6EE00021E
Song, R., and Ji, Q. (2011). Synthesis of carbon nanotubes from polypropylene in the presence of Ni/Mo/MgO catalysts via combustion. Chem. Lett. 40, 1110–1112. doi: 10.1246/cl.2011.1110
Stevens, G. C., and Goosey, M. (2009). “Materials used in manufacturing electrical and electronic products,” in Electronic Waste Management (Cambridge, UK: RSC Publishing), 40–74. doi: 10.1039/9781847559197-00040
Strudwick, A. J., Weber, N. E., Schwab, M. G., Kettner, M., Weitz, R. T., Wünsch, J. R., et al. (2015). Chemical vapor deposition of high quality graphene films from carbon dioxide atmospheres. ACS Nano 9, 31–42. doi: 10.1021/nn504822m
Sukanan, D. (2020). Waste Imports Are Flooding Asian Countries Like Thailand. Available at: https://www.sustainability-times.com/environmental-protection/asian-countries-like-thailand-are-flooded-by-waste-imports/ (accessed May 10, 2020).
Surwade, S. P., Smirnov, S. N., Vlassiouk, I. V., Unocic, R. R., Veith, G. M., Dai, S., et al. (2015). Water desalination using nanoporous single-layer graphene. Nat. Nanotechnol. 10, 459–464. doi: 10.1038/nnano.2015.37
Tang, T., Chen, X., Meng, X., Chen, H., and Ding, Y. (2005). Synthesis of multiwalled carbon nanotubes by catalytic combustion of polypropylene. Angew. Chemie Int. Ed. 44, 1517–1520. doi: 10.1002/anie.200461506
Tang, W., Li, Q., Gao, S., and Shang, J. K. (2011). Arsenic (III,V) removal from aqueous solution by ultrafine α-Fe2O3 nanoparticles synthesized from solvent thermal method. J. Hazard. Mater. 192, 131–138. doi: 10.1016/j.jhazmat.2011.04.111
Tansel, B. (2017). From electronic consumer products to e-wastes: global outlook, waste quantities, recycling challenges. Environ. Int. 98, 35–45. doi: 10.1016/j.envint.2016.10.002
Tatariants, M., Yousef, S., Sakalauskaite, S., Daugelavičius, R., Denafas, G., and Bendikiene, R. (2018). Antimicrobial copper nanoparticles synthesized from waste printed circuit boards using advanced chemical technology. Waste Manag. 78, 521–531. doi: 10.1016/j.wasman.2018.06.016
Thai, Q. B., Le, D. K., Luu, T. P., Hoang, N., Nguyen, D., and Duong, H. M. (2019). Aerogels from wastes and their applications. JOJ Mater. Sci. 5:555663. doi: 10.19080/JOJMS.2019.05.555663
Tran, D. T., Joubert, A., Venditti, D., Durecu, S., Meunier, T., Le Bihan, O., et al. (2017). Characterization of polymer waste containing nano-fillers prior its end-of-life treatment. Waste Biomass Valorization 8, 2463–2471. doi: 10.1007/s12649-016-9757-0
Tripathi, P. K., Durbach, S., and Coville, N. J. (2017). Synthesis of multi-walled carbon nanotubes from plastic waste using a stainless-steel CVD reactor as catalyst. Nanomater 7:284. doi: 10.3390/nano7100284
Turcheniuk, K., and Mochalin, V. N. (2017). Biomedical applications of nanodiamond (Review). Nanotechnology 28, 252001. doi: 10.1088/1361-6528/aa6ae4
Turgut, P., and Yesilata, B. (2008). Physico-mechanical and thermal performances of newly developed rubber-added bricks. Energy Build. 40, 679–688. doi: 10.1016/j.enbuild.2007.05.002
UNEP (2020). Solid Waste Management | UNEP - UN Environment Programme. Available online at: https://www.unenvironment.org/explore-topics/resource-efficiency/what-we-do/cities/solid-waste-management (accessed May 10, 2020).
Urbina, J., Patil, A., Fujishima, K., Paulino-Lima, G., Saltikov, C., and Rothschild, L. J. (2019). A new approach to biomining: bioengineering surfaces for metal recovery from aqueous solutions. Sci. Reports (Nature Publ. Group) 9, 16422. doi: 10.1038/s41598-019-52778-2
Valko, M., Morris, H., and Cronin, M. T. D. (2005). Metals, Toxicity and Oxidative Stress. doi: 10.2174/0929867053764635
Verma, M. L., Puri, M., and Barrow, C. J. (2016). Recent trends in nanomaterials immobilised enzymes for biofuel production. Crit. Rev. Biotechnol. 36, 108–119. doi: 10.3109/07388551.2014.928811
Vermisoglou, E. C., Giannouri, M., Todorova, N., Giannakopoulou, T., Lekakou, C., and Trapalis, C. (2016). Recycling of typical supercapacitor materials. Waste Manag. Res. 34, 337–344. doi: 10.1177/0734242X15625373
Wang, H., Gao, B., Fang, J., Ok, Y. S., Xue, Y., Yang, K., et al. (2018). Engineered biochar derived from eggshell-treated biomass for removal of aqueous lead. Ecol. Eng. 121, 124–129. doi: 10.1016/j.ecoleng.2017.06.029
Westerhoff, P. K., Kiser, A., and Hristovski, K. (2013). Nanomaterial removal and transformation during biological wastewater treatment. Environ. Eng. Sci. 30, 109–117. doi: 10.1089/ees.2012.0340
Wibowo, S., Deng, H., and Zhang, X. (2014). “Evaluating the performance of e-waste recycling programs using fuzzy multiattribute group decision making model,” in Proceedings of the 2014 9th IEEE Conference on Industrial Electronics and Applications, ICIEA 2014 (Institute of Electrical and Electronics Engineers Inc.), 1989–1994. doi: 10.1109/ICIEA.2014.6931495
Wu, C., Wang, Z., Wang, L., Williams, P. T., and Huang, J. (2012). Sustainable processing of waste plastics to produce high yield hydrogen-rich synthesis gas and high quality carbon nanotubes. RSC Adv. 2, 4045–4047. doi: 10.1039/c2ra20261a
Wu, Z. J., Huang, W., Cui, K. K., Gao, Z. F., and Wang, P. (2014). Sustainable synthesis of metals-doped ZnO nanoparticles from zinc-bearing dust for photodegradation of phenol. J. Hazard. Mater. 278, 91–99. doi: 10.1016/j.jhazmat.2014.06.001
Xiang, X., Xia, F., Zhan, L., and Xie, B. (2015). Preparation of zinc nano structured particles from spent zinc manganese batteries by vacuum separation and inert gas condensation. Sep. Purif. Technol. 142, 227–233. doi: 10.1016/j.seppur.2015.01.014
Xiu, F. R., and Zhang, F. S. (2009). Preparation of nano-Cu2O/TiO2 photocatalyst from waste printed circuit boards by electrokinetic process. J. Hazard. Mater. 172, 1458–1463. doi: 10.1016/j.jhazmat.2009.08.012
Xiu, F. R., and Zhang, F. S. (2012). Size-controlled preparation of Cu2O nanoparticles from waste printed circuit boards by supercritical water combined with electrokinetic process. J. Hazard. Mater. 233–234, 200–206. doi: 10.1016/j.jhazmat.2012.07.019
Xu, Y., Li, J., and Liu, L. (2016). Current status and future perspective of recycling copper by hydrometallurgy from waste printed circuit boards. Procedia Environ. Sci. 31, 162–170. doi: 10.1016/j.proenv.2016.02.022
Yang, K., Feng, L., and Liu, Z. (2016a). Stimuli responsive drug delivery systems based on nano-graphene for cancer therapy. Adv. Drug Deliv. Rev. 105, 228–241. doi: 10.1016/j.addr.2016.05.015
Yang, R. X., Chuang, K. H., and Wey, M. Y. (2016b). Carbon nanotube and hydrogen production from waste plastic gasification over Ni/Al-SBA-15 catalysts: effect of aluminum content. RSC Adv. 6, 40731–40740. doi: 10.1039/C6RA04546D
Yang, Z., Tian, J., Yin, Z., Cui, C., Qian, W., and Wei, F. (2019). Carbon nanotube- and graphene-based nanomaterials and applications in high-voltage supercapacitor: a review. Carbon N. Y. 141, 467–480. doi: 10.1016/j.carbon.2018.10.010
Yousef, S., Tatariants, M., Makarevičius, V., Lukošiute, S. I., Bendikiene, R., and Denafas, G. (2018). A strategy for synthesis of copper nanoparticles from recovered metal of waste printed circuit boards. J. Clean. Prod. 185, 653–664. doi: 10.1016/j.jclepro.2018.03.036
Zeng, W., and Bai, H. (2016). High-performance CO2 capture on amine-functionalized hierarchically porous silica nanoparticles prepared by a simple template-free method. Adsorption 22, 117–127. doi: 10.1007/s10450-015-9698-0
Zeng, X., Yang, C., Chiang, J. F., and Li, J. (2017). Innovating e-waste management: from macroscopic to microscopic scales. Sci. Total Environ. 575, 1–5. doi: 10.1016/j.scitotenv.2016.09.078
Zhan, L., Xiang, X., Xie, B., and Sun, J. (2016). A novel method of preparing highly dispersed spherical lead nanoparticles from solders of waste printed circuit boards. Chem. Eng. J. 303, 261–267. doi: 10.1016/j.cej.2016.06.002
Zhang, H., Bussini, D., Hortal, M., Elegir, G., Mendes, J., and Jordá Beneyto, M. (2016). PLA coated paper containing active inorganic nanoparticles: material characterization and fate of nanoparticles in the paper recycling process. Waste Manag. 52, 339–345. doi: 10.1016/j.wasman.2016.03.045
Zhang, H., Xia, B., Wang, P., Wang, Y., Li, Z., Wang, Y., et al. (2020). From waste to waste treatment: mesoporous magnetic NiFe2O4/ZnCuCr-layered double hydroxide composite for wastewater treatment. J. Alloys Compd. 819, 153053. doi: 10.1016/j.jallcom.2019.153053
Zhang, J., Li, J., Cao, J., and Qian, Y. (2008). Synthesis and characterization of larger diameter carbon nanotubes from catalytic pyrolysis of polypropylene. Mater. Lett. 62, 1839–1842. doi: 10.1016/j.matlet.2007.10.015
Zhao, Y. M., Duan, C. L., Wu, L. L., Zhang, H. J., He, J. F., and He, Y. Q. (2012). The separation mechanism and application of a tapered diameter separation bed. Int. J. Environ. Sci. Technol. 9, 719–728. doi: 10.1007/s13762-012-0105-z
Zheng, Y., Yanful, E. K., and Bassi, A. S. (2005). A review of plastic waste biodegradation. Crit. Rev. Biotechnol. 25, 243–250. doi: 10.1080/07388550500346359
Zhou, H., Su, M., Lee, P. H., and Shih, K. (2017). Synthesis of submicron lead oxide particles from the simulated spent lead paste for battery anodes. J. Alloys Compd. 690, 101–107. doi: 10.1016/j.jallcom.2016.08.094
Zhuo, C., Brendan, H., Henning, R., and Yiannis, L. (2010). Synthesis of carbon nanotubes by sequential pyrolysis and combustion of polyethylene. Carbon 48, 4024–4034. doi: 10.1016/j.carbon.2010.07.007
Keywords: carbon nanoparticles, metal nanoparticles, e-waste, plastic waste, industrial waste, nanoparticle-enabled technologies
Citation: Abdelbasir SM, McCourt KM, Lee CM and Vanegas DC (2020) Waste-Derived Nanoparticles: Synthesis Approaches, Environmental Applications, and Sustainability Considerations. Front. Chem. 8:782. doi: 10.3389/fchem.2020.00782
Received: 11 May 2020; Accepted: 27 July 2020;
Published: 31 August 2020.
Edited by:
Francesca Deganello, Italian National Research Council, ItalyReviewed by:
Sergio Gonzalez-Cortes, University of Oxford, United KingdomIl Je Yu, Independent Researcher, Icheon, South Korea
Copyright © 2020 Abdelbasir, McCourt, Lee and Vanegas. This is an open-access article distributed under the terms of the Creative Commons Attribution License (CC BY). The use, distribution or reproduction in other forums is permitted, provided the original author(s) and the copyright owner(s) are credited and that the original publication in this journal is cited, in accordance with accepted academic practice. No use, distribution or reproduction is permitted which does not comply with these terms.
*Correspondence: Diana C. Vanegas, ZHZhbmVnYUBjbGVtc29uLmVkdQ==