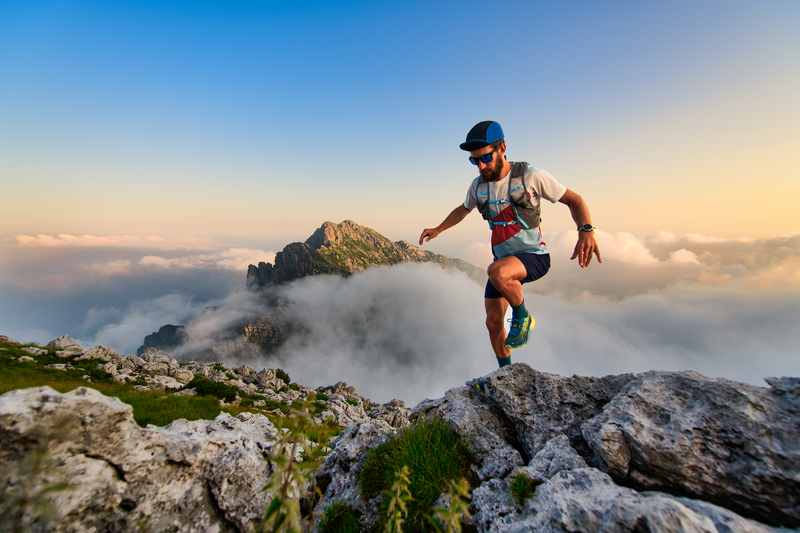
95% of researchers rate our articles as excellent or good
Learn more about the work of our research integrity team to safeguard the quality of each article we publish.
Find out more
MINI REVIEW article
Front. Chem. , 14 July 2020
Sec. Nanoscience
Volume 8 - 2020 | https://doi.org/10.3389/fchem.2020.00537
This article is part of the Research Topic Functionalized Inorganic Semiconductor Nanomaterials: Characterization, Properties, and Applications View all 18 articles
In this mini-review, a comprehensive discussion on the state of the art of hybrid organic–inorganic mixed ionic–electronic conductors (hOI-MIECs) is given, focusing on conducting polymer nanocomposites comprising inorganic nanoparticles ranging from ceramic-in-polymer to polymer-in-ceramic concentration regimes. First, a brief discussion on fundamental aspects of mixed ionic–electronic transport phenomena considering the charge carrier transport at bulk regions together with the effect of the organic–inorganic interphase of hybrid nanocomposites is presented. We also make a recount of updated instrumentation techniques to characterize structure, microstructure, chemical composition, and mixed ionic–electronic transport with special focus on those relevant for hOI-MIECs. Raman imaging and impedance spectroscopy instrumentation techniques are particularly discussed as relatively simple and versatile tools to study the charge carrier localization and transport at different regions of hOI-MIECs including both bulk and interphase regions to shed some light on the mixed ionic–electronic transport mechanism. In addition, we will also refer to different device assembly configurations and in situ/operando measurements experiments to analyze mixed ionic–electronic conduction phenomena for different specific applications. Finally, we will also review the broad range of promising applications of hOI-MIECs, mainly in the field of energy storage and conversion, but also in the emerging field of electronics and bioelectronics.
In the last decades, mixed ionic–electronic conductors (MIECs) have been widely studied for energy storage and energy conversion materials, separation membranes, and catalysts (Shao and Haile, 2004; Maier, 2005; Wachsman and Lee, 2011; Aoki et al., 2014). Both ionic (σi) or electronic (σe) conduction obey separately and analogously to the following equation:
where q is the charge, N is the number, and μ is the mobility of the charge carrier, the latter being proportional to diffusivity (D). In the particular case of inorganic MIECs, some well-known examples are semiconducting compounds such as Ag2X (with X = S, Se, or Te) as mixed silver ion (Ag+) and electronic conducting materials (Yokota, 1961; Miyatani, 1973; Riess, 2003) and A-doped MO2−δ (typically M = Ce or Zr, and A being different dopants) as mixed oxygen ion (O2−) and electronic transport materials (Goodenough, 2000; Balaguer et al., 2011; Lin et al., 2015). However, one of the most relevant inorganic MIEC materials gaining special attention in the recent years are AXM2O4 (with M = Ni, Co, and/or Mn and A = Li or Na) due to their excellent performance, particularly as cathode materials for lithium (Li+) and sodium (Na+) ion batteries (Doeff et al., 1993; Barker et al., 1996; Saïdi et al., 1996; Thackeray, 1997; Dokko et al., 2001; Lu and Dahn, 2001; Cao and Prakash, 2002; Levasseur et al., 2002; Sauvage et al., 2007; Berthelot et al., 2010; Tevar and Whitacre, 2010). For instance, typical electronic conductivities (σe) and lithium-ion diffusivities (Di) for LiXM2O4 cathode materials are σe ~ 10−6-10−1 S cm−1 and Di ~ 10−11-10−8 cm2s−1, respectively, depending strongly on the transition metal (M), lithiation degree (x), and crystallinity (Park et al., 2010). In the particular case of semiconducting inorganic nanomaterials, both ionic and electronic transport present lower charge carrier resistance at the crystalline bulk regions but are drastically compromised by the poor charge carrier conducting nature of grain boundaries (Park et al., 2010). In the last decades, the addition of conducting coating materials and secondary phases such as mixed ionic–electronic conducting organic materials (e.g., conducting polymers), working as linkers between inorganic nanomaterials, has attracted a lot of attention (Judeinstein and Sanchez, 1996; Gómez-Romero and Lira-Cantú, 1997; Guizard et al., 2001; Le Bideau et al., 2011). It is well-accepted that electronic conducting organic polymers, usually called conjugated polymers, are semiconductors in nature and that the most popular cases such as poly(pyrrole) (Ppy) (Della Santa et al., 1997), poly(aniline) (PANI) (Zhang K. et al., 2012a; Chatterjee et al., 2013; Zhang Q. et al., 2013a; Roussel et al., 2015), poly(ethylenedioxythiophene) (PEDOT) (Crispin et al., 2006; Udo et al., 2009; Takano et al., 2012; Kim et al., 2013; Mengistie et al., 2013, 2015; Lee et al., 2014; Kumar et al., 2016; Zia Ullah et al., 2016), and poly(3-hexylthiophene) (P3HT) (Zhang Q. et al., 2012; Pingel and Neher, 2013; Glaudell et al., 2015; Jacobs et al., 2016; Qu et al., 2016; Jung et al., 2017; Wang W. et al., 2017; Lim et al., 2018) generally exhibit an electronic donor behavior. In this case, the most common procedure to enhance the electronic conduction, where charge carriers will be mostly holes rather than electrons, is by doping these polymers with electronic acceptor species (p-type doping) such as halide and sulfonate salts, yielding a decrease in the electronic band gap and an increase of the electronic conductivity up to σe ~ 10−1-103 S cm−1 values (Della Santa et al., 1997; Crispin et al., 2006; Udo et al., 2009; Takano et al., 2012; Zhang K. et al., 2012; Zhang Q. et al., 2012, 2013; Chatterjee et al., 2013; Kim et al., 2013; Mengistie et al., 2013, 2015; Pingel and Neher, 2013; Lee et al., 2014; Glaudell et al., 2015; Roussel et al., 2015; Jacobs et al., 2016; Kumar et al., 2016; Qu et al., 2016; Zia Ullah et al., 2016; Jung et al., 2017; Wang W. et al., 2017; Lim et al., 2018). The mere presence of the dopant, typically halide, or sulfonate salts with relatively high degree of dissociation, will trigger a non-negligible ionic conduction in addition to the electronic transport (Riess, 2000). It is important to mention that there are other “non-dissociable” excellent dopants such as the case of tetracyanoquinodimethane (TCNQ) in all of its fluorinated forms, but as it does not provide highly mobile ionic carriers, it will not be considered in this review. It was long observed that protons (H+), lithium (Li+), sodium (Na+), or potassium (K+) cations yielded a considerable ionic contribution to the total mixed ionic–electronic transport of conjugated polymers (Nigrey et al., 1978; Aldebert et al., 1986; Barthet and Guglielmi, 1995; Watanabe, 1996). The voluminous dopant anions are generally more fixed to the polymer chain, allowing the electronic exchange process (doping) to take place but contributing in a lesser extent to the ionic conductivity except for a few particular cases (Cheng et al., 2005). Pursuing an increase in the ionic conduction of MIECs, blending and co-polymerization (including functionalization of side chains) of electronic conducting polymers with good ionic conducting polymers [e.g., poly(ethylene oxide) (PEO)], has shown enhancement of ionic conductivities up to σi ~ 10−5-10−4 S cm−1 (Li and Khan, 1991; Barthet et al., 1997; Ghosh and Inganäs, 2000; Zhang et al., 2002; Patel et al., 2012; Ju et al., 2014; Kang et al., 2014; Dong et al., 2019; Sengwa and Dhatarwal, 2020). Another strategy includes the simultaneous doping and blending of electronic conducting polymers with polymeric dopants, particularly observed for protons and lithium-ion charge carriers (Murthy and Manthiram, 2011; Fu and Manthiram, 2012; Liu et al., 2012). However, it is important to remark that the inclusion of electronic-insulating polymers inevitably leads to the declining of the electronic conductivity (σe ~ 10−5 S cm−1, i.e., several orders of magnitude less than the isolated conducting polymer in its doped form), and thus, electronic-conducting polymer/ionic-conducting polymer/dopant concentrations need to be rationally balanced (Li and Khan, 1991; Barthet et al., 1997; Ghosh and Inganäs, 2000; Zhang et al., 2002; Murthy and Manthiram, 2011; Fu and Manthiram, 2012; Liu et al., 2012; Patel et al., 2012; Ju et al., 2014; Kang et al., 2014; Dong et al., 2019; Sengwa and Dhatarwal, 2020). Recent comprehensive reviews discussing different types of organic MIEC classes, with particular focus on taxonomy and electronic–ionic interactions, are given by Paulsen et al. (2020), and a thorough discussion of morphologic effects on organic polymeric MIEC is given by Onorato and Luscombe (2019). On the other hand, it is well-known that the addition of semiconducting ceramic nanoparticles, even with negligible intrinsic electronic (or ionic) transport ability, can also yield an enhancement of the electronic (or ionic) conduction in conducting polymer nanocomposites. For instance, the presence of inorganic nanoparticles, particularly transition metal oxides, has yielded a notorious increment of electronic conductivity for electronic–conductor polymer nanocomposites in both ceramic-in-polymer (Mombrú et al., 2017a,b; Mombrú et al., 2019) and polymer-in-ceramic concentration regimes (Huguenin et al., 2004; Wang et al., 2010; Mombrú et al., 2017a). In analogy, the presence of inorganic nanoparticles resulted in an enhancement on the ionic conductivity for ionic conductor polymer nanocomposites (Kloster et al., 1996; Scrosati et al., 2000; Shin and Passerini, 2004). The presence of secondary phases or inorganic nanofillers induces slight structural modifications, altering the degree of order of the conducting polymer chains that could explain the enhancement of the conductivity, without considering direct mediation of charge carriers through the nanoparticle interphase. Although it is accepted that the electronic conduction in polymer nanocomposites is usually related to higher crystallinity (or higher degree of order), the enhancement of the ionic conduction is mostly associated to lower crystallinity (or lower degree of order), but the latter case is still under recent debate (Onorato and Luscombe, 2019). Furthermore, in the case of ceramic nanoparticles' interaction with conducting polymers, the presence of an interphase between both organic and inorganic materials adds a particular complexity to the system and can eventually lead to important consequences in both ionic and electronic transport properties. Leaving out drastic effects such as voids, poor contact, or the presence of decomposition phases due to eventual chemical reactions, it is extremely difficult to obtain well-defined interphases between such different materials. For instance, the presence of defects, mainly in the inorganic nanoparticle boundaries, can lead to the presence of charge localization at the interphase and the presence of different crystallographic surfaces of the inorganic nanoparticle at the interphase can exhibit different electronic interactions with the polymer phase. Up to now, to the best of our knowledge, there are only a few reviews of MIEC materials with particular focus on their applications such as energy (Sengodu and Deshmukh, 2015), bioelectronics (Han S. et al., 2019), and sensing (Inal et al., 2018), but no further insights into hOI-MIECs. In this mini-review, charge carrier localization and transport at different regions of hOI-MIECs including both bulk and interphase regions is revised, focusing on the use of some powerful and versatile instrumental techniques.
There are a lot of instrumentation techniques that can provide particularly rich information about structural features of hOI-MIECs such as Nuclear Magnetic Resonance (NMR), X-ray diffraction (XRD), and wide-/small-angle X-ray scattering (WAXS/SAXS) in both transmission or grazing incidence configurations (Sanjeeva Murthy, 2016). However, it is important to remark that X-ray scattering techniques are relatively accessible but generally give indirect information about charge carrier localization and on the other hand, although NMR could be very powerful to monitor charge carrier's location, it is particularly less versatile than other optical spectroscopies techniques. For instance, a relatively simple and powerful method to monitor not only charge localization but also drift mobility in organic MIECs is the “moving front” experiment, which is based on visible light transmission monitoring through an electrochromic film as it is dedoped due to lateral injection of H+, Na+, or K+ ions from a planar junction with an electrolyte, as shown in Figure 1A (Stavrinidou et al., 2013; Rivnay et al., 2016). Nonetheless, one of the most popular but no less powerful and versatile technique to study structural features of hOI-MIECs is vibrational spectroscopy. Raman spectroscopy is particularly interesting for inorganic materials characterization as it does not exclude highly amorphous systems in comparison with XRD and provides accessibility to vibrational modes with lower wavenumbers (typically νmin ~ 80–100 cm−1) in comparison to infrared spectroscopy (typically νmin ~ 200–400 cm−1). Raman spectroscopy also has the remarkable advantage of needing little sample preparation, allowing the study of materials in its native conditions, as well as permitting collection of in situ and in operando measurements. For instance, in situ/operando Raman spectroscopy has allowed the study of the state of charge of (Li, Na, K)XM2O4 electrodes by monitoring the broadening and shifting of Raman peaks when lowering Li, Na, or K content from nominal X = 1 (full charged cathode), particularly associated to the loss of ions from the interlayer of the MO2 layered structure (Dokko et al., 2003; Nanda et al., 2011; Nishi et al., 2013; Chen et al., 2015; Flores et al., 2018). An example on the use of Raman imaging to monitor the state of charge for a Li1−x(NiyCozAl1−y−z)O2 cathode is shown and described briefly in Figure 1B (Nanda et al., 2011). In addition, the use of micro-Raman imaging technique is highly powerful to study simultaneously both compositional and microstructural features, especially for hybrid inorganic–organic materials, as the characteristic Raman signals for inorganic and organic compounds generally lie well-separated at lower (ν < 800 cm−1) and higher (ν > 800 cm−1) wavenumbers, respectively (Romero et al., 2016; Mombrú et al., 2017a,b,c; Pignanelli et al., 2018, 2019a,b). Furthermore, although Raman spectroscopy is quite sensitive to diluted effects such as doping processes of inorganic materials, it is on the other hand, extremely sensitive to doping effects of organic materials such as conducting polymers (Furukawa, 1996). Briefly, the doping process of conducting polymers yields to drastic modifications of the Raman signature in relation to the charge carrier formation, typically in the form of positive polarons (–C+-C•-) or bipolarons (–C+-C+-), particularly altering both Raman frequency and activity of vibrational modes associated to carbon-to-carbon (C=C) molecular bonds in conjugated polymers (Furukawa, 1996; Kumar et al., 2012; Yamamoto and Furukawa, 2015; Francis et al., 2017; Mombrú et al., 2018; Nightingale et al., 2018). For instance, micro-Raman imaging has evidenced the presence of these types of charge carriers particularly localized near the interphase with inorganic nanoparticles; [e.g., MX2 with M being different transition metals and X = O (for oxides) or S (for sulfides) (Mombrú et al., 2017a,b,c; Mombrú et al., 2019)]. The increment of conducting polymer electronic charge carriers near the interphase could be discussed in view of at least two eventual scenarios: (one or passive) the dopant stabilizes at the interphase due to strong polar or coulombic interactions with nanoparticles surface, or/and (two or active) the nanoparticles are also good electronic acceptors, producing in both cases an enhancement on the doping of nearby polymer chains, as schematized in Figure 1C (upper panel). On the other hand, micro-Raman imaging has also been useful to evidence the enhancement of ionic-pair dissociation occurring near the interphase with inorganic nanoparticles, in agreement with the increment of ionic conductivity (Romero et al., 2016; Pignanelli et al., 2018, Pignanelli et al., 2019a). Analogously, two different scenarios could be discussed for ionic charge carriers: (one or passive) the counter-ion (in analogy to the dopant anion) stabilizes at the interphase due to strong polar or coulombic interactions with nanoparticles surface yielding an enhancement on the ionic-pair dissociation, or/and (two or active) the nanoparticles may also possess mobile ionic carriers at the surface (e.g., active filler) that can be injected into the polymer, as schematized in Figure 1C (lower panel). Whatever the case, the previous micro-Raman imaging studies revealed that the interphase of organic–inorganic nanocomposites, to a greater or lesser extent, always play an important role in the charge carrier transport mechanism.
Figure 1. (A) Schematization indicating the charge distribution around the dedoping front (upper panel) and evolution of dedoping front where ΔT is the change of transmitted light intensity with respect to the zero bias state during the injection of potassium cations for PEDOT:PSS film (lower panel) (Stavrinidou et al., 2013). This figure was used and adapted/altered minimally with permission from John Wiley and Sons. (B) Optical image (upper panel) and Raman imaging (lower panel) providing a semi-quantitative measure of the Li1−x(NiyCozAl1−y−z)O2 (NCA) cathode state of charge (SOC) where the dark region is associated to carbon-rich zone and the colored region is associated to the NCA-rich zone ranging from blue (lower SOC) to red (higher SOC) (Nanda et al., 2011). This figure was used and adapted/altered minimally with permission from John Wiley and Sons. (C) Raman imaging and schematization of charge carrier localization near hybrid organic–inorganic interphases for electronic conducting polymer nanocomposite (sulfonic acid-doped polyaniline with embedded TiO2 nanoparticles; Mombrú et al., 2017a) (upper panel) and ionic conducting polymer nanocomposite (lithium nitrate solid polymethylmethacrylate electrolyte with embedded Li0.3La0.7TiO3 nanoparticles; Romero et al., 2016) (lower panel). References for schematization are as follows: organic polymer (blue), inorganic nanoparticles (red), dopant cation (+, in pink), dopant anion (–, in purple), and electronic charge carriers (+, in dark blue). Micro-Raman images and spectra are portions of figures adapted/altered minimally with permission from Elsevier.
There are several electrochemical methodologies to study the charge carrier conduction in MIECs, but one of the most powerful techniques to access both electronic and ionic transport simultaneously is impedance spectroscopy (Jamnik and Maier, 1999; Vorotyntsev et al., 1999; Huggins, 2002; Atkinson et al., 2004; Lee et al., 2009). Briefly, the impedance response as a function of the frequency (typically 10−3-106 Hz) of an oscillating voltage (typically 10–100 mV amplitude) can provide information about different charge carriers with different relaxation times (τ) depending on their q/m ratio; [i.e., the higher the q/m ratio, the lower τ and the higher associated frequencies (f = 2π/τ)]. In this case, the Nyquist representation of impedance (imaginary impedance vs. real impedance, –Z″ vs. Z′) for a single electronic semiconductor in a continuous medium will show a single semicircle arc. The semicircle arc associated to the electronic carrier transport can be typically modeled using the parallel combination of a resistor (Re) and a capacitor (Ce). In analogy, but with probably higher associated τ (lower f), a single ionic conductor in a continuous medium will also show a similar single semicircle arc associated to the ionic carrier transport that can also be modeled using the parallel combination of a resistor (Ri) and a capacitor (Ci), whose associated charge carrier pathway is represented with a straight line in Figure 2A. If an additional pathway is mediating the electronic (or ionic) transport (e.g., the presence of grain boundaries or depletion regions in less crystalline solids), a second (or ) parallel combination connected in series with the previous one is usually necessary to fit the total impedance response, whose associated charge carrier pathway is represented with a zig-zag line in Figure 2A. For simplicity, from now on, we will only consider the charge carrier transport of ionic and electronic conductor samples assembled in a symmetric cell configuration using ideal metallic ion-blocking electrodes. This means that only electronic carriers will be short-circuited and ionic species will be blocked at the interphase with the ion-blocking metallic electrodes but the opposite will apply in the case of using electronic-blocking electrodes. In the case of using metallic ion-blocking electrodes, in addition to the semicircle arc observed at higher frequencies, the Nyquist plots of single ionic conductors will also show an additional capacitive tail at low frequencies (Cint), which is associated to the polarization of blocked ions at the sample/electrode interphase, as shown in Figure 2A. If now we consider the simplest case of a MIEC material, the bi-continuous ionic and electronic channels can be strategically represented by the parallel combination of ionic and electronic resistances (Ri and Re, respectively) together with a global geometrical capacitance (Cg), with the associated pathway represented by a straight line in Figure 2B. It is important to remark that the Cint element only appears connected in series with the ionic resistance as we are working with ideal ion-blocking electrodes, but the opposite will occur (i.e., an analogous Cint element will only appear connected in series with the electronic resistance) if we are working with electronic-blocking electrodes. The origin of this circuit model simplification is described thoroughly by Jamnik and Maier and is only applicable for macroscopically thick samples considering ideal selectively ion-blocking electrodes and chemical capacitance much larger than the interfacial capacitance of the blocked carriers (Jamnik and Maier, 1999; Lee et al., 2009). In the case that any of the electronic or ionic transport is mediated by the presence of a secondary pathway in a MIEC, generally associated to grain boundaries or depleted regions, as we discuss before, a second (or ) parallel combination connected in series with Re (or Ri), respectively, could be useful to fit the total impedance response, with associated pathway represented by a zig-zag line in Figure 2B (Huggins, 2002). In the recent literature, both the inclusion and exclusion of this second (or ) parallel combination in biphasic polymeric MIECs have been observed, depending mainly on the electronic- and ionic-conducting phase concentration or microstructural differences (Patel et al., 2012; Renna et al., 2017). In the particular case of hOI-MIECs, the second contribution (and probably a third contribution) to ionic or electronic transport could be present due to the mere existence of the organic–inorganic interphase, as shown in Figure 2C. However, even for a simplified experiment configuration, (e.g., using symmetric ion-blocking electrodes), it is important to rationalize the number of elements in a given circuit model to avoid over-parametrization. For instance, in the extreme case of hOI-MIECs based on a continuous organic semiconductor, [e.g., conducting polymer with diluted inorganic nanoparticle additives (ceramic-in-polymer)], both electronic and ionic carriers will be mainly transported through the organic matrix. For instance, and elements could be eventually excluded from the circuit model in the presence of homogeneous (full crystalline or amorphous) polymeric phase. However, in consonance with the non-homogeneous localization of charge carriers discussed in the previous section, the presence of an organic–inorganic interphase can eventually activate another electronic or/and ionic pathway mediated through the interphase that could be passive or active (Irvine et al., 1990). For instance, solid polymer electrolytes with active inorganic nanofillers are the typical case of organic–inorganic interphase-mediated ionic transport (Zheng et al., 2016; Yang et al., 2017; Pignanelli et al., 2019a), and a similar behavior will be observed for the electronic counterpart, if there are electronic interactions at the organic–inorganic interphase (Chen et al., 2010; Nowy et al., 2010; Cai et al., 2012; Mombrú et al., 2017b). This effect, whose associated charge carrier pathway is represented by a curved line in Figure 2C, can also be eventually modeled with (or ) elements connected in series with the electronic (or ionic) part of the mixed ionic–electronic circuit, in analogy to (or ), respectively. However, as mentioned earlier in the previous section, even when the inorganic nanoparticles are passive or non-interacting in nature with charge carriers, the concentration of both electronic or ionic charge carriers at the vicinities of the organic–inorganic interphase could also be activating a second pathway to the charge carrier transport. Nonetheless, in the case of passive interphases, this effect could be rather weak and both charge carrier transport pathways are expected to be mainly through the organic phase without interphase mediation; thus, only a global contribution to the charge carrier transport is usually observed and additional (or ) elements are not necessary to fit the global impedance response. In the other extreme case, [i.e., hOI-MIECs based on inorganic semiconductor nanoparticles with diluted organic polymeric additives (polymer-in-ceramic)], both electronic and ionic carriers are mainly transported through the inorganic matrix. In this case, due to the inevitable presence of grain boundaries in inorganic semiconductor nanoparticles, (or ) elements should always be considered, as this contribution practically governs the global electronic (or ionic) transport. In this case, the polymeric additions usually act as fillers of empty spaces between nanoparticles, resulting in an enhancement of the electronic (or ionic) conductivity, and this is usually evaluated directly on (or ) elements. However, in the case of simultaneous presence of particle-to-particle and particle–polymer–particle interphases, there will be at least two different pathways to electronic (or ionic) transport and additional (or ) elements could be necessary to fit the polymer-mediated transport contribution, as depicted in Figure 2C.
Figure 2. Circuit model schematization for (A) separated electronic and ionic transport in a single phase, (B) mixed ionic–electronic transport in a single phase, and (C) mixed ionic–electronic transport in hOI-MIECs ranging from ceramic-in-polymer (upper panel) to polymer-in-ceramic (lower panel). Electronic and ionic hypothetical pathways are shown with dark blue and pink arrows. The zigzag part of the arrows indicates the presence of eventual grain boundaries or depleted regions [with associated ionic (i′) or electronic (e′) contributions] and the curved part of the arrows indicates the presence of eventual transport pathway mediated through organic–inorganic interfacial regions [with associated ionic (i″) and electronic (e″) contributions].
The successful synergistic properties between organic and inorganic MIECs have yielded excellent performances, especially in the field of energy storage and particularly for lithium- and sodium-ion battery electrode materials (Sengodu and Deshmukh, 2015). In this sense, active cathode or anode materials embedded in polymeric hosts not only increase the mixed ionic–electronic conduction but also act as a sort of protection to the decomposition of active materials (Sengodu and Deshmukh, 2015). For instance, in the case of lithium-ion battery cathode materials: hybrid P3HT-co-PEO/LiFePO4 has improved the delivery of both ionic and electronic charge to active centers (Javier et al., 2011); Ppy/LiFePO4 with different hierarchical structures promoted both electronic and ionic transport (Fedorkova et al., 2010; Shi et al., 2017); PEDOT/LiFePO4 offers excellent discharge capacity (Vadivel Murugan et al., 2008); Ppy/α-LiFeO2 has improved the reversible capacity and cycling stability (Zhang et al., 2013); PPy/MoO3, PPy/V2O5, PPy/LiCoO2, and PPy/LiV3O8 yielded a reduction of charge transfer resistance of the Li+ ion intercalation/deintercalation process (Wang et al., 2010; Tian et al., 2011; Tang et al., 2012a,b; Liu et al., 2013); and PEDOT-co-PEG/LiNi0.6Co0.2Mn0.2O2 showed high discharge capacity and enhanced transport of Li+ ions as well as electrons (Ju et al., 2014). Furthermore, in the case of lithium-ion anode materials, only to mention some examples, hybrid Ppy/SnO2 yielded a more controlled Li+ diffusion (Yuan et al., 2007; Cui et al., 2011) and hybrid PANI-graphene/TiO2 yielded fast charge-to-discharge rate and high enhanced cycling performance (Zhang F. et al., 2012). In the case of sodium-ion battery cathode materials, inorganic NaXMO2 oxides, NaMPO4 phosphates, and NaM[M'(CN6)] hexacyanometalates (commonly known as Prussian blue analogs) have been tested (Xiang et al., 2015; Liu et al., 2020), and to a lesser extent, some organic MIEC polymers such as the case of Ppy (Zhou et al., 2012, Zhou et al., 2013; Zhu et al., 2013). However, in recent literature, hOI-MIECs started to be studied thoroughly as cathode materials for sodium-ion batteries, (e.g., Ppy/NaMnFe(CN)6 (Li et al., 2015), PANI/ NaNiFe(CN)6 (Wang Z. et al., 2017), PEDOT/ NaMnFe(CN)6 (Wang et al., 2020), and Ppy/NaMnO2 Lu et al., 2020). In the case of sodium-ion battery anode materials, the most frequent hOI-MIECs are based on metallic oxides such as PANI/SnO2 (Zhao et al., 2015) and Ppy/SnO2 (Yuan et al., 2018) and sulfides such as PANI/Co3S4 (Zhou et al., 2016) and Ppy/ZnS (Hou et al., 2017). It is interesting to mention that hOI-MIECs are also extensively used as cathodes of lithium-sulfur (Li-S) batteries such as PEDOT:PSS/S (Yang et al., 2011), Ppy/S (Han et al., 2019), and PANI/S (Wei et al., 2019). The study of MIECs as electrochemical transistors was reported long ago for typically doped Ppy (White et al., 1984), PANI (Paul et al., 1985), and PEDOT (Thackeray et al., 1985) conducting polymers, but the exploration of conducting polymers (principally PEDOT) doped with biocompatible materials such as hyaluronic acid, dextran sulfonate, heparin, pectin, guar gum, and deoxyribonucleic acid is rising fast in recent years, especially for bioelectronics purposes (Mantione et al., 2017; Tekoglu et al., 2019). In addition, a very recent report has shown that the preparation of an organic mixed-conducting particulate composite material based on PEDOT: PSS and chitosan enabled facile and effective electronic bonding between soft and rigid electronics, permitting recording of neurophysiological data at the resolution of individual neurons (Jastrzebska-Perfect et al., 2020). However, to the best of our knowledge, up to now, only carbon nanotubes (but no biocompatible inorganic nanoparticles) have been tested with organic MIECs to be evaluated for bioelectronics applications (Nie et al., 2015; Liu et al., 2019; Reddy et al., 2019; Yu et al., 2019).
Herein, the state of the art of hOI-MIECs with special focus on charge carrier localization and transport at different regions including both bulk and interphase regions was discussed. In this particular case, we have mainly based our discussion by means of useful and versatile instrumental techniques such as micro-Raman and impedance spectroscopy, but other instrumental techniques can be very useful and should be considered to gain more insight into the hOI-MIECs transport mechanism. There is no doubt that hOI-MIECs have shown to be very promising for different applications, ranging from more developed applications (e.g., lithium- and sodium-ion batteries) to more emerging applications (e.g., bioelectronics), as mentioned in the previous section. However, more work is still needed to understand the charge carrier transport mechanism of such complicated systems, in order to pursue the filling of the existent gap between fundamental knowledge and applications. In our opinion, in situ/operando monitoring of hOI-MIECs during working conditions is the ideal strategy to gain more insight into this field. However, as we have discussed in this mini-review, the complexity of these particular systems (biphasic by definition and sometimes intrinsically inhomogeneous) requires the rational design of more simple devices in order to make them accessible to a broader range of in situ characterization experiments. We think that the oncoming focus on these experiments is crucial to shed some light on the structural and microstructural correlations of hOI-MIECs with the charge carrier transport mechanism.
MR, RF, and AM contributed to the conception and design of the study. DM and FP selected, compiled, and organized the literature references database. MR created the schematizations, adaptation of figure artwork, and wrote the first draft of the manuscript. DM, FP, RF, and AM wrote sections of the manuscript. All authors contributed to manuscript revision, read, and approved the submitted version.
The authors declare that the research was conducted in the absence of any commercial or financial relationships that could be construed as a potential conflict of interest.
The authors wish to thank the support of Uruguayan CSIC, ANII, and PEDECIBA institutions as well as the Fondo Vaz Ferreira FVF-188 (D2C2-MEC) research project.
Aldebert, P., Audebert, P., Armand, M., Bidan, G., and Pineri, M. (1986). New chemical synthesis of mixed conductivity polymers. J. Chem. Soc. Chem. Commun. 1636–1638. doi: 10.1039/c39860001636
Aoki, Y., Wiemann, C., Feyer, V., Kim, H.-S., Schneider, C. M., Ill-Yoo, H., et al. (2014). Bulk mixed ion electron conduction in amorphous gallium oxide causes memristive behavior. Nat. Commun. 5:473. doi: 10.1038/ncomms4473
Atkinson, A., Baron, S. A., and Brandon, N. P. (2004). AC impedance spectra arising from mixed ionic electronic solid electrolytes. J. Electrochem. Soc. 151, E1E86–E1E93. doi: 10.1149/1.1690291
Balaguer, M., Solís, C., and Serra, J. M. (2011). Study of the transport properties of the mixed ionic electronic conductor Ce1-xTbxO2-δ+Co(x = 0.1,0.2) and evaluation as oxygen-transport membrane. Chem. Mater. 23, 2333–2343. doi: 10.1021/cm103581w
Barker, J., Pynenburg, R., Koksbang, R., and Saidi, M. Y. (1996). An electrochemical investigation into the lithium insertion properties of LixCoO2. Electrochim. Acta 41, 2481–2488. doi: 10.1016/0013-4686(96)00036-9
Barthet, C., and Guglielmi, M. (1995). Mixed electronic and ionic conductors: a new route to Nafion@-doped polyaniline. J. Electroanal. Chem. 388, 35–44. doi: 10.1016/0022-0728(94)03759-V
Barthet, C., Guglielmi, M., and Baudry, P. (1997). A polyaniline + polyethylene oxide mixture as a composite polymer positive electrode in solid-state secondsary batteries. J. Electroanal. Chem. 431, 145–152. doi: 10.1016/S0022-0728(97)00174-5
Berthelot, R., Carlier, D., and Delmas, C. (2010). Electrochemical investigation of the P2-NaxCoO2 phase diagram. Nat. Mater. 10, 74–80. doi: 10.1038/nmat2920
Cai, S. D., Gao, C. H., Zhou, D. Y., Gu, W., and Liao, L. S. (2012). Study of hole-injecting properties in efficient, stable, and simplified phosphorescent organic light-emitting diodes by impedance spectroscopy. ACS Appl. Mater. Interfaces 4, 312–316. doi: 10.1021/am2013568
Cao, F., and Prakash, J. (2002). A comparative electrochemical study of LiMn2O2O4 spinel thin-film and porous laminate. Electrochim. Acta 47, 1607–1613. doi: 10.1016/S0013-4686(01)00884-2
Chatterjee, K., Mitra, M., Kargupta, K., Ganguly, S., and Banerjee, D. (2013). Synthesis, characterization and enhanced thermoelectric performance of structurally ordered cable-like novel polyaniline–bismuth telluride nanocomposite. Nanotechnology 24:215703. doi: 10.1088/0957-4484/24/21/215703
Chen, C.-C., Huang, B.-C., Lin, M.-S., Lu, Y.-J., Cho, T.-Y., Chang, C.-H., et al. (2010). Impedance spectroscopy and equivalent circuits of conductively doped organic hole-transport materials. Org. Electron. 11, 1901–1908. doi: 10.1016/j.orgel.2010.09.005
Chen, D., Ding, D., Li, X., Waller, G., Xiong, X., El-Sayed, M., et al. (2015). Probing the charge storage mechanism of a pseudocapacitive MnO2 electrode using in operando Raman spectroscopy. Chem. Mater. 27, 6608–6619. doi: 10.1021/acs.chemmater.5b03118
Cheng, C. H. W., Lin, F., and Lonergan, M. C. (2005). Charge transport in a mixed ionically/electronically conducting, cationic, polyacetylene ionomer between ion-blocking electrodes. J. Phys. Chem. B 109, 10168–10178. doi: 10.1021/jp0505431
Crispin, X., Jakobsson, F. L. E., Crispin, A., Grim, P. C. M., Andersson, P., Volodin, A., et al. (2006). the origin of the high conductivity of poly(3,4-ethylenedioxythiophene)–poly(styrenesulfonate) (pedot–pss) plastic electrodes. Chem. Mater. 18, 4354–4360. doi: 10.1021/cm061032+
Cui, L., Shen, J., Cheng, F., Tao, Z., and Chen, J. (2011). SnO2 nanoparticles@polypyrrole nanowires composite as anode materials for rechargeable lithium-ion batteries. J. Power Sources 196:2195. doi: 10.1016/j.jpowsour.2010.09.075
Della Santa, A., De Rossi, D., and Mazzoldi, A. (1997). Performance and work capacity of a polypyrrole conducting polymer linear actuator. Synthetic Metals 90, 93–100. doi: 10.1016/S0379-6779(97)81256-8
Doeff, M. M., Ma, Y., Visco, S. J., and De Jonghe, L. C. (1993). Electrochemical insertion of sodium into carbon. J. Electrochem. Soc. 140, L1L69–L1L70. doi: 10.1149/1.2221153
Dokko, K., Mohamedi, M., Fujita, Y., Itoh, T., Nishizawa, M., Umeda, M., et al. (2001). Kinetic characterization of single particles of LiCoO2 by AC impedance and potential step methods. J. Electrochem. Soc. 148, A4A22–A4A26. doi: 10.1149/1.1359197
Dokko, K., Shi, Q., Stefan, I. C., and Scherson, D. A. (2003). In situ raman spectroscopy of single microparticle Li+-intercalation electrodes. J. Phys. Chem. B 107, 12549–12554. doi: 10.1021/jp034977c
Dong, B. X., Nowak, C., Onorato, J. W., Strzalka, J., Escobedo, F. A., Luscombe, C. K., et al. (2019). Influence of side-chain chemistry on structure and ionic conduction characteristics of polythiophene derivatives: a computational and experimental study. Chem. Mater. 31, 1418–1429. doi: 10.1021/acs.chemmater.8b05257
Fedorkova, A., Alejos, A. N., Romero, P. G., Orinakovac, R., and Kaniansky, D. (2010). Structural and electrochemical studies of PPy/PEG-LiFePO4 cathode material for Li-ion batteries. Electrochim. Acta 55, 943–947. doi: 10.1016/j.electacta.2009.09.060
Flores, E., Novác, P., and Berg, E. J. (2018). In situ and operando Raman spectroscopy of layered transition metal oxides for li-ion battery cathodes. Front. Energy Res. 6:82. doi: 10.3389/fenrg.2018.00082
Francis, C., Fazzi, D., Grimm, S. B., Paulus, F., Beck, S., Hillebrandt, S., et al. (2017). Raman spectroscopy and microscopy of electrochemically and chemically doped high-mobility semiconducting polymers. J. Mater. Chem. C 5, 6176–6184. doi: 10.1039/C7TC01277B
Fu, Y., and Manthiram, A. (2012). Enhanced cyclability of lithium–sulfur batteries by a polymer acid-doped polypyrrole mixed ionic–electronic conductor. Chem. Mater. 24, 3081–3087. doi: 10.1021/cm301661y
Furukawa, Y. (1996). Electronic absorption and vibrational spectroscopies of conjugated conducting polymers. J. Phys. Chem. 100, 15644–15653. doi: 10.1021/jp960608n
Ghosh, S., and Inganäs, O. (2000). Networks of electron-conducting polymer in matrices of ion-conducting polymers applications to fast electrodes. Electrochem. Solid-State Lett. 3, 213–215. doi: 10.1149/1.1391005
Glaudell, A. M., Cochran, J. E., Patel, S. N., and Chabinyc, M. L. (2015). Impact of the doping method on conductivity and thermopower in semiconducting polythiophenes. Adv. Energy Mater. 5:1401072. doi: 10.1002/aenm.201401072
Gómez-Romero, P., and Lira-Cantú, M. (1997). Hy. (brid organic-inorganic electrodes: the molecular material formed between polypyrrole and the phosphomolyhdate anion. Adv. Mater. 9, 144–147. doi: 10.1002/adma.19970090210
Goodenough, J. B. (2000). Oxide-ion conductors by design. Nature 404, 821–823. doi: 10.1038/35009177
Guizard, C., Bac, A., Barboiu, M., and Hovnanian, N. (2001). Hybrid organic-inorganic membranes with specific transport properties: applications in separation and sensors technologies. Separat. Purification Technol. 25, 167–180. doi: 10.1016/S1383-5866(01)00101-0
Han, P., Chung, S.-H., and Manthiram, A. (2019). Designing a high-loading sulfur cathode with a mixed ionic-electronic conducting polymer for electrochemically stable lithium-sulfur batteries. Energy Storage Mater. 17, 317–324. doi: 10.1016/j.ensm.2018.11.002
Han, S., Ul Hassan Alvi, N., Granlöf, L., Granberg, H., Berggren, M., Fabiano, S., et al. (2019). A multiparameter pressure–temperature–humidity sensor based on mixed ionic–electronic cellulose aerogels. Adv. Sci. 6:1802128. doi: 10.1002/advs.201802128
Hou, T., Tang, G., Sun, X., Cai, S., Zheng, C., and Hu, W. (2017). Perchlorate ion doped polypyrrole coated ZnS sphere composites as a sodium-ion battery anode with superior rate capability enhanced by pseudocapacitance. RSC Adv. 7, 43636–43641. doi: 10.1039/C7RA07901J
Huggins, R. A. (2002). Simple method to determine electronic and ionic components of the conductivity in mixed conductors, a review. Ionics 8, 300–313. doi: 10.1007/BF02376083
Huguenin, F., Ferreira, M., Zucolotto, V., Nart, F. C., Torresi, R. M., Oliveira, J.r., et al. (2004). Molecular-level manipulation of V2O5/polyaniline layer-by-layer films to control electrochromogenic and electrochemical properties. Chem. Mater. 16, 2293–2299. doi: 10.1021/cm035171s
Inal, S., Rivnay, J., Suiu, A.-O., Malliaras, G. G., and McCulloch, I. (2018). Conjugated polymers in bioelectronics. Acc. Chem. Res. 51, 1368–1376. doi: 10.1021/acs.accounts.7b00624
Irvine, J. T. S., Sinclair, D. C., and West, A. R. (1990). Electroceramics: characterization by impedance spectroscopy. Adv. Mater. 2, 132–138. doi: 10.1002/adma.19900020304
Jacobs, I. E., Aasen, E. W., Oliveira, J. L., Fonseca, T. N., Roehling, J. D., Li, J., et al. (2016). Comparison of solution-mixed and sequentially processed P3HT:F4TCNQ films: effect of doping-induced aggregation on film morphology. J. Mater. Chem. C 4, 3454–3466. doi: 10.1039/C5TC04207K
Jamnik, J., and Maier, J. (1999). Treatment of the impedance of mixed conductors, equivalent circuit model and explicit approximate solutions. J. Electrochem. Soc. 146, 4183–4188. doi: 10.1149/1.1392611
Jastrzebska-Perfect, P., Spyropoulos, G. D., Cea, C., Zhao, Z., Rauhala, O. J., Viswanathan, A., et al. (2020). Mixed-conducting particulate composites for soft electronics. Sci. Adv. 6:eaaz6z767. doi: 10.1126/sciadv.aaz6767
Javier, A. E., Patel, S. N., Hallinan, Jr D. T., Srinivasan, V., and Balsara, N.P. (2011). Simultaneous electronic and ionic conduction in a block copolymer: application in lithium battery electrodes. Angew. Chem. Int. Ed. 50:9848. doi: 10.1002/anie.201102953
Ju, S. H., Kang, I. S., Lee, Y. S., Shin, D. K., Kim, S., Shin, K., et al. (2014). Improvement of the cycling performance of LiNi0.6Co0.2M2Mn0.2O2 cathode active materials by a dual-conductive polymer coating. ACS Appl. Mater. Interfaces 6, 2546–2552. doi: 10.1021/am404965p
Judeinstein, P., and Sanchez, C. (1996). Hybrid organic-inorganic materials: a land of multidisciplinarity. J. Mater. Chem. 6, 511–525. doi: 10.1039/JM9960600511
Jung, I. H., Hong, C. T., Lee, U.-H., Kang, Y. H., Jang, K.-S., and Cho, S. Y. (2017). High thermoelectric power factor of a diketopyrrolopyrrole-based low bandgap polymer via finely tuned doping engineering. Sci. Rep. 7:44704. doi: 10.1038/srep44704
Kang, I. S., Lee, Y. S., and Kim, D. W. (2014). Improved cycling stability of lithium electrodes in rechargeable lithium batteries. J. Electrochem. Soc. 161, A5A3–A5A7. doi: 10.1149/2.029401jes
Kim, G. H., Shao, L., Zhang, K., and Pipe, K. P. (2013). Engineered doping of organic semiconductors for enhanced thermoelectric efficiency. Nat. Mater. 12:719. doi: 10.1038/nmat3635
Kloster, G. M., Thomas, J. A., Brazis, P. W., Kannewurf, C. R., and Shriver, D. F. (1996). Synthesis, characterization, and transport properties of new mixed ionic-electronic conducting V2O5-polymer electrolyte xerogel nanocomposites. Chem. Mater. 8, 2418–2420. doi: 10.1021/cm9603361
Kumar, R., Pillai, R. G., Pekas, N., Wu, Y., and McCreery, R. L. (2012). Spatially resolved raman spectroelectrochemistry of solid-state polythiophene/viologen memory devices. J. Am. Chem. Soc. 134, 14869–14876. doi: 10.1021/ja304458s
Kumar, S. R. S., Kurra, N., and Alshareef, H. N. (2016). Enhanced high temperature thermoelectric response of sulphuric acid treated conducting polymer thin films. J. Mater. Chem. C 4, 215–221. doi: 10.1039/C5TC03145A
Le Bideau, J., Viau, L., and Vioux, A. (2011). Ionogels, ionic liquid based hybrid materials. Chem. Soc. Rev. 40, 907–925. doi: 10.1039/C0CS00059K
Lee, J. S., Jamnik, J., and Maier, J. (2009). Generalized equivalent circuits for mixed conductors: silver sulfide as a model system. Monatshefte für Chemie 140, 1113–1119. doi: 10.1007/s00706-009-0130-x
Lee, S. H., Park, H., Kim, S., Son, W., Cheong, I. W., and Kim, J. H. (2014). Transparent and flexible organic semiconductor nanofilms with enhanced thermoelectric efficiency. J. Mater. Chem. A 2, 7288–7294. doi: 10.1039/C4TA00700J
Levasseur, S., Ménétrier, M., and Delmas, C. (2002). On the dual effect of Mg doping in LiCoO2 and Li1+δCoO2: structural, electronic properties, and 7Li MAS NMR Studies. Chem. Mater. 14 3584–3590. doi: 10.1021/cm021107j
Li, J., and Khan, I. M. (1991). Mixed (electronic and ionic) conductive solid polymer matrix, synthesis and properties of poly(2,5,8,11,14,17,20,23-octaoxapentacosylmethacrylate)-block-poly(4-vinylpyridine). Makromol. Chem. 192, 3043–3050. doi: 10.1002/macp.1991.021921219
Li, W.-J., Chou, S.-L., Wang, J.-Z., Wang, J.-L., Gu, Q.-F., Liu, H.-K., et al. (2015). Multifunctional conducing polymer coated Na1a+xMnFe(CN)6 cathode for sodium-ion batteries with superior performance via a facile and one-step chemistry approach. Nano Energy 13, 200–207. doi: 10.1016/j.nanoen.2015.02.019
Lim, E., Peterson, K. A., Su, G. M., and Chabinyc, M. L. (2018). Thermoelectric properties of poly(3-hexylthiophene) (P3HT) doped with 2,3,5,6-tetrafluoro-7,8-tetracyanoquinodimethane (F4TCNQ) by vapor-phase infiltration. Chem. Mater. 30, 998–1010. doi: 10.1021/acs.chemmater.7b04849
Lin, Y., Fang, S., Su, D., Brinkman, K. S., and Chen, F. (2015). Enhancing grain boundary ionic conductivity in mixed ionic–electronic conductors. Nat. Commun. 6:6824. doi: 10.1038/ncomms7824
Liu, C., Wang, W., Li, Y., Cui, F., Xie, C., Zhu, L., et al. (2019). PMWCNT/PVDF ultrafiltration membranes with enhanced antifouling properties intensified by electric field for efficient blood purification. J. Membr. Sci. 576, 48–58. doi: 10.1016/j.memsci.2019.01.015
Liu, J., Davis, N. R., Liu, D. S., and Hammond, P. T. (2012). Highly transparent mixed electron and proton conducting polymer membranes. J. Mater. Chem. 22:15534. doi: 10.1039/c2jm32296j
Liu, L. L., Wang, X. J., Zhu, Y. S., Hu, C. L., Wu, Y. P., and Holze, R. (2013). Polypyrrole-coated LiV3O8-nanocomposites with good electrochemical performance as anode material for aqueous rechargeable lithium batteries. J. Power Sources 224, 290–294. doi: 10.1016/j.jpowsour.2012.09.100
Liu, Q., Hu, Z., Chen, M., Zou, C., Jin, H., Wang, S., et al. (2020). The cathode choice for commercialization of sodium-ion batteries: layered transition metal oxides versus prussian blue analogs. Adv. Funct. Mater. 30:1909530. doi: 10.1002/adfm.201909530
Lu, D., Yao, Z. J., Li, Y. Q., Zhong, Y., Wang, X. L., Xie, D., et al. (2020). Sodium-rich manganese oxide porous microcubes with polypyrrole coating as a superior cathode for sodium ion full batteries. J. Colloid Interface Sci. 565, 218–226. doi: 10.1016/j.jcis.2020.01.023
Lu, Z., and Dahn, J. R. (2001). In situ X-ray diffraction study of P2-Na-2/3[Ni1/3Mn2/3]O-2. J. Electrochem. Soc. 148, A1A225–A1A229. doi: 10.1149/1.1407247
Maier, J. (2005). Nanoionics: ion transport and electrochemical storage in confined systems. Nature Materials 4, 805–815. doi: 10.1038/nmat1513
Mantione, D., del Agua, I., Sanchez-Sanchez, A., and Mecerreyes, D. (2017). Poly(3,4-ethylenedioxythiophene) (PEDOT) derivatives: innovative conductive polymers for bioelectronics. Polymers 9:354. doi: 10.3390/polym9080354
Mengistie, D. A., Chen, C.-H., Boopathi, K. M., Pranoto, F. W., Li, L.-J., and Chu, C.-W. (2015). Enhanced thermoelectric performance of pedot:pss flexible bulky papers by treatment with secondary dopants. ACS Appl. Mater. Interfaces 7, 94–100. doi: 10.1021/am507032e
Mengistie, D. A., Wang, P.-C., and Chu, C.-W. (2013). Effect of molecular weight of additives on the conductivity of pedot:pss and efficiency for ito-free organic solar cells. J. Mater. Chem. A 1, 9907–9915. doi: 10.1039/c3ta11726j
Miyatani, S. (1973). Electronic and ionic conduction in (AgxCu1-x)2Se. J. Phys. Soc. Jap. 34, 423–432. doi: 10.1143/JPSJ.34.423
Mombrú, D., Romero, M., Faccio, R., Castiglioni, J., and Mombrú, A. W. (2017a). In situ growth of ceramic quantum dots in polyaniline host via water vapor flow diffusion as potential electrode materials for energy applications. J. Solid State Chem. 250, 60–67. doi: 10.1016/j.jssc.2017.03.016
Mombrú, D., Romero, M., Faccio, R., and Mombrú, A. W. (2017b). Raman and impedance spectroscopy under applied dc bias insights on the electrical transport for donor:acceptor nanocomposites based on poly(vinyl carbazole) and TiO2 quantum dots. J. Phys. Chem. C 121, 23383–23391. doi: 10.1021/acs.jpcc.7b08400
Mombrú, D., Romero, M., Faccio, R., and Mombrú, A. W. (2017c). From positive to negative magnetoresistance behavior at low applied magnetic fields for polyaniline:titania quantum dot nanocomposites. J. Appl. Phys. 121:245106. doi: 10.1063/1.4989831
Mombrú, D., Romero, M., Faccio, R., and Mombrú, A. W. (2018). Raman microscopy insights on the out-of-plane electrical transport of carbon nanotube-doped PEDOT:PSS electrodes for solar cell applications. J. Phys. Chem. B 122, 2694–2701. doi: 10.1021/acs.jpcb.8b00317
Mombrú, D., Romero, M., Faccio, R., and Mombrú, A. W. (2019). Transition from positive to negative electrical resistance response under humidity conditions for PEDOT:PSS-MoS2 nanocomposite thin films. J. Mater. Sci. 30, 5959–5964. doi: 10.1007/s10854-019-00895-z
Murthy, A., and Manthiram, A. (2011). Highly water-dispersible, mixed ionic–electronic conducting, polymer acid-doped polyanilines as ionomers for direct methanol fuel cells. Chem. Commun. 47, 6882–6884. doi: 10.1039/c1cc11473e
Nanda, J., Remillard, J., O'Neill, A., Bernardi, D., Ro, T., Nietering, K. E., et al. (2011). Local state-of-charge mapping of lithium-ion battery electrodes. Adv. Funct. Mater. 21, 3282–3290. doi: 10.1002/adfm.201100157
Nie, C., Ma, L., Xia, Y., He, C., Deng, J., Wang, L., et al. (2015). Novel heparin mimicking polymer brush grafted carbon nanotube/PES composite membranes for safe and efficient blood purification. J. Membr. Sci. 475, 455–468. doi: 10.1016/j.memsci.2014.11.005
Nightingale, J., Wade, J., Moia, D., Nelson, J., and Kim, J.-S. (2018). Impact of molecular order on polaron formation in conjugated polymers. J. Phys. Chem. C 122, 29129–29140. doi: 10.1021/acs.jpcc.8b09706
Nigrey, P. J., Macdiarmid, A. G., and Heeg, A. J. (1978). Electrochemistry of polyacetylene, (CH): electrochemical doping of (CH)x films to the metallic state. J. Chem. Soc. Chem. Commun. 594–595. doi: 10.1039/c39790000594
Nishi, T., Nakai, H., and Kita, A. (2013). Visualization of the state-of-charge distribution in a LiCoO2 cathode by in situ raman imaging. J. Electrochem. Soc. 160, A1A785–A1A788. doi: 10.1149/2.061310jes
Nowy, S., Ren, W., Elschner, A., Lovenich, W., and Brutting, W. (2010). Impedance spectroscopy as a probe for the degradation of organic light-emitting diodes. J. Appl. Phys. 107:054501. doi: 10.1063/1.3294642
Onorato, J. W., and Luscombe, C. K. (2019). Morphological effects on polymeric mixed ionic/electronic conductors. Mol. Syst. Des. Eng. 4, 310–324. doi: 10.1039/C8ME00093J
Park, M., Zhang, X., Chung, M., Less, G. B., and Sastry, A. M. (2010). A review of conduction phenomena in Li-ion batteries. J. Power Sources 195, 7904–7929. doi: 10.1016/j.jpowsour.2010.06.060
Patel, S. N., Javier, A. E., Stone, G. M., Mullin, S. A., and Balsara, N. P. (2012). Simultaneous conduction of electronic charge and lithium ions in block copolymers. ACS Nano 6, 1589–1600. doi: 10.1021/nn2045664
Paul, E. W., Ricco, A. J., and Wrighton, M. S. (1985). Resistance of polyaniline films as a function of electrochemical potential and the fabrication of polyaniline-based microelectronic devices. J. Phys. Chem. 1985, 1441–1447. doi: 10.1021/j100254a028
Paulsen, B. D., Tybrandt, K., Stavrinidou, E., and Rivnay, J. (2020). Organic mixed ionic–electronic conductors. Nat. Mater. 19, 13–26. doi: 10.1038/s41563-019-0435-z
Pignanelli, F., Romero, M., Castiglioni, J., Faccio, R., and Mombrú, A. W. (2019b). Novel synergistic in situ synthesis of lithium-ion poly(ethylene citrate)-TiO2 nanocomposites as promising fluorine-free solid polymer electrolytes for lithium batteries. J. Phys. Chem. Solids 135:109082. doi: 10.1016/j.jpcs.2019.109082
Pignanelli, F., Romero, M., Estéves, M., Fernández-Werner, L., Faccio, R., and Mombrú, A. W. (2019a). Lithium titanate nanotubes as active fillers for lithium-ion polyacrylonitrile solid polymer electrolytes. Ionics 25, 2607–2614. doi: 10.1007/s11581-018-2768-z
Pignanelli, F., Romero, M., Faccio, R., Fernández-Werner, L., and Mombrú, A. W. (2018). Enhancement of lithium-ion transport in poly(acrylonitrile) with hydrogen titanate nanotube fillers as solid polymer electrolytes for lithium-ion battery applications. J. Phys. Chem. C 122, 1492–1499. doi: 10.1021/acs.jpcc.7b10725
Pingel, P., and Neher, D. (2013). Comprehensive picture of p-type doping of P3HT with the molecular acceptor F4TCNQ. Phys. Rev. B 87:115209. doi: 10.1103/PhysRevB.87.115209
Qu, S., Yao, Q., Shi, W., Wang, W., and Chen, L. (2016). The influence of molecular configuration on the thermoelectrical properties of poly(3-hexylthiophene). J. Electron. Mater. 45:8. doi: 10.1007/s11664-015-4045-5
Reddy, S., Xiao, Q., Liu, H. Q., Li, C. P., Chen, S. F., Wang, C., et al. (2019). Bionanotube/poly(3,4-ethylenedioxythiophene) nanohybrid as an electrode for the neural interface and dopamine sensor. ACS Appl. Mater. Interfaces 11, 18254–18267. doi: 10.1021/acsami.9b04862
Renna, L. A., Lenef, J. D., Bag, M., and Venkataraman, D. (2017). Mixed ionic–electronic conduction in binary polymer nanoparticle assemblies. Adv. Mater. Interfaces 1700397, 10397–8. doi: 10.1002/admi.201700397
Riess, I. (2000). Polymeric mixed ionic electronic conductors. Solid State Ionics 136–137, 1119–1130. doi: 10.1016/S0167-2738(00)00607-X
Riess, I. (2003). Mixed ionic-electronic conductors-material properties and applications. Solid State Ionics 157, 1–17. doi: 10.1016/S0167-2738(02)00182-0
Rivnay, J., Inal, S., Collins, B. A., Sessolo, M., Stavrinidou, E., Strakosas, X., et al. (2016). Structural control of mixed ionic and electronic transport in conducting polymers. Nat. Commun. 7:11287. doi: 10.1038/ncomms11287
Romero, M., Faccio, R., Vázquez, S., and Mombrú, A. W. (2016). Enhancement of lithium conductivity and evidence of lithium dissociation for LLTO-PMMA nanocomposite electrolyte. Mater. Lett. 172, 1–5. doi: 10.1016/j.matlet.2016.02.128
Roussel, F., Chen Yu King, R., Kuriakose, M., Depriester, M., Hadj-Sahraoui, A., Gors, C., et al. (2015). Electrical and thermal transport properties of polyaniline/silver composites and their use as thermoelectric materials. Synth. Met. 199, 196–204. doi: 10.1016/j.synthmet.2014.11.020
Saïdi, M. Y., Barker, J., and Koksbang, R. (1996). Thermodynamic and kinetic investigation of lithium insertion in the Li1–xMn2O4 spinel phase. J. Solid State Chem. 122, 195–199. doi: 10.1006/jssc.1996.0101
Sanjeeva Murthy, N. (2016). “Chapter 2: x-ray diffraction from polymers,” in Polymer Morphology: Principles, Characterization, and Processing, 1st Edn, ed Qipeng Guo (Hoboken, NJ: John Wiley & Sons, Inc), 14–36. doi: 10.1002/9781118892756.ch2
Sauvage, F., Laffont, L., Tarascon, J. M., and Baudrin, E. (2007). Study of the insertion/deinsertion mechanism of sodium into Na0.44MnO2. Inorg. Chem. 46, 3289–3294. doi: 10.1021/ic0700250
Scrosati, B., Croce, F., and Persi, L. (2000). Impedance spectroscopy study of PEO-based nanocomposite polymer electrolytes. J. Electrochem. Soc. 147, 1718–1721. doi: 10.1149/1.1393423
Sengodu, P., and Deshmukh, A. (2015). Conducting polymers and their inorganic composites for advanced Li-ion batteries: a review. RSC Adv. 5, 42109–42130. doi: 10.1039/C4RA17254J
Sengwa, R. J., and Dhatarwal, P. (2020). Predominantly chain segmental relaxation dependent ionic conductivity of multiphase semicrystalline PVDF/PEO/LiClO4 solid polymer electrolytes. Electrochim. Acta 338:135890. doi: 10.1016/j.electacta.2020.135890
Shao, Z., and Haile, S. M. (2004). A high-performance cathode for the next generation of solid-oxide fuel cells. Nature 431, 170–173. doi: 10.1038/nature02863
Shi, Y., Zhou, X., Zhang, J., Bruck, A. M., Bond, A. C., Marschilok, A. C., et al. (2017). Nanostructured conductive polymer gels as a general framework material to improve electrochemical performance of cathode materials in Li-Ion batteries. Nano Lett. 17, 1906–1914. doi: 10.1021/acs.nanolett.6b05227
Shin, J. H., and Passerini, S. (2004). PEO- LiN(SO2CF2CF3)2 polymer electrolytes V. Effect of fillers on ionic transport properties. J. Electrochem. Soc. 151, A2A38–A2A45.
Stavrinidou, E., Leleux, P., Rajaona, H., Khodagholy, D., Rivnay, J., Lindau, M., et al. (2013). Direct measurement of ion mobility in a conducting polymer. Adv. Mater. 25, 4488–4493. doi: 10.1002/adma.201301240
Takano, T., Masunaga, H., Fujiwara, A., Okuzaki, H., and Sasaki, T. (2012). Pedot nanocrystal in highly conductive pedot:pss polymer films. Macromolecules 45, 3859–3865. doi: 10.1021/ma300120g
Tang, W., Gao, X. W., Zhu, Y. S., Yue, Y. B., Shi, Y., Wu, Y. P., et al. (2012b). A hybrid of V2O5 nanowires and MWCNTs coated with polypyrrole as an anode material for aqueous rechargeable lithium batteries with excellent cycling performance. J. Mater. Chem. 22, 20143–20145. doi: 10.1039/c2jm34563c
Tang, W., Liu, L., Zhu, Y., Sun, H., Wu, Y., and Zhu, K. (2012a). An aqueous rechargeable lithium battery of excellent rate capability based on a nanocomposite of MoO3 coated with PPy and LiMn2O2O4. Energy Environ. Sci. 5, 6909–6913. doi: 10.1039/c2ee21294c
Tekoglu, S., Wielend, D., Scharber, M. C., Sariciftci, N. S., and Yumusak, C. (2019). Conducting polymer-based biocomposites using deoxyribonucleic acid (DNA) as counterion. Adv. Mater. Technol. 5:1900699. doi: 10.1002/admt.201900699
Tevar, A. D., and Whitacre, J. F. (2010). Relating synthesis conditions and electrochemical performance for the sodium intercalation compound Na4Mn9O18 in aqueous electrolyte. J. Electrochem. Soc. 157, A8A70–A8A75. doi: 10.1149/1.3428667
Thackeray, J. W., White, H. S., and Wrighton, M. S. (1985). Poly(3-methylthiophene)-coated electrodes: optical and electrical properties as a function of redox potential and amplification of electrical and chemical signals using poly(3-methylthiophene)-based microelectrochemical transistors. J. Phys. Chem. 1985, 5133–5140. doi: 10.1021/j100269a048
Thackeray, M. M. (1997). Manganese oxides for lithium batteries. Prog. Solid State Chem. 25, 1–71. doi: 10.1016/S0079-6786(97)81003-5
Tian, F., Liu, L., Yang, Z., Wang, X., Chen, Q., and Wang, X. (2011). Electrochemical characterization of a LiV3O8-polypyrrole composite as a cathode material for lithium ion batteries. Mater. Chem. Phys. 127, 151–155. doi: 10.1016/j.matchemphys.2011.01.051
Udo, L., Elisabeth, M., Nicola, N., and Jurg, D. (2009). Microscopical investigations of pedot:pss thin films. Adv. Funct. Mater. 19, 1215–1220. doi: 10.1002/adfm.200801258
Vadivel Murugan, A., Muraliganth, T., and Manthiram, A. (2008). Rapid microwave-solvothermal synthesis of phospho-olivine nanorods and their coating with a mixed conducting polymer for lithium ion batteries. Electrochem. Commun. 10, 903–906. doi: 10.1016/j.elecom.2008.04.004
Vorotyntsev, M. A., Deslouis, C., Musiani, M. M., Tribollet, B., and Aoki, K. (1999). Transport across an electroactive polymer film in contact with media allowing both ionic and electronic interfacial exchange. Electrochim. Acta 44, 2105–2115. doi: 10.1016/S0013-4686(98)00318-1
Wachsman, E. D., and Lee, K. T. (2011). Lowering the temperature of solid oxide fuel cells. Science 334, 935–939. doi: 10.1126/science.1204090
Wang, G. J., Yang, L. C., Qu, Q. T., Wang, B., Wu, Y. P., and Holze, R. (2010). An aqueous rechargeable lithium battery based on doping and intercalation mechanisms. J. Solid State Electrochem. 14:865. doi: 10.1007/s10008-009-0869-3
Wang, W., Chen, C., Tollan, C., Yang, F., Qin, Y., and Knez, M. (2017). Efficient and controllable vapor to solid doping of the polythiophene P3HT by low temperature vapor phase infiltration. J. Mater. Chem. C 5, 2686–2694. doi: 10.1039/C6TC05544C
Wang, X., Wang, B., Tang, Y., Xu, B. B., Liang, C., Yana, M., et al. (2020). Manganese hexacyanoferrate reinforced by PEDOT coating towards high-rate and long-life sodium-ion battery cathode. J. Mater. Chem. A 8, 3222–3227. doi: 10.1039/C9TA12376H
Wang, Z., Liu, Y., Wu, Z., Guan, G., Zhang, D., Zheng, H., et al. (2017). A string of nickel hexacyanoferrate nanocubes coaxially grown on a CNT@bipolar conducting polymer as a high-performance cathode material for sodium-ion batteries. Nanoscale 9, 823–831. doi: 10.1039/C6NR08765E
Watanabe, M. (1996). Molecular design of ion and ion/electron mixed conducting polymers. Macromol. Symp. 105, 229–233. doi: 10.1002/masy.19961050133
Wei, Y., Yan, Y., Zou, Y., Shi, M., Deng, Q., Zhao, N., et al. (2019). The ternary conductive polymer coated S/BDPC composite cathode for enhancing the electrochemical prospects in Li-S batteries. Surface Coatings Technol. 358, 560–566. doi: 10.1016/j.surfcoat.2018.11.038
White, H. S., Kittlesen, G. P., and Wrighton, M. S. (1984). Chemical derivatization of an array of three gold microelectrodes with polypyrrole: fabrication of a molecule-based transistor. J. Am. Chem. Soc. 11, 5375–5377. doi: 10.1021/ja00330a070
Xiang, X., Zhang, K., and Chen, J. (2015). Recent advances and prospects of cathode materials for sodium-ion batteries. Adv. Mater. 27, 5343–5364. doi: 10.1002/adma.201501527
Yamamoto, J., and Furukawa, Y. (2015). Electronic and vibrational spectra of positive polarons and bipolarons in regioregular poly(3-hexylthiophene) doped with ferric chloride. J. Phys. Chem. B 119, 4788–4794. doi: 10.1021/jp512654b
Yang, T., Zheng, J., Cheng, Q., Hu, Y.-Y., and Chan, C. K. (2017). Composite polymer electrolytes with 6th Li7La3Z3Zr2O12 garnet-type nanowires as ceramic fillers: mechanism of conductivity enhancement and role of doping and morphology. ACS Appl Mater Interfaces 9, 21773–21780. doi: 10.1021/acsami.7b03806
Yang, Y., Yu, G., Cha, J. J., Wu, H., Vosgueritchian, M., Yao, Y., et al. (2011). Improving the performance of lithium-sulfur batteries by conductive polymer coating. ACS Nano 5, 9187–9193. doi: 10.1021/nn203436j
Yokota, I. (1961). On the theory of mixed conduction with special reference to conduction in silver sulfide group semiconductors. J. Phys. Soc. Jap. 16, 2213–2223. doi: 10.1143/JPSJ.16.2213
Yu, X., Zhu, Y., Cheng, C., Zhang, T., Wang, X., and Hsiao, B. S. (2019). Novel thin-film nanofibrous composite membranes containing directional toxin transport nanochannels for efficient and safe hemodialysis application. J. Membr. Sci. 582, 151–163. doi: 10.1016/j.memsci.2019.04.006
Yuan, J., Hao, Y., Zhang, X., and Li, X. (2018). Sandwiched CNT@SnO2@PPy nanocomposites enhancing sodium storage. Colloids Surfaces A 555, 795–801. doi: 10.1016/j.colsurfa.2018.07.023
Yuan, L., Wang, J., Chew, S. Y., Chen, J., Guo, Z. P., Zhao, L., et al. (2007). Synthesis and characterization of SnO2-polypyrrole composite for lithium-ion battery. J. Power Sources 174, 1183–1187. doi: 10.1016/j.jpowsour.2007.06.179
Zhang, F., Cao, H., Yue, D., Zhang, J., and Qu, M. (2012). Enhanced anode performances of polyaniline–TiO2-reduced graphene oxide nanocomposites for lithium ion batteries. Inorg. Chem. 2012, 9544–9551. doi: 10.1021/ic301378j
Zhang, K., Davis, M., Qiu, J., Hope-WeekWeeks, L., and Wang, S. (2012). Thermoelectric properties of porous multi-walled carbon nanotube/polyaniline core/shell nanocomposites. Nanotechnology 23:385701. doi: 10.1088/0957-4484/23/38/385701
Zhang, Q., Sun, Y., Xu, W., and Zhu, D. (2012). Thermoelectric energy from flexible P3HT films doped with a ferric salt of triflimide anions. Energy Environ. Sci. 5, 9639–9644. doi: 10.1039/c2ee23006b
Zhang, Q., Wang, W., Li, J., Zhu, J., Wang, L., Zhu, M., et al. (2013). Preparation and thermoelectric properties of multi-walled carbon nanotube/polyaniline hybrid nanocomposites. J. Mater. Chem. A 1, 12109–12114. doi: 10.1039/c3ta12353g
Zhang, X. W., Wang, C., Appleby, A. J., and Little, F. E. (2002). Composite doped emeraldine–polyethylene oxide-bonded lithium-ion nano-tin anodes with electronic–ionic mixed conduction. Solid State Ionics 150, 383–389. doi: 10.1016/S0167-2738(02)00522-2
Zhang, Z. J., Wang, J. Z., Chou, S. L., Liu, H. K., Ozawa, K., and Li, H. (2013). Polypyrrole-coated α-LiFeO2O nanocomposite with enhanced electrochemical properties for lithium-ion batteries. Electrochim. Acta 108, 820–826. doi: 10.1016/j.electacta.2013.06.130
Zhao, X., Zhang, Z., Yang, F., Fu, Y., Lai, Y., and Li, J. (2015). Core–shell structured SnO2O hollow spheres–polyaniline composite as an anode for sodium-ion batteries. RSC Adv. 5, 31465–31471. doi: 10.1039/C5RA02834E
Zheng, J., Tang, M., and Hu, Y. Y. (2016). Lithium ion pathway within Li7La3Z3Zr2O12-polyethylene oxide composite electrolytes. Angew. Chem. Int. Ed. 55, 12538–12542. doi: 10.1002/anie.201607539
Zhou, M., Xiong, Y., Cao, Y., Ai, X., and Yang, H. (2013). Electroactive organic anion-doped polypyrrole as a low cost and renewable cathode for sodium-ion batteries. J. Polym. Sci. B. 51:114. doi: 10.1002/polb.23184
Zhou, M., Zhu, L. M., Cao, Y. L., Zhao, R. R., Qian, J. F., Ai, X. P., et al. (2012). Fe(CN)6–4-doped polypyrrole: a high-capacity and high-rate cathode material for sodium-ion batteries. RSC Adv. 2, 5495–5498. doi: 10.1039/c2ra20666h
Zhou, Q., Liu, L., Huang, Z., Yi, L., Wang, X., and Cao, G. (2016). Co3S4@polyaniline nanotubes as high-performance anode materials for sodium ion batteries. J. Mater. Chem. A 4, 5505–5516. doi: 10.1039/C6TA01497F
Zhu, L. M., Shen, Y. F., Sun, M. Y., Qian, J. F., Cao, Y., Ai, X., et al. (2013). Self-doped polypyrrole with ionizable sodium sulfonate as a renewable cathode material for sodium ion batteries. Chem. Commun. 49, 11370–11372. doi: 10.1039/c3cc46642f
Keywords: hybrid organic–inorganic composites, nanomaterials, mixed ionic–electronic conducting materials, semiconductor, Raman micro spectroscopy, impedance spectroscopy
Citation: Romero M, Mombrú D, Pignanelli F, Faccio R and Mombrú AW (2020) Mini-Review: Mixed Ionic–Electronic Charge Carrier Localization and Transport in Hybrid Organic–Inorganic Nanomaterials. Front. Chem. 8:537. doi: 10.3389/fchem.2020.00537
Received: 16 April 2020; Accepted: 26 May 2020;
Published: 14 July 2020.
Edited by:
Rengaraj Selvaraj, Sultan Qaboos University, OmanReviewed by:
Xiaopeng Han, Tianjin University, ChinaCopyright © 2020 Romero, Mombrú, Pignanelli, Faccio and Mombrú. This is an open-access article distributed under the terms of the Creative Commons Attribution License (CC BY). The use, distribution or reproduction in other forums is permitted, provided the original author(s) and the copyright owner(s) are credited and that the original publication in this journal is cited, in accordance with accepted academic practice. No use, distribution or reproduction is permitted which does not comply with these terms.
*Correspondence: Mariano Romero, bXJvbWVyb0BmcS5lZHUudXk=; Ricardo Faccio, cmZhY2Npb0BmcS5lZHUudXk=; Alvaro W. Mombrú, YW1vbWJydUBmcS5lZHUudXk=
Disclaimer: All claims expressed in this article are solely those of the authors and do not necessarily represent those of their affiliated organizations, or those of the publisher, the editors and the reviewers. Any product that may be evaluated in this article or claim that may be made by its manufacturer is not guaranteed or endorsed by the publisher.
Research integrity at Frontiers
Learn more about the work of our research integrity team to safeguard the quality of each article we publish.