- 1Laboratorio di Nanomedicina, Dipartimento di Scienze Biomediche e Cliniche “L. Sacco”, Università di Milano, Milan, Italy
- 2Laboratorio di Nanomedicina e Imaging Molecolare, Istituti Clinici Scientifici Spa-Società Benefit IRCCS, Pavia, Italy
Indocyanine green (ICG) is a Food and Drug Administration–approved near-infrared fluorescent dye, employed as an imaging agent for different clinical applications due to its attractive physicochemical properties, high sensitivity, and safety. However, free ICG suffers from some drawbacks, such as relatively short circulation half-life, concentration-dependent aggregation, and rapid clearance from the body, which would confine its feasible application in oncology. Here, we aim to discuss encapsulation of ICG within a nanoparticle formulation as a strategy to overcome some of its current limitations and to enlarge its possible applications in cancer diagnosis and treatment. Our purpose is to provide a short but exhaustive overview of clinical outcomes that these nanocomposites would provide, discussing opportunities, limitations, and possible impacts with regard to the main clinical needs in oncology.
Introduction
Recently, growing attention has been addressed to nanocarriers for Indocyanine green (ICG) delivery with the purpose of overcoming some of its current limitations and to expand its possible applications in cancer diagnosis and treatment (Wang et al., 2018). ICG is a widely investigated near infrared (NIR) fluorescent agent, approved for clinical use by the Food and Drug Administration (FDA) in the 1950s (Landsman et al., 1976; Alius et al., 2018). Over the past decade, NIR optical imaging using ICG has become determining for a variety of applications, including lymphangiography, intra-operative lymph node (LN) identification, tissue perfusion, detection of vital structures, and tumor imaging (Fox and Wood, 1960; Starosolski et al., 2017). ICG displays several advantages thoroughly verified during its long clinical use: it is easy to use, cost-effective, radiation-free, and safe. Although ICG fluorescent imaging represents a promising medical tool, its application remains limited due to the intrinsic issues related to ICG degradation and rapid blood clearance (Muckle, 1976; Saxena et al., 2003; Zheng et al., 2012). Therefore, many studies suggest that the exploitation of ICG-based nano-formulations (micelles, polymeric nanoparticles, silica nanoparticles, and liposomes) could boost the efficacy, specificity, and biosafety of this imaging agent for potential oncological applications (Yan et al., 2016; Egloff-Juras et al., 2019).
Properties of ICG
ICG is an amphiphilic tricarbocyanine dye used in the biomedical field for almost six decades for different purposes (Schaafsma et al., 2011; Hill et al., 2015). ICG is an anionic, water-soluble, and fluorescent molecule with a molecular weight of 751 Da and that displays absorption and fluorescence emission in the NIR wavelength region (Zhao et al., 2014). These properties allow deep penetration of the signal and minimize interference of tissues' autofluorescence, making it suitable for bio-imaging uses (Wang et al., 2004; Yuan et al., 2004). Moreover, since it is an FDA-approved dye, well studied in its already known clinical applications, its introduction to new clinical applications is greatly simplified (Alander et al., 2012; Valente et al., 2019). Additionally, due to its photosensitizing properties, ICG can be used to generate oxygen species (ROS) or heat, aiming to destroy cancer cells in photodynamic therapy (PDT) and photothermal therapy (PTT) (Dolmans et al., 2003; Kuo et al., 2012). Despite these compelling properties, the application of ICG is restricted due to its concentration-dependent aggregation, quick degradation, and poor photostability. Furthermore, its non-specific binding to plasma proteins determines a relatively short circulation half-life, and its non-specific targeting remains a limitation (Kirchherr et al., 2009; Yaseen et al., 2009).
ICG as an NIR Fluorescent Contrast Agent: Clinical Applications
As previously mentioned, ICG has an excellent safety profile and, following injection of a clinical standard dose (0.1–0.5 mg/kg), immediately interacts with plasma proteins, acting as an excellent vascular agent for evaluating both the blood perfusion and lymphatic drainage (Alford et al., 2009; Marshall et al., 2010; Boni et al., 2015). Once excited at the wavelength of about 820 nm, ICG emits a fluorescent signal detectable by specific scopes and cameras to allow identification of anatomical structures where the dye localizes (Luo et al., 2011; Daskalaki et al., 2014). Indeed, ICG is used in intraoperative angiography for assessment of superficial eye vessels and in the evaluation of coronary artery bypass grafts, peripheral vascular disease, and solid organ transplantation (Reuthebuch et al., 2004; Sekijima et al., 2004; Desai et al., 2006; Kang et al., 2010a,b; Baillif et al., 2011). Moreover, since, once injected intravenously, ICG is excreted exclusively via the liver, it is used to assess hepatic function (Daskalaki et al., 2014). In addition to these applications, ICG is employed in NIR fluorescence image-guided oncologic surgery with the purpose of identifying structures that need to be resected (e.g., tumor tissue, lymph nodes) and spared, contributing to support the surgeon's decision-making process (Boni et al., 2015; Baiocchi et al., 2018).
Of note, NIR fluorescence imaging via ICG can provide real-time identification of tumor margins and affected lymph nodes (LN) in breast and skin cancers, improving local control of the disease and allowing a more conservative surgery (Sevick-Muraca et al., 2008; Fujiwara et al., 2009; Murawa et al., 2009). Sentinel LN (SLN) mapping is important to detect involved LN and is required for cancer staging, prognosis prediction, and therapy selection (Schaafsma et al., 2011; Wang et al., 2018). Here, ICG is injected near the tumor and flows via lymph circulation to LN, displaying them when lit with excitation light (Tanaka et al., 2006; Alander et al., 2012; Wishart et al., 2012; Verbeek et al., 2014). ICG-NIR fluorescence imaging has been applied also to intraoperative tumor detection in order to ensure a total tumor resection (Gotoh et al., 2009; Onda et al., 2016; Rossi et al., 2018). Indeed, exploiting ICG hepatic clearance and the enhanced permeability and retention (EPR) effect, liver tumors could be identified (Ishizawa et al., 2009; Huang et al., 2018).
To date, the application of ICG as an NIR fluorescence imaging agent in oncology is an active and promising area, but it also has limitations. Aside from the problems inherent with some of its physicochemical properties, ICG is a non-targeted or extremely low targeted tracer, which greatly precludes its application for specific cancer imaging (Landsman et al., 1976; Marshall et al., 2010; Wang et al., 2018; Egloff-Juras et al., 2019).
ICG-NPs and Cancer: Preclinical Studies
Recently, the development of multifunctional ICG-NPs, offering both diagnostic and therapeutic solutions in cancer, has captured the attention of researchers (Han et al., 2018). To overcome the limitations previously discussed, several ICG-NPs have been proposed and tested, both in vitro and in vivo (Liu et al., 2019b; ZhuGe et al., 2019), displaying increased circulation time and improved ICG optical properties and achieving tumor-specific accumulation. Many advantages derive from their use: combining or encapsulating it to/into NPs results in the extension of ICG half-life. Additionally, functionalization with specific cancer-related antibodies may result in preferential accumulation of ICG at the tumor site. Furthermore, ICG-NPs may be useful to limit ICG aggregation and photodegradation as well as to improve its stability in aqueous solutions (Ishizawa et al., 2009; Liu et al., 2019c; ZhuGe et al., 2019). ICG has been loaded or conjugated to a variety of nanostructures, such as polymer-based NPs, lipid-based NPs, and silica NPs with different surface modifications and functionalization strategies (Figure 1A). Among the plethora of ICG-NPs for different targets and applications, we focused on the following main applications for cancer treatment: PDT and PTT (i), in vivo imaging and image-guided surgery (ii), and multimodal therapy (iii) (Figure 1B). A summary of all significant examples of ICG-NPs developed for these applications has been inserted in Table 1.
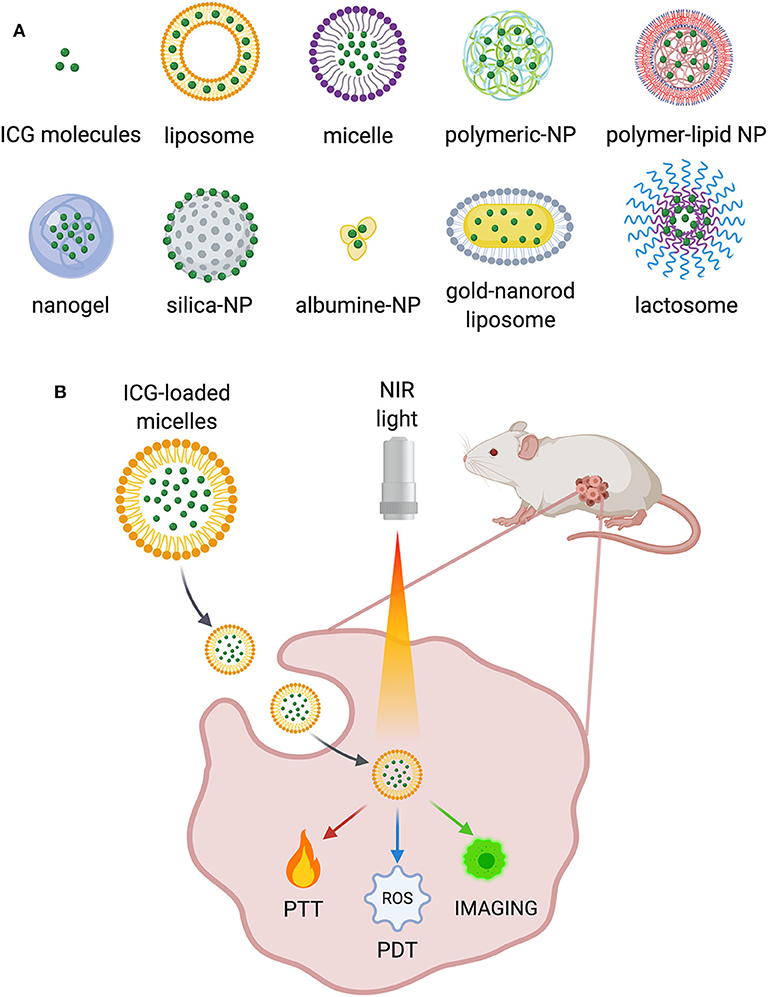
Figure 1. (A) Some examples of the ICG-NPs obtained with different materials and conjugation techniques. (B) Main applications of ICG-NPs in cancer treatment.
PDT is an emerging, minimally invasive cancer treatment based on the production of ROS in response to a source of light, the presence of oxygen and a photosensitizer (i.e., ICG) (ZhuGe et al., 2019). Although this option seems promising for many cancers (Gross et al., 2003; Ritch and Punnen, 2017), selective delivery of the photosensitizers at target tissues/cells remains insufficient for successful clinical use (Zhen et al., 2013). Since PDT could generate an antitumor immune response, ICG-loaded liposomes were studied in combination with NIR irradiation, demonstrating suppression of brain tumor growth and suggesting the potential application for the treatment of tumors near the brain surface (Shibata et al., 2019). Super carbonate apatite-ICG NPs improved ICG uptake in tumor cells and its antitumor effect in a colorectal xenograft model, serving as a useful vehicle for ICG-based PDT (Tamai et al., 2018). Also, hydroxyethyl starch-oleic acid ICG-NPs exhibited excellent stability and efficient ROS generation and increased cellular uptake and tumor accumulation compared to free ICG (Hu et al., 2019).
In addition to PDT, PTT also arose as a promising approach for cancer treatment by using NIR-light to generate heat and achieve tumor ablation (Li et al., 2019a). The main challenge with PTT is that heat could also damage the healthy surrounding tissue and fail to eradicate metastatic cells. Several NPs with excellent NIR light absorption have been developed as PTT agents, including gold, copper, carbon NPs, and NIR dyes (Lv et al., 2017). Also, ICG has been exploited in a multitude of NPs as a PTT agent (Doughty et al., 2019). ICG-conjugated micelles have been investigated for breast and lung cancer treatment displaying increased circulation time, accurate tumor targeting, and efficient PTT effect compared with free ICG (Li et al., 2019b; Zhu et al., 2019). Another work proposed the functionalization with folic acid to achieve accumulation on MCF-7 breast cancer cells, obtaining a significant tumor growth inhibition (Zheng et al., 2014). A formulation of pH-responsive polymeric nano-complexes of graphene oxide and ICG (Sharker et al., 2015) was effective in providing selective sensitivity to tumor environment and tumor regression, confirming its clinical usefulness. Also, in studies with ICG-loaded polymer-lipid NPs against pancreatic cancer (Zhao et al., 2014) and silk fibroin NPs addressing glioblastoma (Xu et al., 2018), the main advantages observed, compared to free ICG, were an extended circulation time and in vivo stability, together with the ability to specifically target cancer cells (Sheng et al., 2019).
An assortment of ICG-NPs also has been developed for bioimaging applications as agents for tumor identification. Since early detection is crucial for the prompt diagnosis and successful treatment of cancer, the benefits of using NPs as vector for ICG to the tumor site would be significant. ICG-incorporating liposomes provide enhanced visualization of the popliteal LN and downstream LN, detected across 1.5 cm of muscle tissue, and free ICG only enables 0.5 cm detection (Kraft and Ho, 2014). Hyaluronic acid (HA) NPs allow contrast enhancement (Hill et al., 2015), and levan NPs display good targeted imaging of breast tumors and the suitability to encapsulate hydrophobic drugs (Kim et al., 2015). ICG-HA–derived NPs improve the NIR signal for intraoperative detection of pancreas and splenic metastasis compared to ICG (Qi et al., 2018), and also nanogels display good performance in targeted imaging of cancers and LN metastases in addition to the feasible drug-encapsulation in their hydrophobic core (Mok et al., 2012). ICG-doped calcium phosphate NPs display increased deep-tissue penetration (Altinoglu et al., 2008). Another promising strategy involves its effectiveness as a photoacoustic-fluorescence imaging probe in liver cancer detection (Guan et al., 2017). Overall, all the considered formulations display a non-toxic safety profile, a longer circulation time, and a higher tumor accumulation than free ICG. This would provide the potential to increase the completeness of surgery and the chances of a better outcome. Furthermore, different ICG-NPs were suggested as contrast agents for SLN mapping. Mannosilated ICG-liposomes show improved stability and fluorescence signal by exploiting their specific recognition by macrophages, making it a good agent for SLN and LN imaging (Jeong et al., 2013). Silica NPs loaded with technetium and ICG improve LN detection in real time although further studies are necessary to assess the appropriate dose (Tsuchimochi et al., 2013), and ICG-loaded lactosomes provide an improved LN detection and a inhibited growth upon PDT treatment (Tsujimoto et al., 2015). The only example tested in the clinic concerns the use of ICG adsorbed to human serum albumin (ICG:HSA) aiming to improve detection and better retention in the SLN after intradermal injection. However, this trial performed on breast cancer patients showed no advantage of ICG:HSA for SLN mapping (Hutteman et al., 2011).
Very often, the applications described above have been used in combination to obtain better therapeutic results. Indeed, many authors consider the use of ICG for imaging-guided PTT, allowing simultaneously tumor detection and eradication. ICG-PL-PEG NPs were investigated in vitro for cell imaging and selective PTT, proving to be an interesting multifunctional system (Zheng et al., 2011), and Liu and coworkers provide a synergistic strategy for both offering contrast enhancement and tumor growth reduction against ovarian cancer (Liu et al., 2019c). Furthermore, the successful application of HSA-ICG NPs for in vivo imaging and tumor margin detection following PDT/PTT synergic phototherapy has been reported (Sheng et al., 2014). Many authors also insist on the strength of the synergistic combination of PTT and PDT to obtain better therapeutic results (Ren et al., 2017; Sheng et al., 2019; Zhu et al., 2019).
Moreover, since monotherapy, either PTT or PDT usually suffers from incomplete tumor killing, leading potentially to tumor relapse (Ma et al., 2018); a combination with chemotherapy could optimize the cancer treatment. In this context, chemotherapy drugs combined with phototherapy have been studied. Doxorubicin has been exploited in superparamagnetic iron oxide NPs with ICG displaying good imaging ability, showing accumulation in the tumor site and high antitumor efficacy with few side effects in glioma-bearing rats (Shen et al., 2018). Phospholipid-calcium-carbonate NPs loaded with doxorubicin and ICG demonstrate strong tumor-homing properties and a synergistic effect in terms of tumor growth reduction (Liu et al., 2019b). Regarding cancer immunotherapy, the photothermal ablation of tumors with immune-adjuvant ICG-NPs, seems promising in activating immune responses potentially applicable for metastasis treatment (Chen et al., 2016). Overall, multimodal therapies appear to enhance the therapeutic effects and prevent possible recurrences.
ICG-NPs: Opportunities, Limitations and Possible Impacts in Oncology
Implementing a plethora of different ICG-NPs makes the comparison between them especially difficult. Overall, the most promising strategies are related to actively targeted ICG-NPs, but there is a significant gap in outcomes between preclinical cancer models and their translation into clinical practice. As previously discussed, several recent studies are focusing on the development of ICG-NPs, aiming to exploit the advantages of ICG in order to further increase cancer therapies.
First, ICG-NPs could be effective in improving the already existing imaging techniques, either by prolonging ICG half-life or by selectively addressing the molecule to cancer cells only; that could be particularly relevant when trying to identify metastases as well as being useful to early detection of cancer cells in case of relapse. The chance to use NPs-ICG as drugs to directly treat tumors represents an additional advantage. By exploiting the ability of ICG to both generate heat and ROS in response to NIR, ICG-NPs could be used for PTT, PDT, or both in order to elicit antitumor response (Han et al., 2018; Liu et al., 2019c). Indeed, the accumulation of ICG in tumor cells and their exposure to light, determines a localized increase in temperature that causes cell damage by apoptosis and necrosis, resulting in tumor ablation (Melamed et al., 2015; Pérez-Hernández et al., 2015). In the meantime, in the presence of oxygen, light-activated ICG also generates ROS, leading to cell death and tissue destruction (Allison and Moghissi, 2013). Nevertheless, recent studies are quite misleading about the application of PTT and PDT, which rely on different therapeutic mechanisms. However, when ICG is used, both effects could be achieved although with a distinct tumor cell–killing contribution by each (Liu et al., 2019a). More clarity about which strategy is being referred to is necessary since, through ICG, one thing does not exclude the other, and the related side effects should be considered as well. Both PTT and PDT are promising for the treatment of several malignancies; however, it is important to understand which aspect to target to design the appropriate NPs (Pinto and Pocard, 2018).
The first concern is about immunogenicity. Although it is common opinion that ICG itself is not toxic and that ICG-NPs can be selectively targeted to the tumor, it is still uncertain if other tissues could be affected by the treatment. NPs must not elicit an immune response and demonstrate not to be toxic for the organism.
A second issue is obvious: cancer is not a single disease. Tumors may be solid or not, have clear or irregular borders, spread in easily reachable districts or in hard-to-treat areas. Additionally, similar tumors may have different density, vasculature, and tumor microenvironment. Here, the ability of ICG-NPs to penetrate into the tumor is unclear, thus making it hard to decide if ICG-NPs should be addressed to primary tumors and metastases or used as adjuvants after surgery (Tsujimoto et al., 2015; Sheng et al., 2019). Research on ICG-NPs should develop the optimal strategy for real clinical applications on each disease instead of just developing NPs that prove to be effective at a preclinical stage but are difficult to translate into clinical trials.
Regarding the multitude of developed ICG-NPs, one fact is evident: every group focuses on the NP type they are used to working with as well as on the tumor model they know better (Table 1). Such an approach is both useful and harmful. On the one hand, long-term expertise in developing a specific NP could be addressed to the production of highly effective ICG-NPs; on the other hand, several cancer models should be tested to evaluate the efficacy of the proposed treatment in different contexts. Focusing on a single model could be limiting if the purpose is to design a consistent model for the development of new therapies. Options to design ICG-NPs are unlimited, and many could be adjusted to target different tumors (Han et al., 2018). NPs can be further enriched by conjugation with other molecules: monoclonal antibodies, fluorescence probes and drugs in order to maximize the antitumor effect of the ICG-NPs (Zheng et al., 2011; Sheng et al., 2014; Ma et al., 2018). Here, given that the enhancement of targeting is crucial to prevent damage of healthy tissues, NPs should be selectively directed to the tumor, either inserting targeting molecules or by exploiting the intrinsic ability of some carriers to bind cancer.
Despite all the advantages deriving from ICG-NPs and the huge amount of solutions proposed so far, a pool of clinical applications have not been outlined yet, thus making it impossible to determine which ones have the potential to become actual drugs. The answer is not one and only: different nanoparticles could be used to treat different tumors, and different molecules could be attached to improve their selectivity. Therefore, developing dozens of different ICG-NPs is not of help in finding the best alternatives that could be eventually tested in clinical trials.
On top of everything, when discussing the potentiality of ICG-NPs in therapy, an uncomfortable yet necessary question should be raised about the costs of clinical trials involving NPs: developing such molecules, especially when they are combined with chemotherapeutic drugs and/or patented monoclonal antibodies, has been proved to be extremely expensive, thus limiting the possibility to produce high amounts of molecules to be used in clinical trials.
Discussion
In conclusion, the potential of ICG-conjugated NPs is undeniable, mostly because they could possibly be directed toward several cancer types with incredibly high specificity (Bozkulak et al., 2009; Montazerabadi et al., 2012). Indeed, they could overcome some of the limitations of current treatments, especially regarding tumors that are poorly accessible by drugs or hard to treat, and they could also limit the side effects usually associated to conventional therapies (Montazerabadi et al., 2012). However, obtaining specific cell targeting as well as maintaining high drug concentration at the tumor site remain the main challenges as they are both necessary conditions for the implementation of ICG-NPs–based imaging, PTT, and PDT. This is the reason why the currently known ICG-NPs have not successfully reached the translation into clinics so far. Therefore, an improved active targeting is required for a major impact on human health. Moreover, since the penetration depth of light in tissues could be limited, even with NIR lasers, endoscope-based clinical devices equipped with a laser may be demanded in order to reach successful outcomes in clinical practice (Chen et al., 2016). Some sort of consensus should also be achieved about the most promising formulations and the real aims of the proposed interventions: PTT, PDT, and imaging are not interchangeable terms, and more precision is required when deciding which therapies would be worth testing. In addition, before trying to develop new ICG-NPs, the economic impact of a potential trial involving such NPs must be carefully considered. Expertise and deep knowledge in the field are mandatory, but feasibility will eventually determine if a promising molecule will ever be translated into an actual therapy.
Author Contributions
MS, FS, AB, and LS contributed in manuscript writing and figure preparation. SM, MT, and FC contributed in manuscript writing, editing, and critical review. All authors contributed to the article and approved the submitted version.
Conflict of Interest
The authors declare that the research was conducted in the absence of any commercial or financial relationships that could be construed as a potential conflict of interest.
Acknowledgments
SM acknowledges Pediatric Clinical Research Center Romeo and Enrica Invernizzi that supports her position. LS and AB postdoctoral and doctoral fellowships are supported by University of Milan. The authors acknowledge University of Milan for support in open access publication.
References
Alander, J. T., Kaartinen, I., Laakso, A., Pätilä, T., Spillmann, T., Tuchin, V. V., et al. (2012). A review of indocyanine green fluorescent imaging in surgery. Int. J. Biomed. Imaging 2012, 1–26. doi: 10.1155/2012/940585
Alford, R., Simpson, H. M., Duberman, J., Hill, G. C., Ogawa, M., Regino, C., et al. (2009). Toxicity of organic fluorophores used in molecular imaging: literature review. Mol. Imaging 8, 341–354. doi: 10.2310/7290.2009.00031
Alius, C., Oprescu, S., Balalau, C., and Elena Nica, A. (2018). Indocyanine green enhanced surgery; principle, clinical applications and future research directions. J. Clin. Invest. Surg. 3, 1–8. doi: 10.25083/2559.5555/31.18
Allison, R. R., and Moghissi, K. (2013). Photodynamic therapy (PDT): PDT mechanisms. Clin. Endosc. 46, 24–29. doi: 10.5946/ce.2013.46.1.24
Altinoglu, E. I., Russin, T. J., Kaiser, J. M., Barth, B. M., Eklund, P. C., Kester, M., et al. (2008). Near-infrared emitting fluorophore-doped calcium phosphate nanoparticles for in vivo imaging of human breast cancer. ACS Nano 2, 2075–2084. doi: 10.1021/nn800448r
Baillif, S., Wolff, B., Paoli, V., Gastaud, P., and Mauget-Faýsse, M. (2011). Retinal fluorescein and indocyanine green angiography and spectral-domain optical coherence tomography findings in acute retinal pigment epitheliitis. Retina 31, 1156–1163. doi: 10.1097/IAE.0b013e3181fbcea5
Baiocchi, G. L., Diana, M., and Boni, L. (2018). Indocyanine green-based fluorescence imaging in visceral and hepatobiliary and pancreatic surgery: state of the art and future directions. World J. Gastroenterol. 24, 2921–2930. doi: 10.3748/wjg.v24.i27.2921
Boni, L., David, G., Mangano, A., Dionigi, G., Rausei, S., Spampatti, S., et al. (2015). Clinical applications of indocyanine green (ICG) enhanced fluorescence in laparoscopic surgery. Surg. Endosc. 29, 2046–2055. doi: 10.1007/s00464-014-3895-x
Bozkulak, O., Yamaci, R. F., Tabakoglu, O., and Gulsoy, M. (2009). Photo-toxic effects of 809-nm diode laser and indocyanine green on MDA-MB231 breast cancer cells. Photodiagnosis. Photodyn. Ther. 6, 117–121. doi: 10.1016/j.pdpdt.2009.07.001
Chen, Q., Xu, L., Liang, C., Wang, C., Peng, R., and Liu, Z. (2016). Photothermal therapy with immune-adjuvant nanoparticles together with checkpoint blockade for effective cancer immunotherapy. Nat. Commun. 7:13193. doi: 10.1038/ncomms13193
Daskalaki, D., Fernandes, E., Wang, X., Bianco, F. M., Elli, E. F., Ayloo, S., et al. (2014). Indocyanine green (ICG) fluorescent cholangiography during robotic cholecystectomy: results of 184 consecutive cases in a single institution. Surg. Innov. 21, 615–621. doi: 10.1177/1553350614524839
Desai, N. D., Miwa, S., Kodama, D., Koyama, T., Cohen, G., Pelletier, M. P., et al. (2006). A randomized comparison of intraoperative indocyanine green angiography and transit-time flow measurement to detect technical errors in coronary bypass grafts. J. Thorac. Cardiovasc. Surg 132, 585–594. doi: 10.1016/j.jtcvs.2005.09.061
Dolmans, D. E. J. G. J., Fukumura, D., and Jain, R. K. (2003). Photodynamic therapy for cancer. Nat. Rev. Cancer 3, 380–387. doi: 10.1038/nrc1071
Doughty, A., Hoover, A., Layton, E., Murray, C., Howard, E., and Chen, W. (2019). Nanomaterial applications in photothermal therapy for cancer. Materials 12:779. doi: 10.3390/ma12050779
Egloff-Juras, C., Bezdetnaya, L., Dolivet, G., and Lassalle, H.-P. (2019). NIR fluorescence-guided tumor surgery: new strategies for the use of indocyanine green. Int. J. Nanomed. 14, 7823–7838. doi: 10.2147/IJN.S207486
Fox, I. J., and Wood, E. H. (1960). Indocyanine green: physical and physiologic properties. Proc. Staff Meet. Mayo Clin 35, 732–744.
Fujiwara, M., Mizukami, T., Suzuki, A., and Fukamizu, H. (2009). Sentinel lymph node detection in skin cancer patients using real-time fluorescence navigation with indocyanine green: preliminary experience. J. Plast. Reconstr. Aesthet. Surg. 62, e373–e378. doi: 10.1016/j.bjps.2007.12.074
Gotoh, K., Yamada, T., Ishikawa, O., Takahashi, H., Eguchi, H., Yano, M., et al. (2009). A novel image-guided surgery of hepatocellular carcinoma by indocyanine green fluorescence imaging navigation. J. Surg. Oncol. 100, 75–79. doi: 10.1002/jso.21272
Gross, S., Gilead, A., Scherz, A., Neeman, M., and Salomon, Y. (2003). Monitoring photodynamic therapy of solid tumors online by BOLD-contrast MRI. Nat. Med. 9, 1327–1331. doi: 10.1038/nm940
Guan, T., Shang, W., Li, H., Yang, X., Fang, C., Tian, J., et al. (2017). From detection to resection: photoacoustic tomography and surgery guidance with indocyanine green loaded gold nanorod@liposome core–shell nanoparticles in liver cancer. Bioconjugate Chem. 28, 1221–1228. doi: 10.1021/acs.bioconjchem.7b00065
Han, Y.-H., Kankala, R. K., Wang, S.-B., and Chen, A.-Z. (2018). Leveraging engineering of indocyanine green-encapsulated polymeric nanocomposites for biomedical applications. Nanomaterials 8:360. doi: 10.3390/nano8060360
Hill, T. K., Abdulahad, A., Kelkar, S. S., Marini, F. C., Long, T. E., Provenzale, J. M., et al. (2015). Indocyanine green-loaded nanoparticles for image-guided tumor surgery. Bioconjugate Chem. 26, 294–303. doi: 10.1021/bc5005679
Hu, H., Chen, J., Yang, H., Huang, X., Wu, H., Wu, Y., et al. (2019). Potentiating photodynamic therapy of ICG-loaded nanoparticles by depleting GSH with PEITC. Nanoscale 11, 6384–6393. doi: 10.1039/C9NR01306G
Huang, S.-W., Ou, J.-J., and Wong, H. P. (2018). The use of indocyanine green imaging technique in patient with hepatocellular carcinoma. Transl. Gastroenterol. Hepatol. 3, 95–95. doi: 10.21037/tgh.2018.10.15
Hutteman, M., Mieog, J. S. D., van der Vorst, J. R., Liefers, G. J., Putter, H., Löwik, C. W. G. M., et al. (2011). Randomized, double-blind comparison of indocyanine green with or without albumin premixing for near-infrared fluorescence imaging of sentinel lymph nodes in breast cancer patients. Breast Cancer Res. Treat. 127, 163–170. doi: 10.1007/s10549-011-1419-0
Ishizawa, T., Fukushima, N., Shibahara, J., Masuda, K., Tamura, S., Aoki, T., et al. (2009). Real-time identification of liver cancers by using indocyanine green fluorescent imaging. Cancer 115, 2491–2504. doi: 10.1002/cncr.24291
Jeong, H.-S., Lee, C.-M., Cheong, S.-J., Kim, E.-M., Hwang, H., Na, K. S., et al. (2013). The effect of mannosylation of liposome-encapsulated indocyanine green on imaging of sentinel lymph node. J. Liposome Res. 23, 291–297. doi: 10.3109/08982104.2013.801488
Kang, Y., Lee, J., Kwon, K., and Choi, C. (2010a). Application of novel dynamic optical imaging for evaluation of peripheral tissue perfusion. Int. J. Cardiol. 145, e99–101. doi: 10.1016/j.ijcard.2008.12.166
Kang, Y., Lee, J., Kwon, K., and Choi, C. (2010b). Dynamic fluorescence imaging of indocyanine green for reliable and sensitive diagnosis of peripheral vascular insufficiency. Microvasc. Res. 80, 552–555. doi: 10.1016/j.mvr.2010.07.004
Kim, S.-J., Bae, P. K., and Chung, B. H. (2015). Self-assembled levan nanoparticles for targeted breast cancer imaging. Chem. Commun. 51, 107–110. doi: 10.1039/C4CC07679F
Kirchherr, A.-K., Briel, A., and Mäder, K. (2009). Stabilization of indocyanine green by encapsulation within micellar systems. Mol. Pharmaceutics 6, 480–491. doi: 10.1021/mp8001649
Kraft, J. C., and Ho, R. J. Y. (2014). Interactions of indocyanine green and lipid in enhancing near-infrared fluorescence properties: the basis for near-infrared imaging in vivo. Biochemistry 53, 1275–1283. doi: 10.1021/bi500021j
Kuo, W.-S., Chang, Y.-T., Cho, K.-C., Chiu, K.-C., Lien, C.-H., Yeh, C.-S., et al. (2012). Gold nanomaterials conjugated with indocyanine green for dual-modality photodynamic and photothermal therapy. Biomaterials 33, 3270–3278. doi: 10.1016/j.biomaterials.2012.01.035
Landsman, M. L., Kwant, G., Mook, G. A., and Zijlstra, W. G. (1976). Light-absorbing properties, stability, and spectral stabilization of indocyanine green. J. Appl. Physiol. 40, 575–583. doi: 10.1152/jappl.1976.40.4.575
Li, Z., Chen, Y., Yang, Y., Yu, Y., Zhang, Y., Zhu, D., et al. (2019a). Recent advances in nanomaterials-based chemo-photothermal combination therapy for improving cancer treatment. Front. Bioeng. Biotechnol. 7:293. doi: 10.3389/fbioe.2019.00293
Li, Z., Yin, Q., Chen, B., Wang, Z., Yan, Y., Qi, T., et al. (2019b). Ultra-pH-sensitive indocyanine green-conjugated nanoprobes for fluorescence imaging-guided photothermal cancer therapy. Nanomedicine 17, 287–296. doi: 10.1016/j.nano.2019.02.001
Liu, P., Yang, W., Shi, L., Zhang, H., Xu, Y., Wang, P., et al. (2019a). Concurrent photothermal therapy and photodynamic therapy for cutaneous squamous cell carcinoma by gold nanoclusters under a single NIR laser irradiation. J. Mater. Chem. B 7, 6924–6933. doi: 10.1039/C9TB01573F
Liu, X., Wang, C., Ma, H., Yu, F., Hu, F., and Yuan, H. (2019b). Water-responsive hybrid nanoparticles codelivering ICG and DOX effectively treat breast cancer via hyperthermia-aided DOX functionality and drug penetration. Adv. Healthcare Mater. 8:1801486. doi: 10.1002/adhm.201801486
Liu, Y., Chen, S., Sun, J., Zhu, S., Chen, C., Xie, W., et al. (2019c). Folate-targeted and oxygen/indocyanine green-loaded lipid nanoparticles for dual-mode imaging and photo-sonodynamic/photothermal therapy of ovarian cancer in vitro and in vivo. Mol. Pharmaceutics 16, 4104–4120. doi: 10.1021/acs.molpharmaceut.9b00339
Luo, S., Zhang, E., Su, Y., Cheng, T., and Shi, C. (2011). A review of NIR dyes in cancer targeting and imaging. Biomaterials 32, 7127–7138. doi: 10.1016/j.biomaterials.2011.06.024
Lv, R., Wang, D., Xiao, L., Chen, G., Xia, J., and Prasad, P. N. (2017). Stable ICG-loaded upconversion nanoparticles: silica core/shell theranostic nanoplatform for dual-modal upconversion and photoacoustic imaging together with photothermal therapy. Sci. Rep. 7:15753. doi: 10.1038/s41598-017-16016-x
Ma, Y., Liu, X., Ma, Q., and Liu, Y. (2018). Near-infrared nanoparticles based on indocyanine green-conjugated albumin: a versatile platform for imaging-guided synergistic tumor chemo-phototherapy with temperature-responsive drug release. Onco. Targets Ther. 11, 8517–8528. doi: 10.2147/OTT.S183887
Marshall, M. V., Rasmussen, J. C., Tan, I.-C., Aldrich, M. B., Adams, K. E., Wang, X., et al. (2010). Near-infrared fluorescence imaging in humans with indocyanine green: a review and update. Open Surg. Oncol. J. 2, 12–25. doi: 10.2174/1876504101002010012
Melamed, J. R., Edelstein, R. S., and Day, E. S. (2015). Elucidating the fundamental mechanisms of cell death triggered by photothermal therapy. ACS Nano 9, 6–11. doi: 10.1021/acsnano.5b00021
Mok, H., Jeong, H., Kim, S.-J., and Chung, B. H. (2012). Indocyanine green encapsulated nanogels for hyaluronidase activatable and selective near infrared imaging of tumors and lymph nodes. Chem. Commun. 48:8628. doi: 10.1039/c2cc33555g
Montazerabadi, A. R., Sazgarnia, A., Bahreyni-Toosi, M. H., Ahmadi, A., and Aledavood, A. (2012). The effects of combined treatment with ionizing radiation and indocyanine green-mediated photodynamic therapy on breast cancer cells. J. Photochem. Photobiol. B 109, 42–49. doi: 10.1016/j.jphotobiol.2012.01.004
Muckle, T. J. (1976). Plasma proteins binding of indocyanine green. Biochem. Med. 15, 17–21. doi: 10.1016/0006-2944(76)90069-7
Murawa, D., Hirche, C., Dresel, S., and Hünerbein, M. (2009). Sentinel lymph node biopsy in breast cancer guided by indocyanine green fluorescence. Br. J. Surg. 96, 1289–1294. doi: 10.1002/bjs.6721
Onda, N., Kimura, M., Yoshida, T., and Shibutani, M. (2016). Preferential tumor cellular uptake and retention of indocyanine green for in vivo tumor imaging: In vivo tumor imaging using indocyanine green. Int. J. Cancer 139, 673–682. doi: 10.1002/ijc.30102
Pérez-Hernández, M., del Pino, P., Mitchell, S. G., Moros, M., Stepien, G., Pelaz, B., et al. (2015). Dissecting the molecular mechanism of apoptosis during photothermal therapy using gold nanoprisms. ACS Nano 9, 52–61. doi: 10.1021/nn505468v
Pinto, A., and Pocard, M. (2018). Photodynamic therapy and photothermal therapy for the treatment of peritoneal metastasis: a systematic review. Pleura and Peritoneum 3:20180124. doi: 10.1515/pp-2018-0124
Qi, B., Crawford, A. J., Wojtynek, N. E., Holmes, M. B., Souchek, J. J., Almeida-Porada, G., et al. (2018). Indocyanine green loaded hyaluronan-derived nanoparticles for fluorescence-enhanced surgical imaging of pancreatic cancer. Nanomedicine 14, 769–780. doi: 10.1016/j.nano.2017.12.015
Ren, S., Cheng, X., Chen, M., Liu, C., Zhao, P., Huang, W., et al. (2017). Hypotoxic and rapidly metabolic PEG-PCL-C3-ICG nanoparticles for fluorescence-guided photothermal/photodynamic therapy against OSCC. ACS Appl. Mater. Interfaces 9, 31509–31518. doi: 10.1021/acsami.7b09522
Reuthebuch, O., Häussler, A., Genoni, M., Tavakoli, R., Odavic, D., Kadner, A., et al. (2004). Novadaq SPY: intraoperative quality assessment in off-pump coronary artery bypass grafting. Chest 125, 418–424. doi: 10.1378/chest.125.2.418
Ritch, C. R., and Punnen, S. (2017). Photodynamic therapy for low risk prostate cancer. BMJ, 356:575. doi: 10.1136/bmj.j575
Rossi, G., Tarasconi, A., Baiocchi, G., De' Angelis, G. L., Gaiani, F., Di Mario, F., et al. (2018). Fluorescence guided surgery in liver tumors: applications and advantages. Acta Biomed. 89, 135–140. doi: 10.23750/abm.v89i9-S.7974
Saxena, V., Sadoqi, M., and Shao, J. (2003). Degradation kinetics of indocyanine green in aqueous solution. J. Pharm. Sci. 92, 2090–2097. doi: 10.1002/jps.10470
Schaafsma, B. E., Mieog, J. S. D., Hutteman, M., van der Vorst, J. R., Kuppen, P. J. K., Löwik, C. W. G. M., et al. (2011). The clinical use of indocyanine green as a near-infrared fluorescent contrast agent for image-guided oncologic surgery. J. Surg. Oncol. 104, 323–332. doi: 10.1002/jso.21943
Sekijima, M., Tojimbara, T., Sato, S., Nakamura, M., Kawase, T., Kai, K., et al. (2004). An intraoperative fluorescent imaging system in organ transplantation. Transplant. Proc. 36, 2188–2190. doi: 10.1016/j.transproceed.2004.09.001
Sevick-Muraca, E. M., Sharma, R., Rasmussen, J. C., Marshall, M. V., Wendt, J. A., Pham, H. Q., et al. (2008). Imaging of lymph flow in breast cancer patients after microdose administration of a near-infrared fluorophore: feasibility study. Radiology 246, 734–741. doi: 10.1148/radiol.2463070962
Sharker, S. M, Lee, J. E., Kim, S. H., Jeong, J. H., In, I., et al. (2015). pH triggered in vivo photothermal therapy and fluorescence nanoplatform of cancer based on responsive polymer-indocyanine green integrated reduced graphene oxide. Biomaterials 61, 229–238. doi: 10.1016/j.biomaterials.2015.05.040
Shen, C., Wang, X., Zheng, Z., Gao, C., Chen, X., Zhao, S., et al. (2018). Doxorubicin and indocyanine green loaded superparamagnetic iron oxide nanoparticles with PEGylated phospholipid coating for magnetic resonance with fluorescence imaging and chemotherapy of glioma. Int. J. Nanomedicine 14, 101–117. doi: 10.2147/IJN.S173954
Sheng, Y., Wang, Z., Ngandeu Neubi, G. M., Cheng, H., Zhang, C., Zhang, H., et al. (2019). Lipoprotein-inspired penetrating nanoparticles for deep tumor-targeted shuttling of indocyanine green and enhanced photo-theranostics. Biomater. Sci. 7, 3425–3437. doi: 10.1039/C9BM00588A
Sheng, Z., Hu, D., Zheng, M., Zhao, P., Liu, H., Gao, D., et al. (2014). Smart human serum albumin-indocyanine green nanoparticles generated by programmed assembly for dual-modal imaging-guided cancer synergistic phototherapy. ACS Nano 8, 12310–12322. doi: 10.1021/nn5062386
Shibata, S., Shinozaki, N., Suganami, A., Ikegami, S., Kinoshita, Y., Hasegawa, R., et al. (2019). Photo-immune therapy with liposomally formulated phospholipid-conjugated indocyanine green induces specific antitumor responses with heat shock protein-70 expression in a glioblastoma model. Oncotarget 10, 175–183. doi: 10.18632/oncotarget.26544
Starosolski, Z., Bhavane, R., Ghaghada, K. B., Vasudevan, S. A., Kaay, A., and Annapragada, A. (2017). Indocyanine green fluorescence in second near-infrared (NIR-II) window. PLoS ONE 12:e0187563. doi: 10.1371/journal.pone.0187563
Tamai, K., Mizushima, T., Wu, X., Inoue, A., Ota, M., Yokoyama, Y., et al. (2018). Photodynamic therapy using indocyanine green loaded on super carbonate apatite as minimally invasive cancer treatment. Mol. Cancer Ther. 17, 1613–1622. doi: 10.1158/1535-7163.MCT-17-0788
Tanaka, E., Choi, H. S., Fujii, H., Bawendi, M. G., and Frangioni, J. V. (2006). Image-guided oncologic surgery using invisible light: completed pre-clinical development for sentinel lymph node mapping. Ann. Surg. Oncol. 13, 1671–1681. doi: 10.1245/s10434-006-9194-6
Tsuchimochi, M., Hayama, K., Toyama, M., Sasagawa, I., and Tsubokawa, N. (2013). Dual-modality imaging with 99mTc and fluorescent indocyanine green using surface-modified silica nanoparticles for biopsy of the sentinel lymph node: an animal study. EJNMMI Res. 3:33. doi: 10.1186/2191-219X-3-33
Tsujimoto, H., Morimoto, Y., Takahata, R., Nomura, S., Yoshida, K., Hiraki, S., et al. (2015). Theranostic photosensitive nanoparticles for lymph node metastasis of gastric cancer. Ann. Surg. Oncol. 22, 923–928. doi: 10.1245/s10434-015-4594-0
Valente, S. A., Al-Hilli, Z., Radford, D. M., Yanda, C., Tu, C., and Grobmyer, S. R. (2019). Near infrared fluorescent lymph node mapping with indocyanine green in breast cancer patients: a prospective trial. J. Am. Coll. Surg. 228, 672–678. doi: 10.1016/j.jamcollsurg.2018.12.001
Verbeek, F. P. R., Troyan, S. L., Mieog, J. S. D., Liefers, G.-J., Moffitt, L. A., Rosenberg, M., et al. (2014). Near-infrared fluorescence sentinel lymph node mapping in breast cancer: a multicenter experience. Breast Cancer Res. Treat. 143, 333–342. doi: 10.1007/s10549-013-2802-9
Wang, H., Li, X., Tse, B. W.-C., Yang, H., Thorling, C. A., Liu, Y., et al. (2018). Indocyanine green-incorporating nanoparticles for cancer theranostics. Theranostics 8, 1227–1242. doi: 10.7150/thno.22872
Wang, X., Ku, G., Wegiel, M. A., Bornhop, D. J., Stoica, G., and Wang, L. V. (2004). Noninvasive photoacoustic angiography of animal brains in vivo with near-infrared light and an optical contrast agent. Opt. Lett. 29:730. doi: 10.1364/OL.29.000730
Wishart, G. C., Loh, S.-W., Jones, L., and Benson, J. R. (2012). A feasibility study (ICG-10) of indocyanine green (ICG) fluorescence mapping for sentinel lymph node detection in early breast cancer. Eur. J. Surg. Oncol. 38, 651–656. doi: 10.1016/j.ejso.2012.05.007
Xu, H.-L., ZhuGe, D.-L., Chen, P.-P., Tong, M.-Q., Lin, M.-T., Jiang, X., et al. (2018). Silk fibroin nanoparticles dyeing indocyanine green for imaging-guided photo-thermal therapy of glioblastoma. Drug Deliv. 25, 364–375. doi: 10.1080/10717544.2018.1428244
Yan, F., Wu, H., Liu, H., Deng, Z., Liu, H., Duan, W., et al. (2016). Molecular imaging-guided photothermal/photodynamic therapy against tumor by iRGD-modified indocyanine green nanoparticles. J. Control. Release 224, 217–228. doi: 10.1016/j.jconrel.2015.12.050
Yaseen, M. A., Yu, J., Jung, B., Wong, M. S., and Anvari, B. (2009). Biodistribution of encapsulated indocyanine green in healthy mice. Mol. Pharmaceutics 6, 1321–1332. doi: 10.1021/mp800270t
Yuan, B., Chen, N., and Zhu, Q. (2004). Emission and absorption properties of indocyanine green in Intralipid solution. J. Biomed. Opt. 9, 497–503. doi: 10.1117/1.1695411
Zhao, P., Zheng, M., Yue, C., Luo, Z., Gong, P., Gao, G., et al. (2014). Improving drug accumulation and photothermal efficacy in tumor depending on size of ICG loaded lipid-polymer nanoparticles. Biomaterials 35, 6037–6046. doi: 10.1016/j.biomaterials.2014.04.019
Zhen, Z., Tang, W., Guo, C., Chen, H., Lin, X., Liu, G., et al. (2013). Ferritin nanocages to encapsulate and deliver photosensitizers for efficient photodynamic therapy against cancer. ACS Nano 7, 6988–6996. doi: 10.1021/nn402199g
Zheng, M., Zhao, P., Luo, Z., Gong, P., Zheng, C., Zhang, P., et al. (2014). Robust ICG theranostic nanoparticles for folate targeted cancer imaging and highly effective photothermal therapy. ACS Appl. Mater. Interfaces 6, 6709–6716. doi: 10.1021/am5004393
Zheng, X., Xing, D., Zhou, F., Wu, B., and Chen, W. R. (2011). Indocyanine green-containing nanostructure as near infrared dual-functional targeting probes for optical imaging and photothermal therapy. Mol. Pharmaceutics 8, 447–456. doi: 10.1021/mp100301t
Zheng, X., Zhou, F., Wu, B., Chen, W. R., and Xing, D. (2012). Enhanced tumor treatment using biofunctional indocyanine green-containing nanostructure by intratumoral or intravenous injection. Mol. Pharm. 9, 514–522. doi: 10.1021/mp200526m
Zhu, L., Li, P., Gao, D., Liu, J., Liu, Y., Sun, C., et al. (2019). pH-sensitive loaded retinal/indocyanine green micelles as an “all-in-one” theranostic agent for multi-modal imaging in vivo guided cellular senescence-photothermal synergistic therapy. Chem. Commun. 55, 6209–6212. doi: 10.1039/C9CC02567G
ZhuGe, D.-L., Wang, L.-F., Chen, R., Li, X.-Z., Huang, Z.-W., Yao, Q., et al. (2019). Cross-linked nanoparticles of silk fibroin with proanthocyanidins as a promising vehicle of indocyanine green for photo-thermal therapy of glioma. Artif. Cells Nanomed. Biotechnol. 47, 4293–4304. doi: 10.1080/21691401.2019.1699819
Keywords: nanoparticles, cancer, indocyanine green (ICG), diagnosis, treatment
Citation: Sevieri M, Silva F, Bonizzi A, Sitia L, Truffi M, Mazzucchelli S and Corsi F (2020) Indocyanine Green Nanoparticles: Are They Compelling for Cancer Treatment? Front. Chem. 8:535. doi: 10.3389/fchem.2020.00535
Received: 08 April 2020; Accepted: 25 May 2020;
Published: 16 July 2020.
Edited by:
Xiaoli Wei, University of California, San Diego, United StatesReviewed by:
Yuanyuan Su, Soochow University, ChinaFeihu Wang, Johns Hopkins University, United States
Copyright © 2020 Sevieri, Silva, Bonizzi, Sitia, Truffi, Mazzucchelli and Corsi. This is an open-access article distributed under the terms of the Creative Commons Attribution License (CC BY). The use, distribution or reproduction in other forums is permitted, provided the original author(s) and the copyright owner(s) are credited and that the original publication in this journal is cited, in accordance with accepted academic practice. No use, distribution or reproduction is permitted which does not comply with these terms.
*Correspondence: Serena Mazzucchelli, c2VyZW5hLm1henp1Y2NoZWxsaSYjeDAwMDQwO3VuaW1pLml0; Fabio Corsi, ZmFiaW8uY29yc2kmI3gwMDA0MDt1bmltaS5pdA==