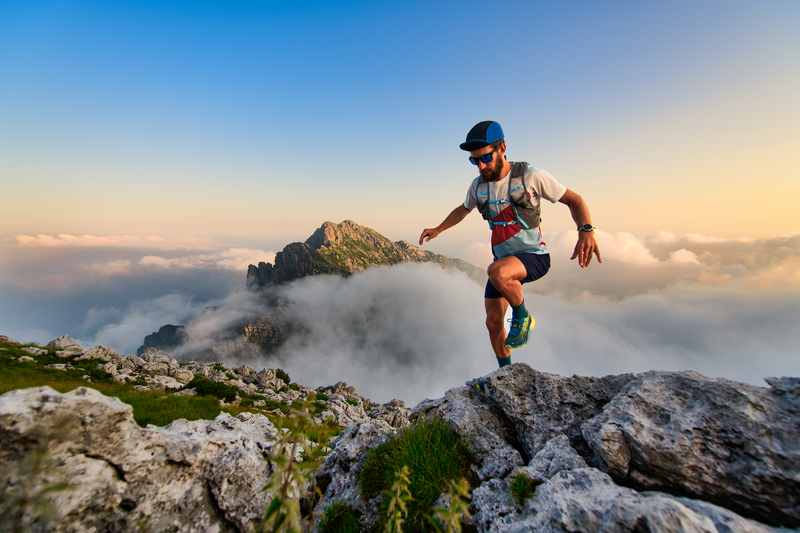
95% of researchers rate our articles as excellent or good
Learn more about the work of our research integrity team to safeguard the quality of each article we publish.
Find out more
ORIGINAL RESEARCH article
Front. Chem. , 10 July 2020
Sec. Green and Sustainable Chemistry
Volume 8 - 2020 | https://doi.org/10.3389/fchem.2020.00528
This article is part of the Research Topic Advanced Membrane Science and Technology for Sustainable Environmental Applications View all 6 articles
Gas separation membranes are essential for the capture, storage, and utilization (CSU) of CO2, especially for H2/CO2separation. However, both glassy and rubbery polymer membranes lead a relatively poor selectivity for H2/CO2 separation because the differences in kinetic diameters of these gases are small. The present study establishing the mixed matrix membranes (MMMs) consist of a nano-sized zeolitic imidazolate frameworks (ZIF-8) blended with the polysulfone (PSf) asymmetric membranes. The gas transport properties (H2, CO2, N2, and CH4) of MMMs with a ZIF-8 loading up to 10 wt% were tested and showing significant improvement on permeance of the light gases (e.g., H2 and CO2). Moreover, the depositional polydopamine (PDA) layer further enhanced the ideal H2/CO2 selectivity, and the PDA-modified MMMs approach the Robeson upper bound of H2/CO2 separation membranes. Hence, the PDA post-modification strategy can effectively repair the defects of MMMs and improved the H2/CO2selectivity.
The continuous rise of atmosphere carbon dioxide (CO2) concentration caused by excessive anthropological combustion of fossil fuels leads to global warming and extreme climate events (Gao et al., 2017). In this situation, capture, storage, and utilization (CSU) of CO2 from other sources have been a worldwide attention (Zhao et al., 2016; Zheng et al., 2016). At present, there are three technically plausible strategies for CO2 CSU: post-combustion CO2 capture (mainly for CO2/N2 separation), oxy-combustion (mainly for O2/N2 separation), and pre-combustion CO2 capture (mainly for H2/CO2 separation; Ramasubramanian et al., 2013; Yan et al., 2015). Among them, pre-combustion CO2 capture is a promising technology, which can reduce the CO2 emission and mitigate energy crisis (Wang et al., 2012; Liao et al., 2015). Since the H2/CO2 syngas from water–gas shift reaction can provide H2 as a preferred fuel or chemical feedstock, it is very important for the separation technologies for H2/CO2.
Membrane separation technology has obtained a great deal of attention for H2/CO2 separation due to the fewer environmental impacts and lower energy costs, compared to conventional industrial methods (e.g., adsorption or cryogenic distillation; Rabiee et al., 2014; Liu et al., 2016; Wang et al., 2017; Ibrahim and Lin, 2018). Besides, membrane separation processes can be employed for the capture of CO2, while H2 is subjected to combustion, which is due to a very high permeation rate of H2 relative to most other gases (Fu et al., 2016). However, polymer membrane performance has been limited by a trade-off between gas permeation and selectivity, known as “Robeson upper bound” (Chua et al., 2011; Zhang et al., 2012; Sánchez Laínez et al., 2018a). Both glassy and rubbery polymer membranes show a relatively poor selectivity for separation of H2/CO2 because the differences in kinetic diameters of these gases are small (2.89 Å for H2 and 3.3 Å for CO2; Hosseini et al., 2010; Kim et al., 2018). The inorganic membranes generally have uniform pore size and excellent resistance to high temperature and pressure, e.g., silica membranes (Song et al., 2016a,b), which can achieved both high permeability and selectivity (Xiang et al., 2017). However, the expensive price limited the large-scale gas application of inorganic membranes. Recent gas separation membranes have been focused on the mixed matrix membranes (MMMs), which compensated the limitations of polymeric and inorganic membranes, while offering an ease in processability and moderate processing cost (Nordin et al., 2015).
Zeolitic imidazolate frameworks (ZIFs) are promising materials for gas separation membrane fabrication, for example, zeolitic imidazolate framework (ZIF-8) as one of the most investigated MOFs with the sod topology and the smaller window of 3.4 Å, which is close to the kinetic diameter of H2 (2.89 Å; Sánchez Laínez et al., 2018b). A highly oriented ZIF-8 membrane on a porous α-alumina support was reported by Bux et al. (2011). The results showed that the H2 permeance of ZIF-8 membrane was ~4,032 Barrer, while the H2/CO2 selectivity was only six. Besides, the pure ZIF-8 membranes are difficult to be reproduced on a large-scale and are too brittle to withstand high operating pressures (Gascon et al., 2012). Recently, the number of studies focused on the utilization of ZIF-8 for MMM preparation, which could potentially overcome the H2/CO2 Robeson upper bound of gas separation membranes (Li et al., 2015). Song et al. (2012) incorporated the ZIF-8 as a nanofiller into a model polymer matrix (Matrimid® 5,218) via a mixing solution, showing enhanced permeability of the MMMs with negligible loss in selectivity. Wijenayake et al. (2013) fabricated a polyimide MMM with 33.3 wt% ZIF-8, and H2 permeability of prepared MMM showed an approximate 400% improvement. Nevertheless, excessive ZIF-8 loadings would increase the chances to agglomerate and increase the defective risks of MMMs.
Developing defect-free ZIF-8/polymer MMMs is a major challenge because the defect leads to the deterioration of the membrane performance (Dechnik et al., 2017). Recently, surface post-treatment could effectively repair defects of MMMs (Nordin et al., 2014). Polydopamine (PDA), which is prepared through dopamine self-polymerization in weak alkaline solutions with the participation of oxygen, forming a PDA coating adhere onto nearly all kinds of substrates, has drawn much attention. (Lu et al., 2017; Yang et al., 2018). This work aims to develop a new type of asymmetric MMMs via phase inversion and PDA modification (Figure 1), further improving the H2/CO2 selectivity. By using our designed strategy, the gas permeance of ZIF-8/PSf MMMs was significantly improved in the presence of a certain amount of ZIF-8 nanoparticles. Furthermore, the effect of polydopamine (PDA)-modified MMMs on the gas transport was studied.
Figure 1. Schematic diagram of preparation of polydopamine (PDA)-modified zeolitic imidazolate frameworks (ZIF-8)/polysulfone (PSf) mixed matrix membranes (MMMs).
PSf (Mn: 22,000 Da) and PDA (98.5%) were purchased from Sigma-Aldrich. Zinc nitrate hexahydrate [Zn(NO3)2•6H2O, 98%], 2-methylimidazole (mIm, 99%), N-N-dimethyl-acetamide (DMAC), tetrahydrofuran (THF), methanol, and ethanol (EtOH) were purchased from Sinopharm Chemical Reagent Co., Ltd. All chemicals were used without further purification.
ZIF-8 nanoparticles were obtained by the solvent method as described in a previous study (Sánchez-Laínez et al., 2016). First, 1.47 g of Zn(NO3)2•6H2O and 3.25 g of mIm were added in 100 ml of MeOH as solutions A and B, respectively. Then, solution A was rapidly poured into solution B under stirring. The mixture solution was reacted for 30 min with continuous stirring at room temperature. Finally, the ZIF-8 nanocrystals were separated from the milky dispersion by centrifugation and then were washed with fresh MeOH three times. The obtained ZIF-8 nanoparticles were directly used for the preparation of MMMs without drying.
Asymmetric PSf membranes were fabricated by a phase separation method as described in the previous study (Ismail et al., 2003), which consist of 22.0 wt% PSf polmyer, 31.8 wt% DMAC, 31.8 wt% THF, and 14.4 wt% EtOH. For asymmetric PSf membranes, the only difference is the evaporation time (30, 45, 60, and 90 s), and the obtained samples were defined as PSf-30, PSf-45, PSf-60, and PSf-90 membranes. For ZIF-8/PSf MMMs, a certain amount (2.5, 5, 7.5, 10, and 15 wt%) of ZIF-8 was added into the mixture solvents under stirring, followed by 30 min of ultrasonication. Then, the PSf polymer was dissolved in a solution mentioned above and kept stirring for 8 h. Next, the dope solution was casted on a glass plate with a 150-μm casting knife (Elcometer3530). The as-prepared MMMs were immersed into a DI-water bath for 24 h after the 30-s evaporation at room temperature. The MMMs were dried for 24 h in ambient atmosphere and defined as 2.5 wt% ZIF-8/PSf-30, 5 wt% ZIF-8/PSf-30, 7.5 wt% ZIF-8/PSf-30, 10 wt% ZIF-8/PSf-30, and 15 wt% ZIF-8/PSf-30. The PDA coating layer could reduce the defects of MMMs. The as-prepared ZIF-8/PSf MMMs were immersed into a PDA Tris buffer solution (2 mg ml−1, 10 mM, and pH = 8.5) with different coating times (0.5, 1, 1.5, 2, 2.5, and 3 h). Then, the PDA-modified MMMs were rinsed with DI-water and dried in a vacuum oven for 12 h at room temperature.
An XRD diffractometer (Bruker D8, Germany) was used to detect the crystal structure of the ZIF-8 at 2θ = 5°-40° with 0.02 step size. The infrared spectral analysis of the ZIF-8 nanoparticles and MMMs were tested by attenuated total reflectance-Fourier transform infrared spectroscopy (ATR-FTIR; Vertex 70; Bruker). The morphology and structure of the samples were observed by scanning electron microscopy (Nova NanoSEM450, USA). The N2 adsorption and desorption isotherms of the ZIF-8 nanoparticles were observed by 3Flex physical adsorption instrument (Micromeritics, USA) at 77 K, and the adsorption isotherms of H2 and CO2 were tested at 273 K. A thermogravimetric analyzer (TGAQ50; TA Instruments-Waters LLC) was used for evaluating the thermal stability of the ZIF-8 nanoparticles and MMMs. All samples were heated from 30 to 800°C with 10°C min−1 heating rate under N2 with a flow of 50-ml min−1.
Single-gas permeability of membranes was measured using a constant-volume variable-pressure method (Zhao et al., 2018). The entire permeation cell was placed in an oven to keep the temperature at 30°C. The permeation cell was kept under vacuum for 12 h to remove other gases. The effective area of membranes is about 0.3 cm2, and the gas permeability of each samples has been tested at least three times. The gas permeation (in terms of Barrer, 1 Barrer = 1 × 10−10 cm3 (STP) cm cm−2 s−1 cmHg−1) was calculated by the following Equation (1):
where, P is the gas permeability in Barrer, V is the constant volume container (cm3), l is the thickness of dense layer of membrane (cm), which are obtained from SEM images. A is the membrane surface area (cm2), T is the temperature (K), P0 is the upstream (feed) pressure (psia), and dp/dt is the change in pressure against time (mmHg/s). The gas ideal selectivity (αi/j) for components i and j was defined as the ratio of gas permeability of the two components by the following Equation (2):
The ZIF-8 nanoparticles were synthesized by the solvent method, and the XRD characteristic peaks of ZIF-8 are shown in Figure 2A, comfirming the typical sodalite (SOD) type structure of ZIF-8 nanoparticles (Park et al., 2006; Cravillon et al., 2009). Figure 2B shows the resembling spherical morphology of ZIF-8 nanoparticles by SEM characterization, and the average size of ZIF-8 nanoparticles was about 150 nm. The microporous structure of ZIF-8 was confirmed by N2 adsorption and desorption isotherms, and the ZIF-8 nanoparticles exhibited a typical Type-I isotherm, as shown in Figure 2C. The BET surface area and pore volume of ZIF-8 nanoparticles were 1,371 m2 g−1 and 0.72 cm3 g−1, respectively. The pore size distribution (PSD) provided further insight into the pore structure of ZIF-8 nanoparticles (Figure 2D). The 0.64 and 0.75 nm PSD centers of ZIF-8 represented the flexible six-membered ring, which arises from the vibrations of imidazole ligands (Guo et al., 2018). In addition, the largest PSD center of ZIF-8 was about 1.0 nm, which corresponds to the diameter of the ZIF-8 SOD cage.
Figure 2. (A) XRD pattern of ZIF-8 nanoparticles; (B) SEM image of ZIF-8 nanoparticles; (C) N2 adsorption–desorption isotherms; and (D) pore size distribution of the ZIF-8 nanoparticles.
To optimize the preparation process of asymmetric PSf membranes, the effect of evaporation for gas separation performance of membranes was investigated as shown in Table 1. It can be found that the H2 and CO2 permeabilities significantly were reduced with the increasing evaporation time. This is due to the evaporation process that induced the skin layer formed of PSf membranes, and the skin layer thickness was improved with increasing evaporation times as shown in Figure 3. The formed skin layer is a resistance barrier between the PSf membrane and the coagulation bath. Presence of this resistance barrier induced the densification of skin layer as the evaporation time (Hołda et al., 2013). Moreover, all asymmetric PSf membranes exhibited lower N2 and CH4 gas permeabilities than the other gases, owning to their larger kinetic diameters. The cross-section morphology of asymmetric PSf membranes with different evaporation times is shown in Figure 3, which consist of the extremely well-defined dense skin layers supported on a highly open-celled structure. Based on the gas separation test results, the PSf-30 membrane was used for the ZIF-8/PSf MMM preparation.
Table 1. Gas permeability and selectivity of asymmetric polysulfone (PSf) membranes with different evaporation time at 4 bar and 30°C.
Figure 3. Cross-section SEM images of the asymmetric polysulfone (PSf) membranes with different evaporation times. (a) 30 s; (b) 45 s; (c) 60 s; and (d) 90 s.
Table 2 summarizes the effects of the ZIF-8 gas loading on the gas separation performance of MMMs. Compared with the asymmetric PSf-30 membrane, it was confirmed that permeability of MMMs for the four gases was improved with increasing ZIF-8 loading below 10 wt.%. However, an excessive amount of ZIF-8 can cause the separation performance to decline in the case of the 15 wt% ZIF-8/PSf-30 MMM. Figure 4 shows the cross-section and top surface of the five different MMMs. The surface defect was significantly increased due to the incorporation of ZIF-8 with different loadings. The defects on the surface of MMMs could be attributed to the ZIF-8 agglomeration (Nafisi and Hägg, 2014; Boroglu and Yumru, 2017). Owing to these defects of surfaces, the gas selectivity of MMMs did not change significantly; only the improvement of H2/N2 selectivity was found. In addition, the thickness of sublayers under the dense skin layers was improved (Figure 4) with the increasing ZIF-8 loading. This morphological change in the MMMs was attributed to the delayed demixing during the phase separation, leading to the dense skin layer transformation to the porous layer (Lu et al., 2016).
Table 2. Gas permeation of zeolitic imidazolate frameworks (ZIF-8)/polysulfone (PSf)-30 mixed matrix membranes (MMMs) with different ZIF-8 loading at 4 bar and 30°C.
Figure 4. Cross-section and surface of SEM images of ZIF-8/PSf-30 MMMs with different ZIF-8 loadings. (a) 2.5 wt%; (b) 5 wt%; (c) 7.5 wt%; (d) 10 wt%; and (e) 15 wt%.
The PDA modified method can overcome the limitations caused by the traditional self-assembly, entrapment, and chemical binding methods (Lu et al., 2017; Wang et al., 2019). After the PDA modification, the stability of MMMs could be improved, and the defects of surfaces will be repaired (Liu et al., 2013; Huang et al., 2014; Yuan et al., 2014). In order to further study the gas separation of MMMs after PDA coating with different time, the H2 and CO2 gases were selected as the representatives. Figure 5A showed the H2 and CO2 permeabilities and H2/CO2 selectivity of 10 wt% ZIF-8/PSf-30 MMM with the different PDA coating time. Either H2 or CO2 permeability follows a decreasing trend when increasing PDA coating times. This is because the improved denser layers of MMMs and the enhanced gas transport resistance. However, for smaller kinetic diameter gas such as H2, the permeability inhibition was not obvious than that of CO2. Hence, the H2/CO2 selectivity of MMMs was improved, such as the selectivity of PDA-2/10 wt% ZIF-8/PSf-30 MMM achieved 9.3 at 4 bar and 30°C. Figure 5B showed the H2 and CO2 permeabilities and H2/CO2 selectivity of PDA-2/10 wt% ZIF-8/PSf-30 MMM as a function of feed pressure. H2 and CO2 permeabilities of PDA-2/10 wt% ZIF-8/PSf-30 MMM change slightly with increasing feed pressure. However, the H2/CO2 selectivity decreased significantly when the pressure increases, which is due to the CO2 permeance improvement. This can be attributed to enhanced CO2 solution into the PDA coating layer at high pressures (Huang et al., 2014; Wang et al., 2015).
Figure 5. (a) Effect of different PDA coating time of the 10 wt% ZIF-8/PSf-30 MMM on H2/CO2 separation at 30°C under 4 bar; (b) Effect of feed pressures on the H2/CO2 separation of PDA-2/10 wt% ZIF-8/PSf-30 MMM at 30°C; (c) attenuated total reflectance-Fourier transform infrared spectroscopy (ATR-FTIR) spectra; and (d) TG curves of the ZIF-8, PSf-30 membranes, 10 wt% ZIF-8/PSf-30, and PDA-2/10 wt% ZIF-8/PSf-30 MMM.
In addition, the surface property of PDA-2/10 wt% ZIF-8/PSf-30 MMM was investigated by ATR-FTIR as shown in Figure 5C. Compared with the 10-wt% ZIF-8/PSf-30 MMM, a different peak at 1,540 cm−1 was attributed to the N–H bending vibration of PDA (Habibi et al., 2015; Zhou et al., 2015), which proved the successful PDA modified layers. However, the TG curves showed good thermal stability of MMMs as shown in Figure 5D. The surface and cross-section SEM images of the 10-wt% ZIF-8/PSf-30 with different coating times are shown in Figure 6. The SEM images were taken to investigate the changes in surface morphology of MMMs with different coating times. With increasing PDA coating time, it can be observed that the defects in the surface almost disappeared, and the little rough surface becomes quite smooth.
Figure 6. Surface and cross-section of the 10 wt% ZIF-8/PSf-30 MMMs with different PDA coating times. (a) 1 h; (b) 2 h; and (c) 3 h.
The correlation between selectivity and permeability for H2/CO2 is shown in Figure 7. Embedding ZIF-8 nanoparticles into the PSf-30 asymmetric membrane had a positive effect on the H2 permeability. However, the selectivity of H2/CO2 was not improved. After the PDA coating, the H2/CO2 selectivity was significantly improved, while the H2 permeability was reduced, and the result of PDA-2/10 wt% ZIF-8/PSf-30 MMMs was very close to the 2008 Roberson upper bound. Compared to the reported MMMs, the PDA-modified MMMs showed higher selectivity of H2/CO2 as shown in Table 3.
Figure 7. Selectivity of H2/CO2 vs. H2 permeability for the prepared MMMs at 30°C under four bar in the presence of 2008 Robeson upper bound.
The novel MMMs have been developed for gas separation application by the PDA post-modified strategy. The ZIF-8 nanoparticles have been embedded in the PSf asymmetric membranes as the MMMs, and the gas permeability of MMMs was significantly improved. The optimal ZIF-8 concentration of 10 wt% produced an H2 permeability of 87 Barrer, but the H2/CO2 selectivity was only 2.38. The PDA modification has been considered as an effective method for improving the properties of membranes. This binding method can overcome the limitations caused by the traditional self-assembly, entrapment, and chemical binding methods (Lu et al., 2017, 2019). Coating the MMMs with PDA repaired most defects of the surfaces, which reduced the H2 permeability of MMMs and improved the H2/CO2 selectivity. For PDA-2/10 wt% ZIF-8/PSf-30 MMM, the H2 permeability was 23.3 Barrer and the H2/CO2 selectivity achieved 9.3 at 30°C under 4 bar. Prepared PDA-modified MMMs were highly promising for H2/CO2 separation, owing to the simple manufacturing process and effective improvement. These results demonstrated the availability of the PDA post-modified MMMs for gas separation application.
All datasets presented in this study are included in the article/ supplementary material.
XM designed, analyzed data, and wrote the main manuscript. SY performed the experiments and performance evaluation. PL conceptualized, designed, and edited the main manuscript. YZ and JZ helped design complement experiments and reviewed and edited the manuscript. All authors contributed to the review and approval of the manuscript.
This work is financially supported by the Zhejiang Provincial Natural Science Foundation (LQ19E080005), the Ningbo Natural Science Foundation (2019A610140, 2018A610285), and Ningbo Municipal Bureau of Science and Technology (2019B10096).
The authors declare that the research was conducted in the absence of any commercial or financial relationships that could be construed as a potential conflict of interest.
Boroglu, M. S., and Yumru, A. B. (2017). Gas separation performance of 6FDA-DAM-ZIF-11 mixed-matrix membranes for H2/CH4 and CO2/CH4 separation. Sep. Purif. Technol. 173, 269–279. doi: 10.1016/j.seppur.2016.09.037
Bux, H., Feldhoff, A., Cravillon, J., Wiebcke, M., Li, Y., and Caro, J. (2011). Oriented zeolitic imidazolate framework-8 membrane with sharp H2/C3 H8 molecular sieve separation. Chem. Mater. 23, 2262–2269. doi: 10.1021/cm200555s
Chua, M. L., Shao, L., Low, B. T., Xiao, Y. C., and Chung, T. (2011). Polyetheramine-polyhedral oligomeric silsesquioxane organic-inorganic hybrid membranes for CO2/H2 and CO2/N2 separation. J. Membr. Sci. 385, 40–48. doi: 10.1016/j.memsci.2011.09.008
Cravillon, J., MÃnzer, S., Lohmeier, S., Feldhoff, A., Huber, K., and Wiebcke, M. (2009). Rapid room-temperature synthesis and characterization of nanocrystals of a prototypical zeolitic imidazolate framework. Chem. Mater. 21, 1410–1412. doi: 10.1021/cm900166h
Dechnik, J., Gascon, J., Doonan, C. J., Janiak, C., and Sumby, C. J. (2017). Mixed-matrix membranes. Angew. Chem. Int. Ed. 56, 9292–9310. doi: 10.1002/anie.201701109
Fu, J. R., Das, S., Xing, G. L., Ben, T., Valtchev, V., and Qiu, S. L. (2016). Fabrication of COF-MOF composite membranes and their highly selective separation of H2/CO2. J. Am. Chem. Soc. 138, 7673–7680. doi: 10.1021/jacs.6b03348
Gao, W. L., Zhou, T. T., Gao, Y. S., Louis, B., O'Hare, D., and Wang, Q. (2017). Molten salts-modified MgO-based adsorbents for intermediate-temperature CO2 capture: a review. J. Energy. Chem. 26, 830–838. doi: 10.1016/j.jechem.2017.06.005
Gascon, J., Kapteijn, F., Zornoza, B., Sebastian, V., Casado, C., and Coronas, J. (2012). Practical approach to zeolitic membranes and coatings: state of the art, opportunities, barriers, and future perspectives. Chem. Mater. 24, 2829–2844. doi: 10.1021/cm301435j
Guo, A., Ban, Y. J., Yang, K., and Yang, W. S. (2018). Metal-organic framework-based mixed matrix membranes: synergetic effect of adsorption and diffusion for CO2/CH4 separation. J. Membr. Sci. 562, 76–84. doi: 10.1016/j.memsci.2018.05.032
Habibi, S., Nematollahzadeh, A., and Mousavi, S. A. (2015). Nano-scale modification of polysulfone membrane matrix and the surface for the separation of chromium ions from water. Chem. Eng. J. 267, 306–316. doi: 10.1016/j.cej.2015.01.047
Hołda, A. K., Aernouts, B., Saeys, W., and Vankelecom, I. F. (2013). Study of polymer concentration and evaporation time as phase inversion parameters for polysulfone-based SRNF membranes. J. Membr. Sci. 442, 196–205. doi: 10.1016/j.memsci.2013.04.017
Hosseini, S. S., Peng, N., and Chung, T. S. (2010). Gas separation membranes developed through integration of polymer blending and dual-layer hollow fiber spinning process for hydrogen and natural gas enrichments. J. Membr. Sci. 349, 156–166. doi: 10.1016/j.memsci.2009.11.043
Huang, A. S., Liu, Q., Wang, N. Y., and Caro, J. (2014). Highly hydrogen permselective ZIF-8 membranes supported on polydopamine functionalized macroporous stainless-steel-nets. J. Mater. Chem. A. 2, 8246–8251. doi: 10.1039/C4TA00299G
Ibrahim, A. F., and Lin, Y. S. (2018). Synthesis of graphene oxide membranes on polyester substrate by spray coating for gas separation. Chem. Eng. Sci. 190, 312–319. doi: 10.1016/j.ces.2018.06.031
Ismail, A. F., Ng, B. C., and Rahman, W. A. (2003). Effects of shear rate and forced convection residence time on asymmetric polysulfone membranes structure and gas separation performance. Sep. Purif. Technol. 33, 255–272. doi: 10.1016/S1383-5866(03)00009-1
Kim, S., Shamsaei, E., Lin, X. C., Hu, Y. X., Simon, G. P., and Seong, J. G., et al. (2018). The enhanced hydrogen separation performance of mixed matrix membranes by incorporation of two-dimensional ZIF-L into polyimide containing hydroxyl group. J. Membr. Sci. 549, 260–266. doi: 10.1016/j.memsci.2017.12.022
Li, X. Q., Cheng, Y. D., Zhang, H. Y., Wang, S. F., Jiang, Z. Y., and Guo, R. L., et al. (2015). Efficient CO2 capture by functionalized graphene oxide nanosheets as fillers to fabricate multi-permselective mixed matrix membranes. ACS Appl. Mater. Inter. 7, 5528–5537. doi: 10.1021/acsami.5b00106
Liao, J. Y., Wang, Z., Gao, C. Y., Wang, M., Yan, K., and Xie, X. M., et al. (2015). A high performance PVAm-HT membrane containing high-speed facilitated transport channels for CO2 separation. J. Mater. Chem. A 3, 16746–16761. doi: 10.1039/C5TA03238E
Liu, G. P., Jin, W. Q., and Xu, N. P. (2016). Two-dimensional-material membranes: a new family of high-performance separation membranes. Angew. Chem. Int. Ed. 55, 13384–13397. doi: 10.1002/anie.201600438
Liu, Q., Wang, N. Y., Caro, J., and Huang, A. S. (2013). Bio-inspired polydopamine: a versatile and powerful platform for covalent synthesis of molecular sieve membranes. J. Am. Chem. Soc. 135, 17679–17682. doi: 10.1021/ja4080562
Lu, P., Li, W. J., Yang, S., Liu, Y., Wang, Q., and Li, Y. S. (2019). Layered double hydroxide-modified thin-film composite membranes with remarkably enhanced chlorine resistance and anti-fouling capacity. Sep. Purif. Technol. 220, 231–237. doi: 10.1016/j.seppur.2019.03.039
Lu, P., Liang, S., Qiu, L., Gao, Y. S., and Wang, Q. (2016). Thin film nanocomposite forward osmosis membranes based on layered double hydroxide nanoparticles blended substrates. J. Membr. Sci. 504, 196–205. doi: 10.1016/j.memsci.2015.12.066
Lu, P., Liang, S., Zhou, T. T., Xue, T. S., Mei, X. Y., and Wang, Q. (2017). Layered double hydroxide nanoparticle modified forward osmosis membranes via polydopamine immobilization with significantly enhanced chlorine and fouling resistance. Desalination 421, 99–109. doi: 10.1016/j.desal.2017.04.030
Nafisi, V., and Hägg, M. (2014). Development of dual layer of ZIF-8/PEBAX-2533 mixed matrix membrane for CO2 capture. J. Membr. Sci. 459, 244–255. doi: 10.1016/j.memsci.2014.02.002
Nordin, N. A. H. M., Ismail, A. F., Mustafa, A., Murali, R. S., and Matsuura, T. (2014). The impact of ZIF-8 particle size and heat treatment on CO2/CH4 separation using asymmetric mixed matrix membrane. RSC. Adv. 4, 52530–52541. doi: 10.1039/C4RA08460H
Nordin, N. A. H. M., Ismail, A. F., Mustafa, A., Murali, R. S., and Matsuura, T. (2015). Utilizing low ZIF-8 loading for an asymmetric PSf/ZIF-8 mixed matrix membrane for CO2/CH4 separation. RSC. Adv. 5, 30206–30215. doi: 10.1039/C5RA00567A
Ordoñez, M. J. C., Balkus, K. J., Ferraris, J. P., and Musselman, I. H. (2010). Molecular sieving realized with ZIF-8/Matrimid® mixed-matrix membranes. J. Membr. Sci. 361, 28–37. doi: 10.1016/j.memsci.2010.06.017
Pakizeh, M., Moghadam, A. N., Omidkhah, M. R., and Namvar-Mahboub, M. (2013). Preparation and characterization of dimethyldichlorosilane modified SiO2/PSf nanocomposite membrane. Korean J. Chem. Eng. 30, 751–760. doi: 10.1007/s11814-012-0186-x
Park, K. S., Ni, Z., Cote, A. P., Choi, J. Y., Huang, R., and Uribe-Romo, F. J., et al. (2006). Exceptional chemical and thermal stability of zeolitic imidazolate frameworks. Proc. Natl. Acad. Sci. 103, 10186–10191. doi: 10.1073/pnas.0602439103
Rabiee, H., Soltanieh, M., Mousavi, S. A., and Ghadimi, A. (2014). Improvement in CO2/H2 separation by fabrication of poly (ether-b-amide6)/glycerol triacetate gel membranes. J. Membr. Sci. 469, 43–58. doi: 10.1016/j.memsci.2014.06.026
Ramasubramanian, K., Zhao, Y. N., and Winston Ho, W. S. (2013). CO2 capture and H2 purification: Prospects for CO2-selective membrane processes. AIChE J. 59, 1033–1045. doi: 10.1002/aic.14078
Sánchez Laínez, J., Friebe, S., Zornoza, B., Mundstock, A., Strauß, I., and Téllez, C., et al. (2018a). Polymer-stabilized percolation membranes based on nanosized zeolitic imidazolate frameworks for H2/CO2 separation. Chem. Nano. Mat. 4, 698–703. doi: 10.1002/cnma.201800126
Sánchez Laínez, J., Paseta, L., Navarro, M., Zornoza, B., Téllez, C., and Coronas, J. (2018b). Ultrapermeable thin film ZIF-8/Polyamide membrane for H2/CO2 separation at high temperature without using sweep gas. Adv. Mater. Interfaces 5:1800647. doi: 10.1002/admi.201800647
Sánchez-Laínez, J., Zornoza, B., Friebe, S., Caro, J., Cao, S., and Sabetghadam, A., et al. (2016). Influence of ZIF-8 particle size in the performance of polybenzimidazole mixed matrix membranes for pre-combustion CO2 capture and its validation through interlaboratory test. J. Membr. Sci. 515, 45–53. doi: 10.1016/j.memsci.2016.05.039
Song, H. T., Zhao, S. F., Chen, J. W., and Qi, H. (2016a). Hydrothermally stable Zr-doped organosilica membranes for H2/CO2 separation. Micropor. Mesopor. Mat. 224, 277–284. doi: 10.1016/j.micromeso.2016.01.001
Song, H. T., Zhao, S. F., Lei, J. J., Wang, C. Y., and Qi, H. (2016b). Pd-doped organosilica membrane with enhanced gas permeability and hydrothermal stability for gas separation. J. Mater. Sci. 51, 6275–6286. doi: 10.1007/s10853-016-9924-5
Song, Q. L., Nataraj, S. K., Roussenova, M. V., Tan, J. C., Hughes, D. J., and Li, W., et al. (2012). Zeolitic imidazolate framework (ZIF-8) based polymer nanocomposite membranes for gas separation. Energ. Environ. Sci. 5, 8359–8369. doi: 10.1039/c2ee21996d
Sorribas, S., Zornoza, B., Téllez, C., and Coronas, J. (2014). Mixed matrix membranes comprising silica-(ZIF-8) core-shell spheres with ordered meso–microporosity for natural- and bio-gas upgrading. J. Membr. Sci. 452, 184–192. doi: 10.1016/j.memsci.2013.10.043
Wang, M. H., Zhao, J. J., Wang, X. X., Liu, A. D., and Gleason, K. K. (2017). Recent progress on submicron gas-selective polymeric membranes. J. Mater. Chem. A 5, 8860–8886. doi: 10.1039/C7TA01862B
Wang, N. Y., Liu, Y., Qiao, Z. W., Diestel, L., Zhou, J., and Huang, A. S., et al. (2015). Polydopamine-based synthesis of a zeolite imidazolate framework ZIF-100 membrane with high H2/CO2 selectivity. J. Mater. Chem. A 3, 4722–4728. doi: 10.1039/C4TA06763K
Wang, Q., Tay, H. H., Zhong, Z. Y., Luo, J. Z., and Borgna, A. (2012). Synthesis of high-temperature CO2 adsorbents from organo-layered double hydroxides with markedly improved CO2 capture capacity. Energ. Environ. Sci. 5, 7526–7530. doi: 10.1039/c2ee21409a
Wang, Y., Li, X. Y., Zhao, S. F., Fang, Z. D., Ng, D., and Xie, C. X., et al. (2019). Thin-Film composite membrane with interlayer decorated Metal-Organic Framework UiO-66 toward enhanced forward osmosis performance. Ind. Eng. Chem. Res. 58, 195–206. doi: 10.1021/acs.iecr.8b04968
Wijenayake, S. N., Panapitiya, N. P., Versteeg, S. H., Nguyen, C. N., Goel, S., and Balkus Jr, K. J., et al. (2013). Surface cross-linking of ZIF-8/polyimide mixed matrix membranes (MMMs) for gas separation. Ind. Eng. Chem. Res. 52, 6991–7001. doi: 10.1021/ie400149e
Xiang, L., Sheng, L. Q., Wang, C. Q., Zhang, L. X., Pan, Y. C., and Li, Y. S. (2017). Amino-functionalized ZIF-7 nanocrystals: improved intrinsic separation ability and interfacial compatibility in mixed-matrix membranes for CO2/CH4 separation. Adv. Mater. 29:1606999. doi: 10.1002/adma.201606999
Yan, S. P., Zhao, S. F., Wardhaugh, L., and Feron, P. H. (2015). Innovative use of membrane contactor as condenser for heat recovery in carbon capture. Environ. Sci. Technol. 49, 2532–2540. doi: 10.1021/es504526s
Yang, H. C., Waldman, R. Z., Wu, M. B., Hou, J. W., Chen, L., and Darling, S. B., et al. (2018). Dopamine: just the right medicine for membranes. Adv. Funct. Mater. 28:1705327. doi: 10.1002/adfm.201705327
Yang, T. X., and Chung, T. (2013). High performance ZIF-8/PBI nano-composite membranes for high temperature hydrogen separation consisting of carbon monoxide and water vapor. Int. J. Hydrogen Energ. 38, 229–239. doi: 10.1016/j.ijhydene.2012.10.045
Yang, T. X., Shi, G. M., and Chung, T. (2012). Symmetric and asymmetric zeolitic imidazolate frameworks (ZIFs)polybenzimidazole (PBI) nanocomposite membranes for hydrogen purification at high temperatures. Adv. Energy. Mater. 2, 1358–1367. doi: 10.1002/aenm.201200200
Yuan, C. F., Liu, Q., Chen, H. F., and Huang, A. S. (2014). Mussel-inspired polydopamine modification of supports for the facile synthesis of zeolite LTA molecular sieve membranes. RSC. Adv. 4, 41982–41988. doi: 10.1039/C4RA05400H
Zhang, L. L., Hu, Z. Q., and Jiang, J. W. (2012). Metal-organic framework/polymer mixed-matrix membranes for H2/CO2 separation: A fully atomistic simulation study. J. Phys. Chem. C. 116, 19268–19277. doi: 10.1021/jp3067124
Zhao, S. F., Feron, P. H., Deng, L. Y., Favre, E., Chabanon, E., and Yan, S. P., et al. (2016). Status and progress of membrane contactors in post-combustion carbon capture: A state-of-the-art review of new developments. J. Membr. Sci. 511, 180–206. doi: 10.1016/j.memsci.2016.03.051
Zhao, S. S., Liao, J. Y., Li, D. F., Wang, X. D., and Li, N. W. (2018). Blending of compatible polymer of intrinsic microporosity (PIM-1) with Tröger's Base polymer for gas separation membranes. J. Membr. Sci. 566, 77–86. doi: 10.1016/j.memsci.2018.08.010
Zheng, Q. W., Huang, L., Zhang, Y., Wang, J. Y., Zhao, C., and Zhang, Q., et al. (2016). Unexpected highly reversible topotactic CO2 sorption/desorption capacity for potassium dititanate. J. Mater. Chem. A 4, 12889–12896. doi: 10.1039/C6TA04117E
Keywords: polysulfone, mixed matrix membranes, ZIF-8 nanoparticles, polydopamine, H2/CO2separation
Citation: Mei X, Yang S, Lu P, Zhang Y and Zhang J (2020) Improving the Selectivity of ZIF-8/Polysulfone-Mixed Matrix Membranes by Polydopamine Modification for H2/CO2 Separation. Front. Chem. 8:528. doi: 10.3389/fchem.2020.00528
Received: 14 April 2020; Accepted: 22 May 2020;
Published: 10 July 2020.
Edited by:
Liguo Shen, Zhejiang Normal University, ChinaReviewed by:
Neil Zhao, Deakin University, AustraliaCopyright © 2020 Mei, Yang, Lu, Zhang and Zhang. This is an open-access article distributed under the terms of the Creative Commons Attribution License (CC BY). The use, distribution or reproduction in other forums is permitted, provided the original author(s) and the copyright owner(s) are credited and that the original publication in this journal is cited, in accordance with accepted academic practice. No use, distribution or reproduction is permitted which does not comply with these terms.
*Correspondence: Peng Lu, bHVwZW5nQG5idS5lZHUuY24=; Jian Zhang, anpoYW5nQG5pbXRlLmFjLmNu
Disclaimer: All claims expressed in this article are solely those of the authors and do not necessarily represent those of their affiliated organizations, or those of the publisher, the editors and the reviewers. Any product that may be evaluated in this article or claim that may be made by its manufacturer is not guaranteed or endorsed by the publisher.
Research integrity at Frontiers
Learn more about the work of our research integrity team to safeguard the quality of each article we publish.