- 1Univ Lyon, Université Claude Bernard Lyon 1, CNRS, IRCELYON, Villeurbanne, France
- 2LCIO, Laboratoire de Chimie de Coordination Inorganique et Organométallique, Université Libanaise- Faculté des Sciences I, Beyrouth, Lebanon
Catalysts based on molybdenum carbide or nitride nanoparticles (2–5 nm) supported on titania were prepared by wet impregnation followed by a thermal treatment under alkane (methane or ethane)/hydrogen or nitrogen/hydrogen mixture, respectively. The samples were characterized by elemental analysis, volumetric adsorption of nitrogen, X-ray diffraction, and aberration-corrected transmission electron microscopy. They were evaluated for the hydrogenation of CO2 in the 2–3 MPa and 200–300°C ranges using a gas-phase flow fixed bed reactor. CO, methane, methanol, and ethane (in fraction-decreasing order) were formed on carbides, whereas CO, methanol, and methane were formed on nitrides. The carbide and nitride phase stoichiometries were tuned by varying the preparation conditions, leading to C/Mo and N/Mo atomic ratios of 0.2–1.8 and 0.5–0.7, respectively. The carbide activity increased for lower carburizing alkane concentration and temperature, i.e., lower C/Mo ratio. Enhanced carbide performances were obtained with pure anatase titania support as compared to P25 (anatase/rutile) titania or zirconia, with a methanol selectivity up to 11% at 250°C. The nitride catalysts appeared less active but reached a methanol selectivity of 16% at 250°C.
Introduction
The use of carbon dioxide (CO2) in current industrial processes is limited to synthesis of urea and its derivatives, carbonates, and salicylic acid, and in the production of methanol from natural gas or coal (Mikkelsen et al., 2010; Wang et al., 2011). With the development of carbon capture technologies, CO2 is considered as an attractive renewable resource. By using it as raw material for the synthesis of chemicals, waste CO2 might turn into a valuable feedstock (Sakakura et al., 2007). The catalytic hydrogenation of CO2 as a C1 building block is one of the promising routes currently investigated (Saeidi et al., 2014).
The catalytic conversion of CO2 to CO can occur via reverse water-gas shift (RWGS) reaction. CO is a valuable precursor molecule that can be used for methanol synthesis and for the production of longer-chain hydrocarbons via the Fischer-Tropsch process (Khodakov et al., 2007; Kattel et al., 2017). Today, most of the methanol is synthesized from syngas, i.e., through CO hydrogenation (Olah et al., 2009). An alternative feedstock is CO2, where methanol can be produced either via CO2 conversion to CO (RWGS) and subsequent hydrogenation, or by the direct hydrogenation of CO2 (Wang et al., 2011; Saeidi et al., 2014). Methanol is used in chemical industries, for the synthesis of formaldehyde and acetic acid, the production of dimethyl ether (used as a fuel), or as a solvent (Olah et al., 2009; Alvarado, 2016). Alternatively, the hydrogenation of CO2 to methane is also an important catalytic process for CO2 valorization (Wei and Jinlong, 2011; Stangeland et al., 2017). Indeed, the methanation (Sabatier's) reaction has gained increasing attention through the “power-to-gas” approach used for energy storage. Throughout this concept, renewable energies such as wind and solar radiation are used to power water electrolysis, generating H2. Subsequently, H2 is combined with CO2 to produce CH4. Methane can be stored in classical natural gas infrastructures and be used on demand (Schiebahn et al., 2015).
Tailoring the selectivity to these products depends on both the reaction conditions and the catalyst nature. For example, higher reaction temperatures can lower the selectivity toward methanol that is produced via exothermic reaction, while favoring the selectivity to CO which is produced via the endothermic RWGS reaction (Saeidi et al., 2014). Copper-based catalysts, such as Cu-Ni/Al2O3, exhibit high selectivity to CO (Liu and Liu, 1999; Wang et al., 2011), and nickel catalysts supported on oxides are the most widely studied materials for methanation (Wei and Jinlong, 2011). Cu/ZnO/Al2O3 is currently the commercial catalyst for methanol production from H2/CO/CO2 (Ott et al., 2012). However, these processes still face challenges; for example, one of the major problems with Ni-based catalysts is their deactivation due to carbon deposition and sintering of nickel (Wei and Jinlong, 2011). Furthermore, in methanol synthesis, relatively harsh reaction conditions (220–300°C and 50–100 bar) are used, leading to thermal degradation and sintering of active metal as well as extensive consumption of H2 (Ott et al., 2012). Besides Cu or Ni, noble metal-based catalysts are reported to be active and stable in CO2 hydrogenation (Kattel et al., 2017). Nevertheless, their high price and scarcity hinder their use.
Recently, transition metal carbides have attracted attention as promising catalysts for the conversion of CO2 into CO, methanol, methane, and other hydrocarbons (Nagai et al., 1998b; Solymosi et al., 2002; Porosoff et al., 2014; Posada-Pérez et al., 2014, 2016a,b; Xu et al., 2014; Chen et al., 2015, 2016; Gao et al., 2016; Liu et al., 2016; Han et al., 2019; Reddy et al., 2019). It has been reported through computational work that transition metal (e.g., Ti, Mo, and V) carbide surfaces are able to uptake and activate CO2 (Posada-Pérez et al., 2014, 2015; Kunkel et al., 2016, 2018, 2019; Liu et al., 2017). In liquid phase, metals (Pd, Cu, Co, Fe) supported on Mo2C have been tested for the hydrogenation of CO2 in dioxane under 40 bars, at 135–200°C (Chen et al., 2015, 2016). Pd and Cu promote the formation of methanol while Co and Fe favor ethanol and C2+ hydrocarbons (e.g., C2H4, C2H6). The hydrogenation of CO2 in gas phase has been evaluated over unsupported cubic MoC [particles Xu et al., 2014 or nanowires Gao et al., 2016], hexagonal Mo2C [particles Liu et al., 2017; Reddy et al., 2019 or nanowires Gao et al., 2016], Mo2C coated with nitrogen and sulfur-codoped carbon (Han et al., 2019), and metals (Au, Cu, Co, Ni) supported on molybdenum carbide (Xu et al., 2015; Posada-Pérez et al., 2016b) or titanium carbide (Rodriguez et al., 2013). The addition of a metal increases the activity and affects the selectivity; e.g., Ni, Co, and Cu favor the formation of methane, hydrocarbons, and methanol, respectively (Xu et al., 2015).
Supported molybdenum carbides have been rarely evaluated for the hydrogenation of CO2. At atmospheric pressure and 300°C, Mo2C/Al2O3 (Nagai et al., 1998b) promotes the RWGS reaction. At 220°C and 30 bar, Mo2C/MCM-41 exhibits high selectivity to CH4 (70–95%), along with CO (0–20%) and methanol (2–27%) at 4 to 10% conversion (Liu et al., 2016). Increasing the Mo2C loading from 10 to 40% over MCM-41 increases the selectivity to CO at the expense of CH4 and methanol. At 300°C, MoxCy/SiO2 generates exclusively CO, CH4, and H2O at atmospheric pressure, while methanol and hydrocarbons (C2H6, C3H8) are also obtained at high pressure (20 bars) (García Blanco et al., 2019).
Molybdenum nitride catalysts have been successfully used to promote ammonia synthesis (Hargreaves, 2014), hydrodesulfurization [e.g., thiophene Gong et al., 2005], hydrodenitrogenation [e.g., carbazole Nagai et al., 1998a], and hydrogenation [e.g., p-chloronitrobenzene Perret et al., 2012] reactions. Although they can exhibit similar activity as carbides (Alexander and Hargreaves, 2010), to date only one study reports the use of a nitride for the hydrogenation of CO2 (Yao et al., 2019). γ-Mo2N is active for the production of CO with selectivity above 98%. The addition of Co increases the conversion in the RWGS reaction.
The synthesis parameters, such as gas composition, gas space velocity, heating rate, and final temperature, influence the final properties of molybdenum nitrides and carbides (Oyama, 1992; Nagai et al., 1998a; Xiao et al., 2000; Hanif et al., 2002; Mo et al., 2016; Cárdenas-Lizana et al., 2018). The presence of a support and its nature also affect the structural and catalytic properties (García Blanco et al., 2019). The crystallographic structure, the metal-to-carbon and metal-to-nitrogen ratio, and the Mo oxidation state are key parameters to be taken in consideration when evaluating the catalytic performances of Mo carbide and nitride catalysts for CO2 hydrogenation (Xu et al., 2014; Posada-Pérez et al., 2016a; García Blanco et al., 2019; Han et al., 2019). For example, at 250°C and 20 bar, hexagonal Mo2C exhibits higher activity and selectivity for methanation, whereas cubic MoC1−x is less active but more selective to CO and methanol (Xu et al., 2014). However, the opposite trend was observed elsewhere (García Blanco et al., 2019). This might be due to the different synthesis method employed in both studies, i.e., reduction–carburization (Xu et al., 2014) vs. carbothermal reduction route with sucrose (García Blanco et al., 2019). At high temperature [400°C Liu et al., 2017 or 600°C Gao et al., 2016], RWGS occurs and a high selectivity to CO (>98%) is obtained over bulk cubic and hexagonal molybdenum carbides. For Mo2C coated with nitrogen and sulfur-codoped carbon, Mo4+ favors the synthesis of methanol while methane is formed in the presence of materials with the highest contents of Mo2+ and Mo3+ (Han et al., 2019).
Materials and Methods
Preparation of Catalysts
Commercial TiO2-P (TiO2 P25, Degussa-Evonik, surface area 55 m2 g−1), TiO2-D (TiO2 DT51, Tronox, surface area 85 m2 g−1), and ZrO2 (MEL Chemicals, surface area 129 m2 g−1) were used as supports. Supported molybdenum carbide and nitride catalysts were prepared by impregnation followed by temperature-programmed reduction–carburization and reduction–nitridation, respectively. First, an appropriate amount (10–12% w/w Mo/support) of ammonium molybdate tetrahydrate (NH4)6Mo7O24.4H2O (Aldrich Chemical Co, 99.98% trace metals basis) was mixed with 5 g of support and 60 ml of water and stirred for 2 h at room temperature (RT). Water was evaporated under vacuum and the solid was dried in an oven in N2 at 80°C overnight. For the second step, the fine powder (1–5 g) was placed in a quartz cell under gas stream and submitted to a thermal treatment.
A range of supported molybdenum carbides was prepared by reduction–carburization under 60 ml min−1 (gas hourly space velocity, GHSV = 1090 or 1,530 h−1) of x % v/v C2H6/H2 or CH4/H2, with x = 5, 10, or 20%. The temperature was increased at 0.5°C min−1 up to the final temperature (600, 700, or 800°C) held for 2 h, and then decreased to RT while flowing argon (60 ml min−1).
Two different methods were used for the synthesis of supported molybdenum nitride. For method A, a gas stream of 150 ml min−1 (GHSV = 8,200 h−1) of 17% v/v N2/H2 was used. The temperature was raised at 5°C min−1 to 700°C and held for 2 h, and then decreased to RT while flowing N2 (60 ml min−1). For method B, a gas stream of 432 ml min−1 (GHSV = 23,600 h−1) of 83% v/v N2/H2 was used. The temperature was raised at 9.2°C min−1 to 300°C, then at 0.6°C min−1 to 500°C, then at 2°C min−1 to 700°C, held for 2 h, and then decreased to RT while flowing N2 (60 ml min−1).
The non-passivated (NP) samples were transferred into the reactor via a glovebox, in order to avoid exposure to air. All the other samples were passivated for 4 h under 1% v/v O2/N2 (60 ml min−1).
MoO3/TiO2-D was prepared by impregnation followed by calcination under 180 ml min−1 of air. The temperature was increased at 0.5°C min−1 to 600°C, held for 2 h, and cooled down to RT.
Characterization of Catalysts
The Mo content of the catalysts was determined by inductively coupled plasma optical emission spectroscopy (ICP-OES) with an Activa instrument from Horiba Jobin Yvon. Before analysis, the samples were mineralized by fusion with lithium tetraborate in Pt-Au crucibles at 1,100°C and then soaked with 20% HCl.
Carbon elemental analyses were conducted via a Leco SC144 micro-analyzer. The total combustion of the samples was performed at 1,050°C under a stream of helium and oxygen. The carbon was converted to carbon dioxide and quantified by a thermal conductivity detector (TCD).
Nitrogen elemental analyses were performed with a FLASH 2000 analyzer from ThermoFisher. The oxidation/reduction reaction of nitrogen-containing species into N2 gas was performed at 950°C with an O2 flow of 90 ml/min for 17 s. N2 was analyzed via separation on a packed column and detection with a TCD. Helium was used as a carrier gas with a flow of 140 ml/min and as a reference for the detector. A three-point calibration curve was built for quantification with methionine calibration samples purchased from ThermoFisher.
BET surface areas of the samples were determined from N2 physisorption after desorption at 150°C for 3 h under ultra-high vacuum (10−4 mbar) using an ASAP 2020 Micromeritics apparatus. The values are reported with an absolute precision of ± 5 m2 g−1.
Powder X-ray diffraction (XRD) patterns of the catalysts were recorded in the range 2θ = 10–90° at 0.04° s−1 using a Bruker D8 A25 X-ray diffractometer and a CuKα radiation source (λ = 1.54184 Å). Phase identification, lattice parameters, and mean crystallite sizes (d = 4/3 × LVol-IB, with LVol-IB being the volume-averaged column height) were obtained by performing Rietveld refinement using Topas 5 software.
Transmission electron microscopy (TEM) and scanning TEM–high angle annular dark field (STEM-HAADF) images were obtained using an image-aberration-corrected FEI Titan ETEM G2 instrument operated at 300 kV, equipped with an X-MAX SDD EDX detector from Oxford Instruments. Samples were prepared by dispersing the solids in ethanol and then depositing them onto carbon-coated copper grids.
Catalytic Testing
The catalyst evaluation in CO2 hydrogenation was carried out in a fixed-bed flow reactor. In standard conditions, a mass of 400 mg of the catalyst was placed in a tubular Pyrex glass reactor comprising a glass frit. This borosilicate tube was inserted into a stainless-steel U-shaped cover. A graphited Teflon gasket was put around the top of the borosilicate tube to hug the metal cover and pushed by a metal gasket, ensuring at the same time full flow inside the tube reactor and sealing of the cover. The reactor set was placed in a tubular resistive oven. The system was purged with the H2/CO2/N2 (30/10/10 ml/min) reaction mixture and then kept exposed to this flow till reaching a total pressure of 30 bar controlled with a back-pressure regulator, at which heating to 250°C was started. Every valve and connection was heated to maintain the mixture in gas phase for online analyses. All parameters of flow, pressure, and temperature were recorded using a home-made Labview program. The reaction was performed for 3 h. Some of the reaction conditions were varied: the mass of catalyst (400 or 800 mg), the flow of H2/CO2/N2 (30/10/10 or 50/10/10 ml/min), the reaction temperature (from 200 to 300°C), and the pressure (20 or 30 bar).
The reaction system was connected to a R3000 micro gas chromatograph (MicroGC) with a TCD detector, purchased from SRA Instruments, with which samples were taken every 3 min during the reaction. Three modules were used to analyze the gases. H2, N2, CO, and CH4 were analyzed with a 10-m Molsieve 5 A capillary column with a backflush injector and a 3-m PlotU precolumn. An 8-m Poraplot U capillary column was used for CO2, C2-C3 hydrocarbons, methanol, and water. A 10-m Stabilwax capillary column was employed for oxygenated compounds such as dimethyl ether, alcohols, and water as well. The MicroGC was calibrated and operated by using the Soprane software. For the determination of catalytic performances from GC data, we used the following definitions of conversion and selectivity:
where F(CO2)in and F(CO2)out are the incoming and outgoing flow rates of CO2, respectively.
where nc(P) is the number of carbon atoms per molecule of P and F(P)out is the (outgoing) flow rate of product P. Conversion and selectivity are given with an error of 8 and 7%, respectively.
Results and Discussion
Preparation and Characterization of Molybdenum Carbide Catalysts
A series of molybdenum carbide catalysts supported on TiO2-P, TiO2-D, and ZrO2 were synthesized from ethane and methane. The preparation conditions are reported in Table 1 and Table S1: molar fraction of hydrocarbon in H2 (from 5 to 20%), maximal temperature (600°C−800°C), and GHSV employed during reduction–carburization (1,090 or 1,530 h−1).
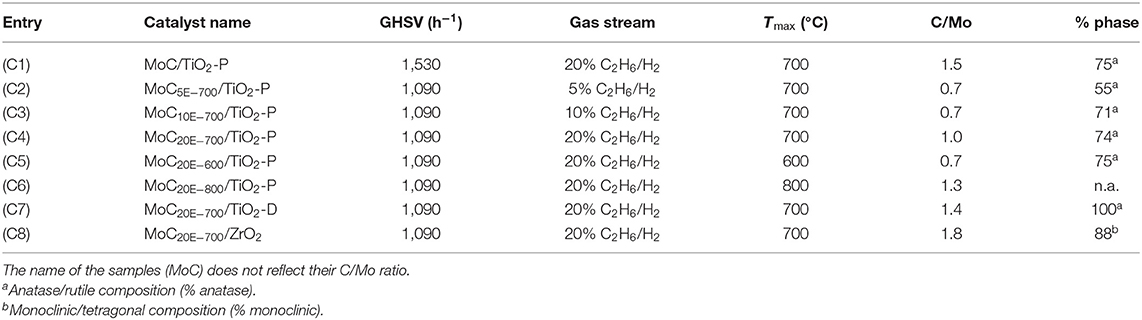
Table 1. List of the supported molybdenum carbide catalysts synthesized from C2H6/H2, with the corresponding preparation conditions, the C/Mo atomic ratio derived from elemental analyses, and the support composition (% phase).
The MoC/TiO2-P catalyst (entry C1, Table 1) was first synthesized and used to test several reaction conditions for the hydrogenation of CO2. However, a high GHSV (1,530 h−1) was employed during the synthesis, which resulted in a high carbon content (2.2 wt%) and an excess of carbon on the surface. Our group previously reported that the carbon content decreases as the GHSV decreases (Abou Hamdan et al., 2019), which can be ascribed to the higher hydrocarbon decomposition rate at higher flow rate (i.e., GHSV) (Solymosi et al., 1994). Therefore, the other catalysts were synthesized with a lower GHSV (1090 h−1) in order to limit the formation of graphite.
The molybdenum (9.1–11.8 wt%) and carbon (0.3–2.2 wt%) contents are reported in Table S2. The C/Mo atomic ratios (Table 1 and Table S1) are between 0.2 and 1.5, knowing that this value should be between 0.5 (Mo2C) and 1 (MoC) for stoichiometric fully carburized molybdenum carbide. As expected, MoC20E−700/TiO2-P (C4, Table 1) exhibits a lower C/Mo ratio than MoC/TiO2-P (1.0 vs. 1.5) as the GHSV is lower and the other parameters being identical. MoC20M−600/TiO2-P and MoC5M−700/TiO2-P are only partially carburized, owing probably to the low temperature and the low amount of methane in the gas stream, respectively, during reduction–carburization. It is expected that methane decomposition increases with increasing methane concentration in the methane/hydrogen mixture, as reported elsewhere (Ostrovski and Zhang, 2006). Consequently, the C/Mo ratio increases as the hydrocarbon concentration increases and reaches unity for MoC20E−700/TiO2-P. In addition, the degree of carburization is higher when using ethane instead of methane, as the carburization occurs at lower temperature (Hanif et al., 2002). A C/Mo ratio superior to 1.0 indicates an excess of carbon, associated with the presence of amorphous graphite on the surface, as demonstrated elsewhere by Raman analyses (Abou Hamdan et al., 2019). This is the case for the samples synthesized with a high amount of ethane, a high temperature or a high GHSV. This phenomenon is also more pronounced for TiO2-D and ZrO2 than for TiO2-P, suggesting an influence of the support on the decomposition of the hydrocarbon. There is evidence in the literature on supported Ni and Pd systems that CH4 decomposition depends on metal crystallite size, metal-support interaction, nature, and crystal phase of the support (e.g., tetragonal vs. monoclinic ZrO2) and on acid–base properties of the materials (Solymosi et al., 1994; Bengaard et al., 2002; Zhang et al., 2019). However, we were unable to find any related study for supported Mo or MoOx systems. It is worth pointing out that hydrocarbon decomposition and reduction of the precursor occur at the same time, which complicates the analysis. However, it is beyond the scope of this paper to go into a detailed discussion of hydrocarbon decomposition.
All the samples were characterized by XRD. The patterns associated with MoC10E−700/TiO2-P, MoC/TiO2-P, MoC20E−800/TiO2-P, and MoC20E−700/TiO2-D are presented in Figure 1, while all the others are included in Figures S1, S2. The patterns obtained for the catalysts synthesized at 600°C and 700°C present mainly the peaks associated with the support: a mixture of anatase and rutile phases for TiO2-P, anatase for TiO2-D, and a mixture of monoclinic and tetragonal phases for ZrO2. With catalysts based on TiO2-P (75% anatase/25% rutile), the final amount of anatase is between 55 and 75%, depending on the synthesis conditions (Table 1 and Table S1). In presence of Mo carbide, the anatase is stable up to 700°C under 20% hydrocarbon/H2 while it transforms into rutile under 5% and 10% hydrocarbon/H2 gas streams. Thus, the presence of fully carburized Mo carbide and/or free carbon seems to stabilize the anatase phase. However, after reduction–carburization at 800°C, the support is partially reduced to Ti5O9 and Ti4O7 (Figure 1C and Figure S2F). Finally, the crystallite sizes of the anatase phase (26–29 nm) are similar while the ones associated with rutile vary from 11 to 82 nm (Table S2). Regarding the catalysts based on TiO2-D, the anatase phase is stable, i.e., no rutile was observed and the crystallite size is similar to the bare support (ca. 26 nm). This agrees with the results observed for TiO2-P at 700°C under 20% hydrocarbon/H2. In the same manner, the ZrO2 support is stable, with a composition that remains at 88% monoclinic/12% tetragonal.
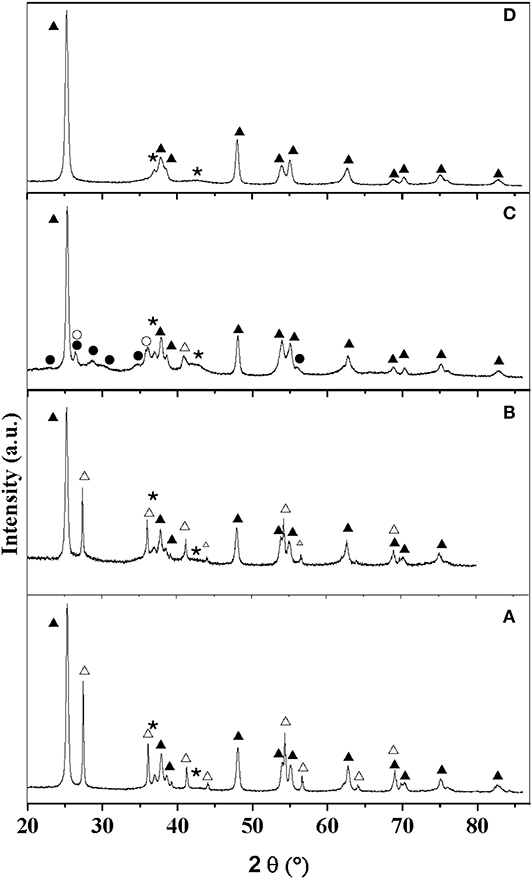
Figure 1. XRD patterns of the catalysts: (A) MoC10E−700/TiO2-P, (B) MoC/TiO2-P, (C) MoC20E−800/TiO2-P, and (D) MoC20E−700/TiO2-D. Assignment of peaks: (▴) anatase, (△) rutile, (•) Ti5O9, (○) Ti4O7, and (⋆) MoC.
The specific surface area of Mo carbide supported on TiO2-P and synthesized at 600°C (54 m2 g−1; Table S2) is similar to that of the bare support (55 m2 g−1). However, these values decrease to 43 m2 g−1 as the percentage of anatase decreases to 55%. Finally, the surface area is of 28 m2 g−1 due to the reduction of the support after treatment at 800°C, for MoC20M−800/TiO2-P. The catalysts supported on TiO2-D and ZrO2 exhibit surface areas similar to those of the bare supports (129 m2 g−1 for ZrO2; 90 m2 g−1 for TiO2-D).
XRD analyses suggest the formation of fcc MoC in all the samples. This phase is characterized by two main peaks at 36.4° and 42.2°, associated with the (111) and (002) planes (PDF 01-089-2868). While the first one overlaps with the peaks associated with the supports, the second one can be more easily observed. The Mo carbide crystallite sizes were estimated by Rietveld refinement to be around 2–4 nm for all the samples (Table S2).
STEM-HAADF images of the catalysts (Figure 2) confirm the presence of small particles (size < 4 nm) at the surface of the supports, independently of the synthesis conditions. The interplanar distances and angles estimated by electron diffraction and TEM analyses on selected samples (MoC20E−700/TiO2-D: Figure S3 and Table S3; MoC10E−700/TiO2-P: Figure S4 and Table S4) confirm the formation of the MoC phase with fcc structure.
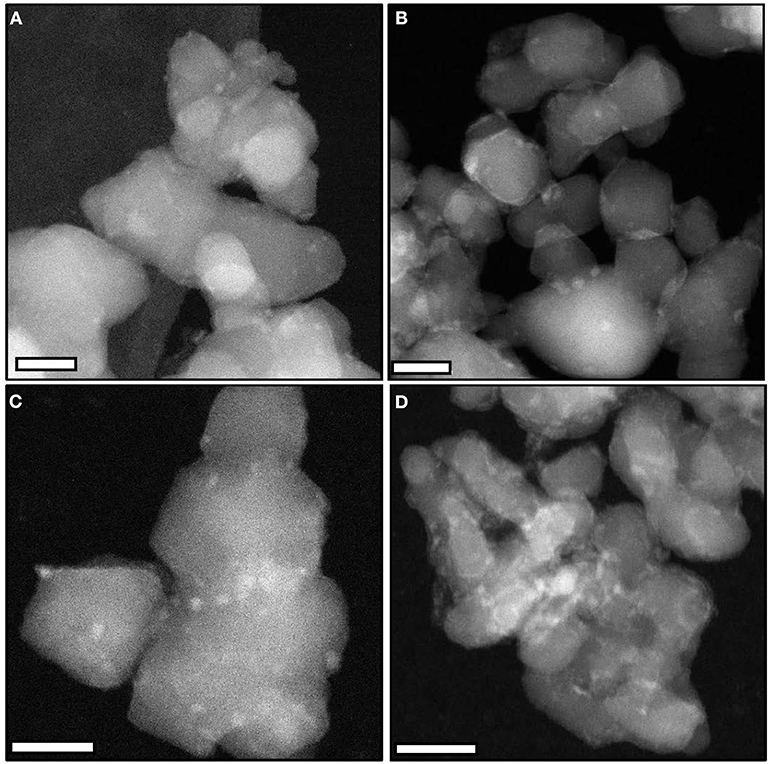
Figure 2. Representative STEM-HAADF images (scale bars, 20 nm) of (A) MoC/TiO2-P, (B) MoC10E−700/TiO2-P, (C) MoC10M−700/TiO2-P, and (D) MoC20E−700/TiO2-D.
Preparation and Characterization of Molybdenum Nitride Catalysts
In order to compare the nature of the atoms incorporated into the structure, a series of supported molybdenum nitride catalysts were synthesized using method A (MoNA) or method B (MoNB) with TiO2-P, TiO2-D, or ZrO2 as support (Table 2). Based on the literature dealing with the synthesis of bulk molybdenum nitride (Ghampson et al., 2012; Perret et al., 2012; Cárdenas-Lizana et al., 2018), the formation of hexagonal β-Mo2N is favored when using low GHSV, high heating ramp and low N2 content in the gas stream (method A) while the opposite conditions usually generate cubic γ-Mo2N with high surface area (method B).
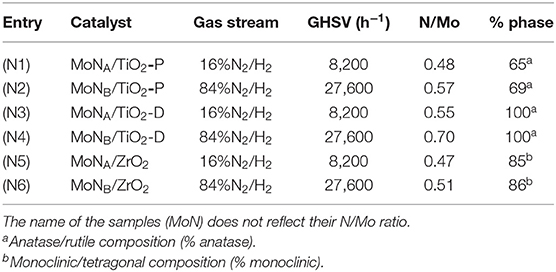
Table 2. List of the supported molybdenum nitride catalysts with the corresponding preparation conditions, the N/Mo atomic ratio derived from elemental analyses, and the support composition (% phase).
The molybdenum loading is around 9% for all the catalysts (Table S5). The N/Mo ratios presented in Table 2 are around 0.5, suggesting the formation of Mo2N. For all the samples, the ratios were slightly higher when synthesized with method B as compared to method A. MoNB/TiO2-D exhibits a ratio of 0.70, slightly above what has been reported in the literature for bulk molybdenum (N/Mo ratios: 0.3–0.5) (Gong et al., 2006; Kong et al., 2008; Cairns et al., 2009; Perret et al., 2012). This might be due to the incorporation of some nitrogen atoms in interstitial sites and defects of TiO2. This has previously been reported for molybdenum nitride supported on Al2O3 and SBA-15 (Ghampson et al., 2012; Zheng et al., 2013).
The diffraction patterns associated with MoNA/TiO2-P, MoNB/TiO2-P, and MoNB/TiO2-D are presented in Figure 3, while the other ones are reported in Figure S5. Rietveld refinements suggest the presence of small crystallites of cubic Mo nitride in all the samples (Table S5). However, the two main peaks of the cubic and hexagonal phases of Mo2N are close to each other (37.3°/43.3° and 37.3°/43.1°, respectively). As the minor diffraction lines were not observable by XRD due to the low loading and the small size, it is difficult to discriminate between the two phases. For MoNA/TiO2-P and MoNA/TiO2-D, an additional peak at 40° with a small intensity can be attributed to metallic Mo. In agreement with the elemental analyses, the results suggest that method A does not allow a full nitridation of the samples; hence, some metallic Mo is still present. Similarly to supported carbides, the thermal treatment under reducing atmosphere is associated with a slight transformation of anatase to rutile (from 75 to 65–69% of anatase) for TiO2-P, while TiO2-D and ZrO2 remain stable (Table 2).
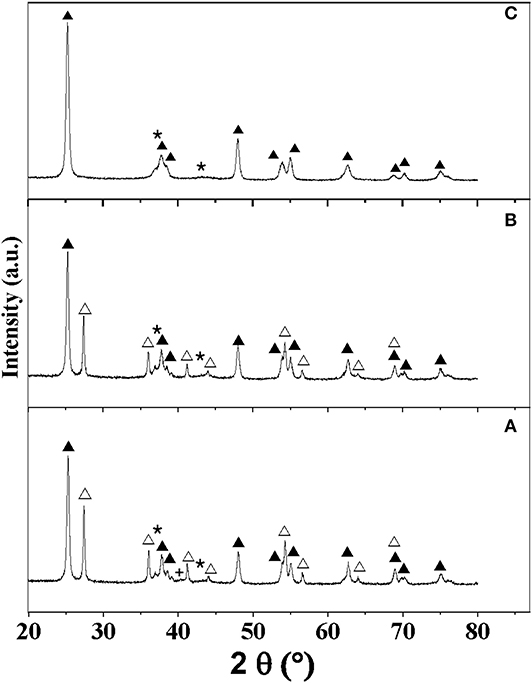
Figure 3. XRD patterns of the catalysts: (A) MoNA/TiO2-P, (B) MoNB/TiO2-P, and (C) MoNB/TiO2-D. Assignment of peaks: (▴) anatase, (△) rutile, (⋆) MoN, and (+) Mo.
The catalysts were also characterized by STEM, TEM, and electron diffraction, as shown in Figure 4. The catalysts exhibit small (<5 nm) Mo nitride nanoparticles. Moreover, the d-spacing and angles estimated for some particles correspond to cubic Mo2N (Figures S6, S7 and Tables S6, S7), in agreement with XRD.
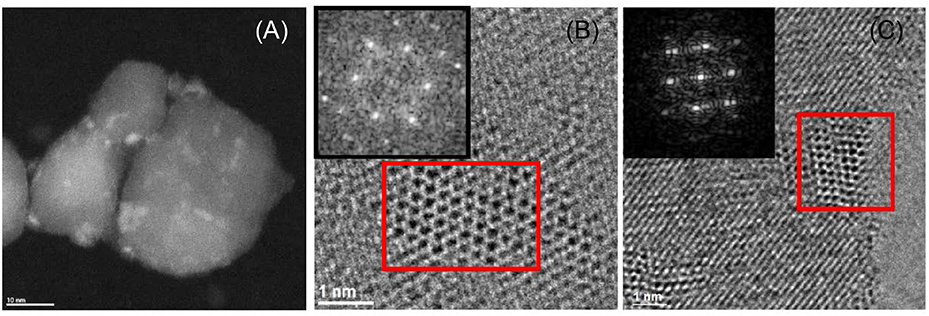
Figure 4. Representative STEM image of MoNB/TiO2-D (A); TEM images and electron diffraction patterns (corresponding to the red squares in the images) of MoNB/TiO2-D (B) and MoNA/ZrO2 (C).
CO2 Hydrogenation Over Supported Molybdenum Carbide
Preliminary Catalytic Test Over MoC/TiO2-P
All the preliminary tests were conducted with MoC/TiO2-P (C1, Table 1). For the first evaluation, the reaction conditions used by Rodriguez and coworkers (Xu et al., 2014) were employed. The reaction was performed at 250°C, with a total pressure of 20 bar and a total flow rate of 30 ml min−1 using a reaction mixture of 75% H2/15% CO2/10% N2. Figure S8A shows the temporal evolution of CO2 conversion and product yields. CO2 conversion started around 190°C; it increased with the temperature and reached a maximum (3.5%) at 113 min corresponding to the overshooting of the temperature of the oven (260°C). The evolution of the product selectivity as a function of temperature, shown in Figure S8B, reveals that, at low temperature, only CO was produced. Afterwards, a decrease in CO selectivity was observed, associated with a corresponding increase in CH4. In parallel, C2H6 and CH3OH were formed in small quantities; however, the selectivity of the former product gradually increased. Figure 5 presents CO2 conversion (around 2.5%) and selectivity to products once the temperature is stabilized at 250°C. At quasi-steady state, the major product is CO with a selectivity of 69%, followed by CH4 (24%), C2H6 (4%), and CH3OH (3%).
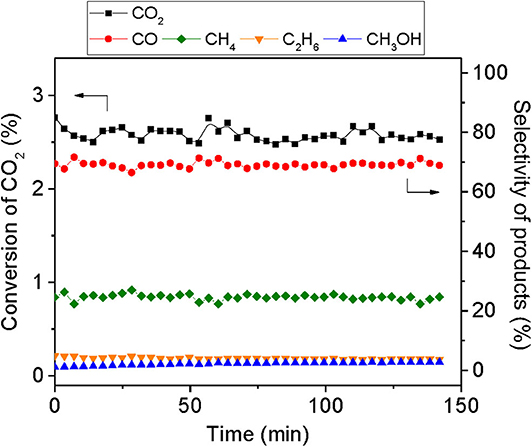
Figure 5. Temporal evolution of CO2 conversion and selectivity of products during the hydrogenation of CO2 over 400 mg of MoC/TiO2-P at 250°C and 20 bar total pressure, with a total flow rate of 30 ml min−1 of H2 /CO2/N2 with a H2:CO2 ratio of 5:1, 150 min on stream after stabilization of the temperature.
These results suggest that MoC/TiO2-P behaves primarily as a RWGS catalyst. According to the molybdenum carbide content, the conversion (2.5% for 56 mg of MoC) is similar to the one reported for a cubic molybdenum carbide catalyst under the same reactions conditions (11% conversion over 200 mg of MoC) (Xu et al., 2014). However, the product distribution is different and the present catalyst shows higher selectivity to CO and CH4 with lower selectivity to methanol. Indeed, the authors reported that cubic MoC is selective to CO (51%), CH3OH (23%), CH4 (16%), and C2H6 (3%), whereas hexagonal MoC leads to less CO (34%) and CH3OH (12%), but more CH4 (37%) and C2H6 (9%). The authors proposed that methane and ethane would result from the hydrogenation of the carbon generated from the full decomposition of CO2 on the surface. Our results are closer to the ones reported by García Blanco et al. (2019) over cubic MoC1−x under similar conditions (240°C, 20 bar). They measured an 8% conversion, with selectivity to CO (55%), CH4 (30%), C2H6 (4%), and methanol (3%).
Screening of the Reaction Conditions Over MoC/TiO2-P
Several reaction conditions were assessed by varying the total gas flow rate, the H2/CO2 molar ratio, the temperature, and the catalyst weight. Table S8 reports the CO2 conversion and product selectivity results at quasi-steady state. First, the reproducibility and the absence of mass transfer limitations were checked. Afterwards, the total flow rate of the reactant mixture was decreased from 50 ml min−1 to 10 ml min−1. As expected, decreasing the flow rate increased the conversion to the same extent (from 4 to 12%). The selectivity to CH4 and C2H6 increased at the detriment of CO and methanol.
The effect of temperature on the reaction kinetics was investigated for 200, 250, and 300°C. When the temperature increases, the quasi-steady-state conversion increases from 0.5 to 11.4%. This is associated with a slight increase in selectivity toward CO (from 72 to 79%) and C2H6 (from 0 to 3%), along with a decrease in selectivity toward CH4 (from 26 to 18%) and methanol (from 2 to 0.4%). Less methanol is expected when the temperature is increased, according to the Le Chatelier principle (Romero-Saez et al., 2019). However, as far as CO and methane are concerned, Xu et al. (2014) measured a lower CO selectivity and a higher CH4 selectivity at higher temperature for cubic MoC.
Finally, the influence of the passivation treatment on the catalytic performance of MoC/TiO2-P was evaluated by conducting another test with a fresh non-passivated catalyst transferred into the reactor through a glovebox. This catalyst appears more active than the passivated one, while the product selectivity is unaltered. This is consistent with the study of Nagai et al. (1998b), who showed that passivation decreases the activity of Mo carbide catalysts supported on alumina in CO2 hydrogenation at 300°C and atmospheric pressure (however, the authors did not mention whether passivation had an influence on the selectivity).
Effect of the Catalyst Carburization Conditions on the Activity of MoC/TiO2-P
MoC/TiO2-P catalysts were prepared with different methane or ethane concentrations (5, 10, and 20%) and carburization temperatures (600, 700, and 800). The XRD, STEM, and TEM analyses confirmed the formation of well-dispersed fcc MoC particles on the support. The main difference between these catalysts lies in the C/Mo ratios.
The evolution of CO2 conversion with time-on-stream for MoC/TiO2-P catalysts prepared with ethane is presented in Figure 6. Figure 7 reports the corresponding product distributions at quasi-steady state (CO is not shown). The general trend is that CO2 conversion decreases with the increase in carbon content (higher C/Mo ratio, Figure S9), which is in accordance with the literature (Posada-Pérez et al., 2014; Xu et al., 2014). Substantial increases in the selectivity toward CO and methanol were reported when moving from a C-rich surface to a Mo-rich surface. In our case, the selectivity to CO (72–75%), methane (20–23%), and ethane (3%) does not significantly differ between the catalysts. However, differences appear in terms of methanol selectivity, which varies from 1 to 3%. It is worth noting that all the conversions and selectivity remained stable with time. A previous study on supported molybdenum carbide (Mo2C/MCM-41) reported that the conversion decreased with time and the selectivity varied (Liu et al., 2016).
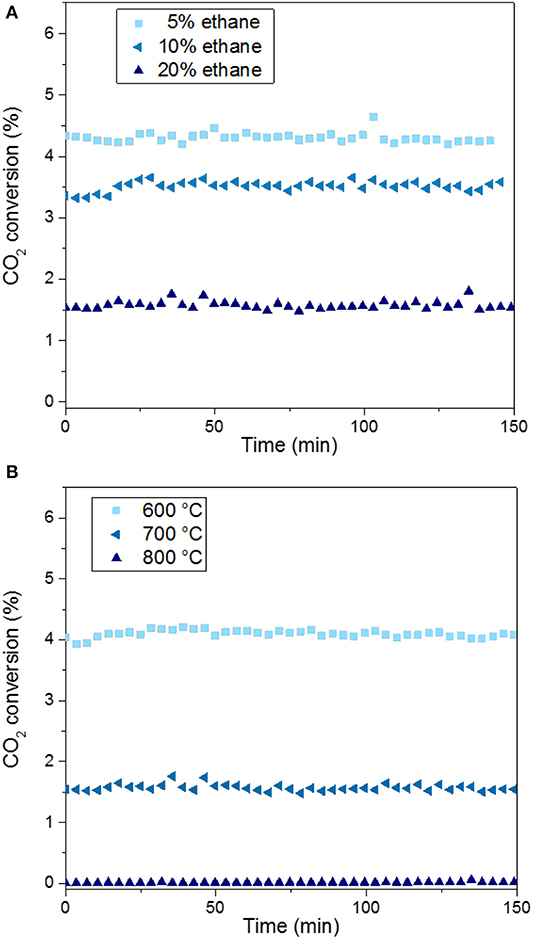
Figure 6. CO2 conversion over MoC/TiO2-P as a function of the amount of ethane used for carburization at 700°C (A) and the carburization temperature used for 20% ethane (B). Catalytic conditions: 400 mg of catalyst, 50 ml min−1, 250°C, 30 bar, H2:CO2 = 3:1, 150 min on stream after stabilization of the temperature.
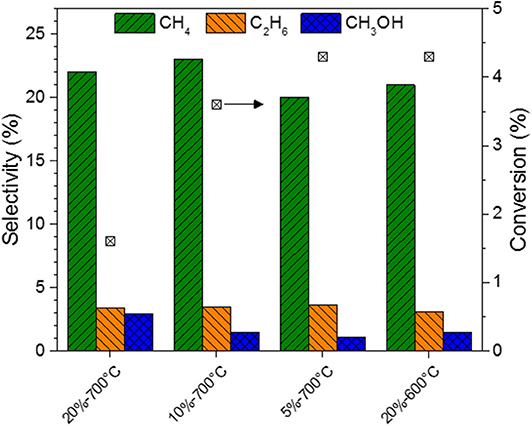
Figure 7. Selectivity to methane, ethane, and methanol, and conversion for MoC/TiO2-P as a function of the carburizing ethane concentration (5, 10, or 20% in H2), and the carburization temperature (600 or 700°C). Catalytic conditions: 400 mg of catalyst, 50 ml/min, 250°C, 30 bar, H2:CO2 = 3:1, 150 min on stream after stabilization of the temperature.
Regarding the catalysts synthesized at 700°C (Figure 6A), the higher the alkane concentration during the catalyst preparation, the lower the CO2 conversion is. The preparation of the carbide with a low concentration (5%) of ethane favors CO2 conversion: 4.3% for MoC5E−700/TiO2-P, which is the highest value. In contrast, a high concentration of ethane (MoC20E−700/TiO2-P) leads to the highest selectivity to methanol (3%), but at the lowest conversion (1.5%).
The performances of the catalysts prepared with ethane at different carburization temperatures are shown in Figure 6B. The conversion decreases from 4% to near-zero value with the increase in the carburization temperature from 600 to 800°C. The graphitic carbon present on the surface of the fully carburized catalysts may block the access of the reactants to the active surface of the material, i.e., it may act as a poison, as previously reported (Nagai et al., 1998b). The support undergoes significant phase changes at 800°C (section Preparation and Characterization of Molybdenum Carbide Catalysts), which might also explain the lack of activity of MoC20E−800/TiO2-P. As this catalyst was barely active, its selectivity is not reported in Figure 7.
The same trends are observed when using methane as carburizing hydrocarbon (Figures S10, S11). Thus, the increase in carbon content of the carbide appears to moderate its activity, consistently with previous work (Posada-Pérez et al., 2016a). The selectivity to methanol increases up to 3% with the increase in temperature of preparation or hydrocarbon content, when using ethane. The highest selectivity to methanol (5.3%) is observed for the catalyst with the lowest C/Mo ratio, i.e., MoC20M−600/TiO2-P.
Effect of the Support Nature
The nature of the support is known to affect catalytic performances. For example, Kim et al. have shown that the support crystal structure of Ru/TiO2 strongly affects the hydrogenation of CO2 (Kim et al., 2018). Moreover, PtCo/ZrO2 and PtCo/TiO2 exhibit distinct selectivity in CO2 hydrogenation, the former catalyst being more selective to CH4 (Kattel et al., 2016). In both studies, the differences in behaviors were attributed to distinct metal–support interactions in the catalysts.
The performances of Mo carbide catalysts synthesized from ethane at 700°C and supported on TiO2-P, TiO2-D, and ZrO2 are compared in Table 3. The catalyst supported on TiO2-D (pure anatase) shows a higher conversion than those supported on TiO2-P and ZrO2. This is in agreement with the results obtained with Ru/TiO2, where the conversion of CO2 increases with the anatase content in the support (Kim et al., 2018). A significant difference is observed in the product selectivity, which is affected by the nature of the support more than by the C/Mo ratio. The selectivity to methanol of the catalyst supported on TiO2-D is superior by ca. 8% compared to the case of TiO2-P. This catalyst also shows lower CO and CH4 selectivity. The selectivity of MoC20E−700/ZrO2 is similar to that of MoC20E−700/TiO2-P. The same trends are observed for the catalysts synthesized using methane as hydrocarbon (Table S9). These results make the use of TiO2-D preferable over the other supports as CO2 conversion and methanol selectivity both increase in the order: MoC supported on TiO2-D > TiO2-P ≈ ZrO2.
The active sites reported for molybdenum carbide catalysts in the hydrogenation of CO2 are Mo-terminated and C-terminated Mo2C (Posada-Pérez et al., 2016b). Molybdenum and carbon defects are also present at the surface and may affect the catalytic response (de Oliveira et al., 2014). As the conversion increases with the decrease in C/Mo ratio (section Effect of the Catalyst Carburization Conditions on the Activity of MoC/TiO2-P), our results suggest that the amount of carbon vacancies affects the catalytic performance and/or that the C-terminated sites are inactive. The supported carbides were compared with MoO3/TiO2-D in order to assess the respective roles of molybdenum and carbon species. The XRD diffraction pattern (Figure S12) confirmed the formation of orthorhombic MoO3. A very low conversion (0.5%) was measured with this catalyst (Table 3); hence, Mo6+ are not active for this reaction. We have previously shown that MoC/TiO2 exhibits mainly Moδ+ (δ+ < 2) species (Abou Hamdan et al., 2019). Therefore, it seems that the combination of Moδ+ (δ+ < 2) species with C vacancies is favorable to the hydrogenation of CO2.
CO2 Hydrogenation Over Supported Molybdenum Nitride
The series of Mo nitride catalysts supported on TiO2-P, TiO2-D, and ZrO2 was tested for the hydrogenation of CO2 in the same conditions as those used for the carbides. The performances at quasi-steady state are reported in Figure 8. The conversions are in the range 0.7–1.1%, which implies that the nitride catalysts are less active than their carbide counterparts. Similarly to the carbides, the activity of supported MoN varies in the order: TiO2-D > P25 TiO2-P ≈ ZrO2.
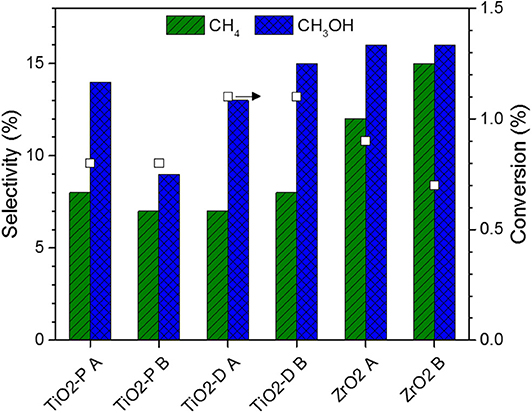
Figure 8. Conversion and selectivity for supported Mo nitride catalysts. Catalytic conditions: 400 mg of catalyst, 50 ml min−1, 250°C, 30 bar, H2:CO2 = 3:1, 150 min on stream after stabilization of the temperature.
The selectivity to CO, methane, and methanol are in the ranges 69–84, 7–15, and 9–16%, respectively. The highest CO selectivity are those of the TiO2-supported samples, while the highest methane and methanol selectivity are those of ZrO2-supported catalysts. With respect to the carbides, the CO/CH4 selectivity ratio is higher for the nitrides. Moreover, no ethane is produced on these catalysts, which suggests a weaker adsorption of CO2, leading to the absence of CO2 decomposition to atomic carbon (Xu et al., 2014).
Finally, despite the different degrees of nitridation provided by methods A and B, the synthesis method poorly affects the catalytic performance, confirming the low activity of N-terminated sites. Moreover, Mo2N catalysts are characterized by the presence of Moδ+ (2 ≤ δ+ < 4) (Perret et al., 2012), which confirms that the oxidation states of Mo affect the hydrogenation of CO2.
γ-Mo2N synthesized using reduction–nitridation under NH3 at 700°C was reported to be active and selective for the RWGS reaction (Yao et al., 2019). At 350°C, ca. 2.5% conversion and a CO selectivity above 98% was measured. Our results confirm that Mo2N is able to uptake and activate CO2 as ca. 1% conversion was observed under our reaction conditions. However, supported molybdenum carbides outperform the nitride counterparts. Simple ways of increasing CO2 conversions consist in decreasing the reactant gas flow rate and increasing catalyst weight, as done during the preliminary tests (Table S8).
Conclusion
Molybdenum carbide and nitride nanoparticles supported on TiO2 and ZrO2 were evaluated in the hydrogenation of CO2 for the first time. The catalysts were synthesized by impregnation followed by reduction–carburization or reduction–nitridation. Synthesis parameters such as temperature, nature, and composition of the gas stream, and space velocity were varied. Characterization by TEM/STEM and XRD showed the formation of cubic MoC and Mo2N nanoparticles. Different degrees of carburization (Mo/C molar ratio) and nitridation (Mo/N) were obtained depending on the synthesis conditions.
For MoC/TiO2, employing a low concentration of hydrocarbon in hydrogen and a low temperature during the synthesis results in a lower C/Mo ratio and a higher activity. Moreover, the presence of graphite inhibits the reaction. The carbide catalysts generate mainly CO, along with methane, ethane, and methanol. The nature of the support affects the catalytic performances, which are higher for TiO2-D (commercial DT51) support. The preparation conditions and the support affect the selectivity to methanol, which varies from 1 to 11%. The Mo nitride catalysts are less active than their carbide counterparts. The Mo/N molar ratio does not affect the conversion and the product distribution. Regarding the carbide catalysts, the presence of carbon vacancies seems to be crucial. Further work will focus on the control of the amount of these vacancies in an attempt to increase the catalytic activity and tune the selectivity.
Data Availability Statement
The original contributions presented in the study are included in the article/Supplementary Materials, further inquiries can be directed to the corresponding author/s.
Author Contributions
MA and AN conducted the experiments. MA wrote the manuscript. RC set up the catalytic bench and helped with operating the experiments. MJ and CP assisted in supervising the work, data analyzing, and quality controlling. LP and NP designed the study, acquired the founding, supervised the work, and reviewed the manuscript.
Conflict of Interest
The authors declare that the research was conducted in the absence of any commercial or financial relationships that could be construed as a potential conflict of interest.
Acknowledgments
This work was partially funded by CNRS through its Energy department (Cellule Energie), project CARNICO2. The authors acknowledge IRCELYON's characterization platform. They also thank Patrick Jame and Erik Bonjour (ISA) for conducting the elemental analyses, and Franck Morfin (IRCELYON) for his help with CO2 hydrogenation data analysis.
Supplementary Material
The Supplementary Material for this article can be found online at: https://www.frontiersin.org/articles/10.3389/fchem.2020.00452/full#supplementary-material
References
Abou Hamdan, M., Loridant, S., Jahjah, M., Pinel, C., and Perret, N. (2019). TiO2-supported molybdenum carbide: an active catalyst for the aqueous phase hydrogenation of succinic acid. Appl. Catal. A Gen. 571, 71–81. doi: 10.1016/j.apcata.2018.11.009
Alexander, A.-M., and Hargreaves, J. S. J. (2010). Alternative catalytic materials: carbides, nitrides, phosphides and amorphous boron alloys. Chem. Soc. Rev. 39:4388. doi: 10.1039/b916787k
Bengaard, H. S., Nørskov, J. K., Sehested, J., Clausen, B. S., Nielsen, L. P., Molenbroek, A. M., et al. (2002). Steam reforming and graphite formation on Ni catalysts. J. Catal. 209, 365–384. doi: 10.1006/jcat.2002.3579
Cairns, A. G., Gallagher, J. G., Hargreaves, J. S. J., Mckay, D., Morrison, E., Rico, J. L., et al. (2009). The influence of precursor source and thermal parameters upon the formation of beta-phase molybdenum nitride. J. Alloys Compd. 479, 851–854. doi: 10.1016/j.jallcom.2009.01.065
Cárdenas-Lizana, F., Lamey, D., Kiwi-Minsker, L., and Keane, M. A. (2018). Molybdenum nitrides: a study of synthesis variables and catalytic performance in acetylene hydrogenation. J. Mater. Sci. 53, 6707–6718. doi: 10.1007/s10853-018-2009-x
Chen, Y., Choi, S., and Thompson, L. T. (2015). Low-temperature CO2 hydrogenation to liquid products via a heterogeneous cascade catalytic system. ACS Catal. 5, 1717–1725. doi: 10.1021/cs501656x
Chen, Y., Choi, S., and Thompson, L. T. (2016). Low temperature CO2 hydrogenation to alcohols and hydrocarbons over Mo2C supported metal catalysts. J. Catal. 343, 147–156. doi: 10.1016/j.jcat.2016.01.016
de Oliveira, C., Salahub, D. R., de Abreu, H. A., and Duarte, H. A. (2014). Native defects in α-Mo2C: insights from first-principles calculations. J. Phys. Chem. C 118, 25517–25524. doi: 10.1021/jp507947b
Gao, J., Wu, Y., Jia, C., Zhong, Z., Gao, F., Yang, Y., et al. (2016). Controllable synthesis of α-MoC1-x and β-Mo2C nanowires for highly selective CO2 reduction to CO. Catal. Commun. 84, 147–150. doi: 10.1016/j.catcom.2016.06.026
García Blanco, A. A., Furlong, O. J., Stacchiola, D. J., Sapag, K., and Nazzarro, M. S. (2019). Porous MoxCy/SiO2 material for CO2 hydrogenation. Top. Catal. 62, 1026–1034. doi: 10.1007/s11244-019-01195-w
Ghampson, I. T., Sepúlveda, C., Garcia, R., García Fierro, J. L., Escalona, N., and DeSisto, W. J. (2012). Comparison of alumina- and SBA-15-supported molybdenum nitride catalysts for hydrodeoxygenation of guaiacol. Appl. Catal. A Gen. 43, 51–60. doi: 10.1016/j.apcata.2012.05.039
Gong, S., Chen, H., Li, W., and Li, B. (2005). Synthesis of β-Mo2N0.78 hydrodesulfurization catalyst in mixtures of nitrogen and hydrogen. Appl. Catal. A Gen. 279, 257–261. doi: 10.1016/j.apcata.2004.10.038
Gong, S. W., Chen, H. K., Li, W., and Li, B. Q. (2006). Catalytic behaviors of β-Mo2N0.78 as a hydrodesulfurization catalyst. Energy Fuels 20, 1372–1376. doi: 10.1021/ef050208b
Han, H., Geng, W., Xiao, L., and Wu, W. (2019). High selective synthesis of methanol from CO2 over Mo2C@NSC. J. Taiwan Inst. Chem. Eng. 95, 112–118. doi: 10.1016/j.jtice.2018.10.005
Hanif, A., Xiao, T., York, A. P. E., Sloan, J., and Green, M. L. H. (2002). Study on the structure and formation mechanism of molybdenum carbides. Chem. Mater. 14, 1009–1015. doi: 10.1021/cm011096e
Hargreaves, J. S. J. (2014). Nitrides as ammonia synthesis catalysts and as potential nitrogen transfer reagents. Appl. Petrochem. Res. 4, 3–10. doi: 10.1007/s13203-014-0049-y
Kattel, S., Liu, P., and Chen, J. G. (2017). Tuning selectivity of CO2 hydrogenation reactions at the metal/oxide interface. J. Am. Chem. Soc. 139, 9739–9754. doi: 10.1021/jacs.7b05362
Kattel, S., Yu, W., Yang, X., Yan, B., Huang, Y., Wan, W., et al. (2016). CO2 hydrogenation over oxide-supported PtCo catalysts: the role of the oxide support in determining the product selectivity. Angew. Chem. Int. Ed. 55, 7968–7973. doi: 10.1002/anie.201601661
Khodakov, A. Y., Chu, W., and Fongarland, P. (2007). Advances in the development of novel cobalt fischer–tropsch catalysts for synthesis of long-chain hydrocarbons and clean fuels. Chem. Rev. 107, 1692–1744. doi: 10.1021/cr050972v
Kim, A., Debecker, D. P., Devred, F., Dubois, V., Sanchez, C., and Sassoye, C. (2018). CO2 methanation on Ru/TiO2 catalysts: on the effect of mixing anatase and rutile TiO2 supports. Appl. Catal. B Environ. 220, 615–625. doi: 10.1016/j.apcatb.2017.08.058
Kong, L., Wu, J., Lü, B., Li, Q., and Xiong, T. (2008). Midscale preparation and characterization of transient metal nitride catalyst. Ind. Eng. Chem. Res. 47, 1779–1783. doi: 10.1021/ie070829n
Kunkel, C., Viñes, F., and Illas, F. (2016). Transition metal carbides as novel materials for CO2 capture, storage, and activation. Energy Environ. Sci. 9, 141–144. doi: 10.1039/C5EE03649F
Kunkel, C., Viñes, F., and Illas, F. (2018). Biogas upgrading by transition metal carbides. ACS Appl. Energy Mater. 1, 43–47. doi: 10.1021/acsaem.7b00086
Kunkel, C., Viñes, F., Ramírez, P. J., Rodriguez, J. A., and Illas, F. (2019). Combining theory and experiment for multitechnique characterization of activated CO2 on transition metal carbide (001) surfaces. J. Phys. Chem. C 123, 7567–7576. doi: 10.1021/acs.jpcc.7b12227
Liu, X., Kunkel, C., Ramírez de la Piscina, P., Homs, N., Viñes, F., and Illas, F. (2017). Effective and highly selective CO generation from CO2 using a polycrystalline α-Mo2C catalyst. ACS Catal. 7, 4323–4335. doi: 10.1021/acscatal.7b00735
Liu, X., Song, Y., Geng, W., Li, H., Xiao, L., and Wu, W. (2016). Cu-Mo2C/MCM-41: an efficient catalyst for the selective synthesis of methanol from CO2. Catalysts 6:75. doi: 10.3390/catal6050075
Liu, Y., and Liu, D. (1999). Study of bimetallic Cu-Ni/γ-Al2O3 catalysts for carbon dioxide hydrogenation. Int. J. Hydrogen Energy 24, 351–354. doi: 10.1016/S0360-3199(98)00038-X
Mikkelsen, M., Jørgensen, M., and Krebs, F. C. (2010). The teraton challenge. A review of fixation and transformation of carbon dioxide. Energy Environ. Sci. 3, 43–81. doi: 10.1039/B912904A
Mo, T., Xu, J., Yang, Y., and Li, Y. (2016). Effect of carburization protocols on molybdenum carbide synthesis and study on its performance in CO hydrogenation. Catal. Today 261, 101–115. doi: 10.1016/j.cattod.2015.07.014
Nagai, M., Goto, Y., Uchino, O., and Omi, S. (1998a). TPD and XRD studies of molybdenum nitride and its activity for hydrodenitrogenation of carbazole. Catal. Today 43, 249–259. doi: 10.1016/S0920-5861(98)00154-0
Nagai, M., Oshikawa, K., Kurakami, T., Miyao, T., and Omi, S. (1998b). Surface properties of carbided molybdena-alumina and its activity for CO2 hydrogenation. J. Catal. 180, 14–23. doi: 10.1006/jcat.1998.2262
Olah, G. A., Goeppert, A., and Prakash, G. K. S. (2009). Chemical recycling of carbon dioxide to methanol anddimethyl ether: from greenhouse gas to renewable, environmentally carbon neutral fuels and synthetic hydrocarbons. J. Org. Chem. 74, 487–498. doi: 10.1021/jo801260f
Ostrovski, O., and Zhang, G. (2006). Reduction and carburization of metal oxides by methane-containing gas. AIChE J. 52, 300–310. doi: 10.1002/aic.10628
Ott, J., Gronemann, V., Pontzen, F., Fiedler, E., Grossmann, G., Kersebohm, D. B., et al. (2012). “Methanol,” in Ullmann's Encyclopedia of Industrial Chemistry (Weinheim: Wiley-VCH Verlag GmbH & Co. KGaA), 1–27. doi: 10.1002/14356007.a16_465.pub3
Oyama, S. T. (1992). Preparation and catalytic properties of transition metal carbides and nitrides. Catal. Today 15, 179–200. doi: 10.1016/0920-5861(92)80175-M
Perret, N., Cárdenas-Lizana, F., Lamey, D., Laporte, V., Kiwi-Minsker, L., and Keane, M. A. (2012). Effect of crystallographic phase (β vs. γ) and surface area on gas phase nitroarene hydrogenation over Mo2N and Au/Mo2N. Top. Catal. 55, 955–968. doi: 10.1007/s11244-012-9881-4
Porosoff, M. D., Yang, X., Boscoboinik, J. A., and Chen, J. G. (2014). Molybdenum carbide as alternative catalysts to precious metals for highlyselective reduction of CO2 to CO. Angew. Chem. Int. Ed. 53, 6705–6709. doi: 10.1002/anie.201404109
Posada-Pérez, S., Ramírez, P. J., Evans, J., Viñes, F., Liu, P., Illas, F., et al. (2016a). Highly active Au/δ-MoC and Cu/δ-MoC catalysts for the conversion of CO2: the metal/c ratio as a key factor defining activity, selectivity, and stability. J. Am. Chem. Soc. 138, 8269–8278. doi: 10.1021/jacs.6b04529
Posada-Pérez, S., Ramírez, P. J., Gutiérrez, R. A., Stacchiola, D. J., Viñes, F., Liu, P., et al. (2016b). The conversion of CO2 to methanol on orthorhombic β-Mo2C and Cu/β-Mo2C catalysts: mechanism for admetal induced change in the selectivity and activity. Catal. Sci. Technol. 6, 6766–6777. doi: 10.1039/C5CY02143J
Posada-Pérez, S., Viñes, F., Ramirez, P. J., Vidal, A. B., Rodriguez, J. A., and Illas, F. (2014). The bending machine: CO2 activation and hydrogenation on δ-MoC(001) and β-Mo2C(001) surfaces. Phys. Chem. Chem. Phys. 16, 14912–14921. doi: 10.1039/C4CP01943A
Posada-Pérez, S., Vines, F., Rodriguez, J. A., and Illas, F. (2015). Fundamentals of methanol synthesis on metal carbide based catalysts: activation of CO2 and H2. Top. Catal. 58, 159–173. doi: 10.1007/s11244-014-0355-8
Reddy, K. P., Dama, S., Mhamane, N. B., Ghosalya, M. K., Raja, T., Satyanarayana, C. V., et al. (2019). Molybdenum carbide catalyst for the reduction of CO2 to CO: surface science aspects by NAPPES and catalysis studies. Dalton Trans. 48, 12199–12209. doi: 10.1039/C9DT01774G
Rodriguez, J. A., Evans, J., Feria, L., Vidal, A. B., Liu, P., Nakamura, K., et al. (2013). CO2 hydrogenation on Au/TiC, Cu/TiC, and Ni/TiC catalysts: production of CO, methanol, and methane. J. Catal. 307, 162–169. doi: 10.1016/j.jcat.2013.07.023
Romero-Saez, M., Jaramillo, L. Y., Henao, W., and de la Torre, U. (2019). “Nanomaterials for CO2 hydrogenation,” in Emerging Nanostructured Materials for Energy and Environmental Science, Environmental Chemistry for a Sustainable World, eds S. Rajendran, M. Naushad, K. Raju, and R. Boukherroub (Dordrecht; Heidelberg; London; New York, NY: Springer), 173–214. doi: 10.1007/978-3-030-04474-9_4
Saeidi, S., Amin, N. A. S., and Rahimpour, M. R. (2014). Hydrogenation of CO2 to value-added products-A review and potential future developments. J. CO2 Util. 5, 66–81. doi: 10.1016/j.jcou.2013.12.005
Sakakura, T., Choi, J.-C., and Yasuda, H. (2007). Transformation of carbon dioxide. Chem. Rev. 107, 2365–2387. doi: 10.1021/cr068357u
Schiebahn, S., Grube, T., Robinius, M., Tietze, V., Kumar, B., and Stolten, D. (2015). Power to gas: technological overview, systems analysis and economic assessment for a case study in Germany. Int. J. Hydrogen Energy 40, 4285–4294. doi: 10.1016/j.ijhydene.2015.01.123
Solymosi, F., Erdöhelyi, A., Cserényi, J., and Felvégi, A. (1994). Decomposition of CH4 over supported Pd catalysts. J. Catal. 147, 272–278. doi: 10.1006/jcat.1994.1138
Solymosi, F., Oszkó, A., Bánsági, T., and Tolmacsov, P. (2002). Adsorption and reaction of CO2 on Mo2C catalyst. J. Phys. Chem. B 106, 9613–9618. doi: 10.1021/jp0203696
Stangeland, K., Kalai, D., Li, H., and Yu, Z. (2017). CO2 methanation: the effect of catalysts and reaction conditions. Energy Procedia 105, 2022–2027. doi: 10.1016/j.egypro.2017.03.577
Wang, W., Wang, S., Ma, X., and Gong, J. (2011). Recent advances in catalytic hydrogenation of carbon dioxide. Chem. Soc. Rev. 40, 3703–3727. doi: 10.1039/c1cs15008a
Wei, W., and Jinlong, G. (2011). Methanation of carbon dioxide: an overview. Front. Chem. Sci. Eng. 5:3. doi: 10.1007/s11705-010-0528-3
Xiao, T., York, A. P. E., Williams, V. C., Al-Megren, H., Hanif, A., Zhou, X., et al. (2000). Preparation of molybdenumcarbides using butane and their catalytic performance. Chem. Mater. 12, 3896–3905. doi: 10.1021/cm001157t
Xu, W., Ramirez, P. J., Stacchiola, D., Brito, J. L., and Rodriguez, J. A. (2015). The carburization of transition metal molybdates (MxMoO4, M=Cu, Ni or Co) and the generation of highly active metal/carbide catalysts for CO2 hydrogenation. Catal. Lett. 145, 1365–1373. doi: 10.1007/s10562-015-1540-5
Xu, W., Ramirez, P. J., Stacchiola, D., and Rodriguez, J. A. (2014). Synthesis of α-MoC1-x and β-MoCy catalysts for CO2 hydrogenation by thermal carburization of Mo-oxide in hydrocarbon and hydrogen mixtures. Catal. Lett. 144, 1418–1424. doi: 10.1007/s10562-014-1278-5
Yao, S., Lin, L., Liao, W., Rui, N., Li, N., Liu, Z., et al. (2019). Exploring metal-support interactions to immobilize subnanometer Co clusters on γ-Mo2N: a highly selective and stable catalyst for CO2 activation. ACS Catal. 9, 9087–9097. doi: 10.1021/acscatal.9b01945
Zhang, X., Zhang, M., Zhang, J., Zhang, Q., Tsubaki, N., Tan, Y., et al. (2019). Methane decomposition and carbon deposition over Ni/ZrO2 catalysts: comparison of amorphous, tetragonal, and monoclinic zirconia phase. Int. J. Hydrogen Energy 44, 17887–17899. doi: 10.1016/j.ijhydene.2019.05.174
Keywords: CO2 hydrogenation, carbide, nitride, supported catalysts, TiO2, ZrO2
Citation: Abou Hamdan M, Nassereddine A, Checa R, Jahjah M, Pinel C, Piccolo L and Perret N (2020) Supported Molybdenum Carbide and Nitride Catalysts for Carbon Dioxide Hydrogenation. Front. Chem. 8:452. doi: 10.3389/fchem.2020.00452
Received: 02 April 2020; Accepted: 30 April 2020;
Published: 09 June 2020.
Edited by:
Svetlana Ivanova, University of Seville, SpainReviewed by:
Tomas Ramirez Reina, University of Surrey, United KingdomCristina Megías-Sayago, Université de Strasbourg, France
Copyright © 2020 Abou Hamdan, Nassereddine, Checa, Jahjah, Pinel, Piccolo and Perret. This is an open-access article distributed under the terms of the Creative Commons Attribution License (CC BY). The use, distribution or reproduction in other forums is permitted, provided the original author(s) and the copyright owner(s) are credited and that the original publication in this journal is cited, in accordance with accepted academic practice. No use, distribution or reproduction is permitted which does not comply with these terms.
*Correspondence: Laurent Piccolo, bGF1cmVudC5waWNjb2xvQGlyY2VseW9uLnVuaXYtbHlvbjEuZnI=; Noémie Perret, bm9lbWllLnBlcnJldEBpcmNlbHlvbi51bml2LWx5b24xLmZy