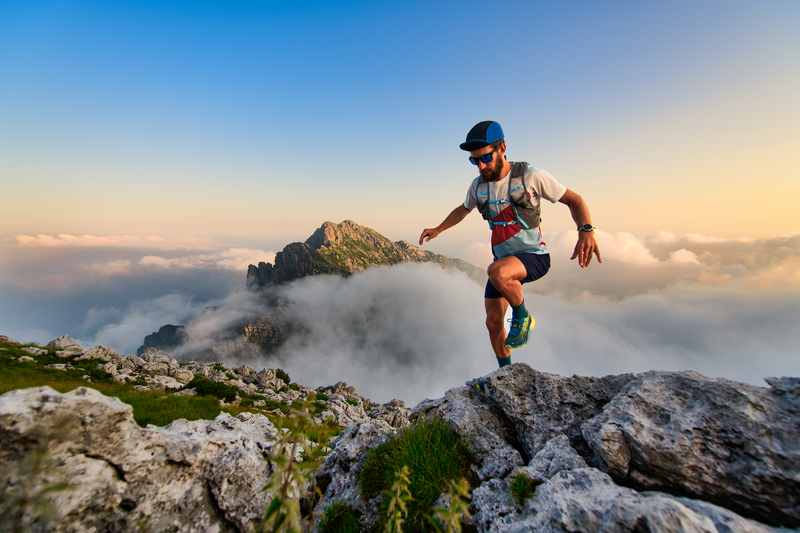
94% of researchers rate our articles as excellent or good
Learn more about the work of our research integrity team to safeguard the quality of each article we publish.
Find out more
ORIGINAL RESEARCH article
Front. Chem. , 19 June 2020
Sec. Medicinal and Pharmaceutical Chemistry
Volume 8 - 2020 | https://doi.org/10.3389/fchem.2020.00433
The appearance of drug-resistant strains of Mycobacterium tuberculosis and the dramatic increase in infection rates worldwide evidences the urgency of developing new and effective compounds for treating tuberculosis. Benzimidazoles represent one possible source of new compounds given that antimycobacterial activity has already been documented for some derivatives, such as those bearing electron-withdrawing groups. The aim of this study was to synthesize two series of benzimidazoles, di- and trisubstituted derivatives, and evaluate their antimycobacterial activity. Accordingly, 5a and 5b were synthesized from hydroxymoyl halides 3a and 3b, and nitro-substituted o-phenylenediamine 4. Compound 11 was synthesized from an aromatic nitro compound, 4-chloro-1,2-phenylenediamine 9, mixed with 3-nitrobenzaldehyde 10, and bentonite clay. Although the synthesis of 11 has already been reported, its antimycobacterial activity is herein examined for the first time. 1,2,5-trisubstituted benzimidazoles 7a, 7b, and 12 were obtained from N-alkylation of 5a, 5b, and 11. All benzimidazole derivatives were characterized by FT-IR, NMR, and HR-MS, and then screened for their in vitro antimycobacterial effect against the M. tuberculosis H37Rv strain. The N-alkylated molecules (7a, 7b, and 12) generated very limited in vitro inhibition of mycobacterial growth. The benzimidazoles (5a, 5b, and 11) showed in vitro potency against mycobacteria, reflected in minimal inhibitory concentration (MIC) values in the range of 6.25–25 μg/mL. Consequently, only the 2,5-disubstituted benzimidazoles were assessed for biological activity on mouse macrophages infected with M. tuberculosis. A good effect was found for the three compounds. The cytotoxicity assay revealed very low toxicity for all the test compounds against the macrophage cell line. According to the docking study, 2,5-disubstituted benzimidazoles exhibit high affinity for an interdomain cleft that plays a key role in the GTP-dependent polymerization of the filamentous temperature-sensitive Z (FtsZ) protein. The ability of different benzimidazoles to impede FtsZ polymerization is reportedly related to their antimycobacterial activity. On the other hand, the 1,2,5-trisubstituted benzimidazoles docked to the N-terminal of the protein, close to the GTP binding domain, and did not show strong binding energies. Overall, 5a, 5b, and 11 proved to be good candidates for in vivo testing to determine their potential for treating tuberculosis.
Tuberculosis, an infectious disease caused by the bacillus Mycobacterium tuberculosis, is the most widespread infectious disease worldwide. In 2018, 1.2 million deaths were attributed to tuberculosis and 10 million new cases emerged, according to the World Health Organization (WHO). Thus, it poses a serious threat to global health. M. tuberculosis is the deadliest infectious agent and one of the top 10 causes of mortality worldwide (World Health Organization (WHO), 2019). The appearance and spread of drug-resistant strains of this bacterium is an important reason for the reemergence of tuberculosis. The treatment of drug-resistant M. tuberculosis requires combinations of second- and third-line drugs. Apart from being less effective, these drugs are more expensive and more toxic. Hence, there is an urgent need to develop new compounds that can provide better results against susceptible and resistant tuberculosis strains.
The relevant strategies for developing new antimycobacterial drugs (Rode et al., 2019) are comprised of two approaches:
the chemical modification of a known molecule to enhance its biological activity (Thiagarajan et al., 2013; Sutherland et al., 2018), and the design and development of new molecules with antimycobacterial activity (Goverdhan et al., 2015; Lokesh et al., 2016). Within the framework of the former, the benzimidazole system is a scaffold for the development of new organic compounds with potential biological effects. Benzimidazoles have demonstrated antiulcer (Patil et al., 2008), anthelmintic (Dubey and Sanyal, 2010), antihypertensive (Naik et al., 2010), anticoagulant (Mederski et al., 2004), anti-inflammatory (Mader et al., 2008), antimicrobial (Lam et al., 2014), and antifungal (Alp et al., 2009) activity. The antimycobacterial effect of benzimidazole derivatives is also well-documented (Kazimierczuk et al., 2005; Stanley et al., 2012; Gong et al., 2014). An interesting review was published recently about promising antimycobacterial candidates formed by using a range of substituents at the 1-, 2-, 5-, and 6-positions of the benzimidazole core (Keri et al., 2016). An increase in the biological activity of benzimidazole has been achieved by making substitutions with electron-withdrawing groups (Klimesová et al., 2002; Pytela and Klimešová, 2011; Roh et al., 2017).
Although the mechanism of action of benzimidazole is not well understood, at least three plausible hypotheses have been made. Firstly, benzimidazoles are structural isosteres to purines (Arjmand et al., 2005) and thus may inhibit the biosynthesis of nucleic acids and proteins by competing with these heterocycles, leading to the death of bacterial cells (Zhang et al., 2013). Secondly, these derivatives can efficiently inhibit bacterial topoisomerases (Nimesh et al., 2014; Liu et al., 2018). Thirdly, the filamentous temperature-sensitive Z (FtsZ) protein, a tubulin homolog (van den Ent et al., 2001), has been explored as a molecular target for benzimidazoles, finding that the antimycobacterial activity of different benzimidazoles is related to their ability to impede FtsZ polymerization (Awasthi et al., 2013; Park et al., 2014).
The aim of the present study was to synthesize two series of 2,5-disubstituted benzimidazoles, including one previously reported compound, and test them for antimycobacterial activity. The six test compounds all bear electron-withdrawing groups. We hypothesized that an increase in the lipophilicity of the lead compound could enhance its biological activity since the M. tuberculosis cell wall is rich in lipids (Daffé, 2015). Accordingly, a linear aliphatic chain of 16 carbons was chemically linked to the N-1 position of the 2,5-disubstituted benzimidazoles to generate the series of 1,2,5-trisubstituted benzimidazoles. After being screened with an in vitro evaluation of their capacity to inhibit the M. tuberculosis H37Rv strain, the three best compounds were tested on a mouse macrophage cell line infected with M. tuberculosis. Because some of the benzimidazoles demonstrated antimicrobial activity against M. tuberculosis, the potential mechanism of action was explored by molecular docking simulations.
Benzimidazoles are recognized as an excellent platform for the development of new molecules with potential biological activity. Taking into account that the new molecules should have a simple, easily-prepared chemically structure, the test compounds were herein synthesized by environmentally friendly methods based on short and efficient synthesis procedures.
Among the reported methods for obtaining benzimidazoles, some utilize harsh (Gobis et al., 2015; Aghapoor et al., 2019) and others soft reaction conditions (Leutbecher et al., 2011; Sriramoju et al., 2018). Whereas, the former is carried out at a high temperature and with strong acids, the second is performed at or near room temperature (rt) in the presence of soft acids, mainly through heterogeneous catalysis. The first series of benzimidazoles herein synthesized was elaborated in two stages with a short, efficient, and sustainable method. The N-alkylation reaction used to form the second series required a longer synthetic procedure and led to low yields.
The procedure published by Abdelhamid et al. (1988) was modified to synthesize the new benzimidazoles, 5a and 5b. In order to substitute a nitro group at the 5-position of 3a and 3b (containing Cl and Br, respectively), the two 2-thiophenehydroxymoyl halides were treated with 4-nitro-1,2-phenylenediamine [4] in dry DMF at 80°C for 6 h (Scheme 1). Then the 2,5-disubstituted benzimidazoles were N-alkylated with hexadecyl methanesulfonate in basic medium at 120°C for 4 h to provide the 1,2,5-trisubstituted benzimidazoles (7a and 7b) (Scheme 1).
Scheme 1. Synthesis of benzimidazoles 5a/5b and 7a/7b by using 2-thiophenhydroxymoyl halides 3a/3b as key intermediates.
2-Thiophenecarboxaldehyde oxime 2 was synthesized following the procedure described by Iwakura et al. (1968) to give the desired product as a white solid in 93% yield (mp 137–138°C, reported as 136°C) (Iwakura et al., 1968). In its FT-IR spectrum, oxime 2 showed a strong and broad band at 3,025 cm−1 attributed to the NO-H bond, and a band at 1632 due to the C=NOH double bond (Figure S1). In its 1H NMR spectrum, the compound exhibited two singlets at 11.68 and 7.68, corresponding to the hydroxyl and oxime protons, respectively, and three doublets of doublets at 7.54, 7.35, and 7.05 ppm assigned to H5, H3, and H4, respectively (Figure S2). In its 13C NMR spectrum, it displayed signals at 139.4, 131.0, 130.2, 130.1, and 125.6 ppm (Figure S3). The 1H NMR data were similar to those in the literature (Langa et al., 2000). On the other hand, 5-chlorothiophene-2-carbohydroximoyl chloride (3a) and 5-bromothiophene-2-carbohydroxamoyl bromide (3b) were synthesized with a modified protocol of Kanemasa (Kanemasa et al., 2000) by using 3 molar equivalents of N-chlorosuccinimide (NCS) or N-bromosuccinimide (NBS) relative to the oxime. Compound 3a was afforded as a yellow solid in 95% yield (mp 129.5°C, similar to the 127–128°C reported by Iwakura et al.) (Iwakura et al., 1968), and 3b was furnished as a brown oil in 93% yield.
One of the new benzimidazoles, 5a, was obtained as an amorphous beige solid in 60% yield (mp 262–263°C). In the FT-IR spectrum of 5a, there were strong bands at 3,253, 1,576, 1,504, and 1,338 cm−1, corresponding to the H-N, C=N, and NO2 groups, respectively (Figure S4). In its 1H NMR spectrum, the benzimidazole ring generated a doublet at (δ) 8.44 (J = 2.4 Hz for H4), a doublet of doublets at 8.17 (J = 8.7 and 2.4 Hz for H6), and a doublet at 7.72 (J = 9.7 Hz for H7), while the thiophene system produced two doublets at 7.76 and 7.19 (J = 3.9 Hz) for and , respectively (Figure S5). In the 13C NMR spectrum, resonances for 11 aromatic carbons were observed at δ (ppm): 150.5, 144.5, 132.6, 128.9, 128.2, 119.7, 119.5, 118.8, 115.8, 112.1, and 108.5 (Figure S6). The LR-MS spectrum of benzimidazole 5a revealed the expected pattern of molecular ions at 279 M and 281 M+2. The HR-MS spectrum allowed for the determination of an exact experimental mass of 279.9811 (M+H) (Figure S7) and an exact calculated mass of 279.9947 (M+H), thus confirming the structure of benzimidazole 5a (Figure S8).
The other new benzimidazole, 5b, was provided as an amorphous brown solid in 98% yield (mp 254–255°C). In the FT-IR spectrum of 5b, the strong and broad band at [υ (cm−1)] 3,385 cm−1 corresponded to the N-H bond, and the strong bands at 1,625, 1,573, and 1,337 cm−1 to the C=N and NO2 groups (Figure S9). The 1H NMR spectrum showed a singlet [δ (ppm)] at 8.38 (for H4), a doublet at 8.09 (J = 7 Hz for H6), a singlet at 7.70 (for H7 and ), and another singlet at 7.38 (for ) (Figure S10). In the 13C NMR spectrum, only nine signals were found [δ (ppm)], at 150.1, 142.8, 133.9, 132.0, 128.9, 118.2, 116.1, 114.4, and 111.8] (Figure S11). In the LR-MS spectrum, 5b displayed molecular ions at 323 and 325 due to the presence of a bromine atom (Figure S12). Based on the HR-MS spectrum, an exact experimental mass of 323.0410 was established, as was the exact calculated mass of 322.9364 (Figure S13).
The 1,2,5-trisubstituted benzimidazoles 7a and 7b were obtained by N-alkylation with the hexadecyl methanesulfonate in basic medium by heating at 124°C for 3 h, giving the regioisomer 7a as a beige amorphous solid in 35% yield (704 mg) (mp 94–95°C). Its IR (KBr) spectrum exhibited the following absorption bands [υ(cm−1)]: two very strong bands at 2,916 and 2,848 corresponding to the aliphatic chain, a weak band at 1,520 owing to C=N, and bands at 1,472 and 1,462 attributed to the NO2 group. The 1H NMR spectrum showed the following signals at δ (ppm): a singlet signal at 8.31 for H4, a broad doublet of doublets at 8.21, the coupling constant (J = 10 Hz) for H5, and three doublets at 7.79 (J = 10 Hz), 7.41, and 7.05 (J = 5 Hz) assigned to H7, H3, and H4, respectively. Additionally, the triplet at 3.62 (J = 5.0 Hz) was ascribed to the methylene protons next to the benzimidazole nitrogen, a very broad singlet at 1.33–1.24 associated with protons of the aliphatic chain, and finally a triplet signal at 0.86 (J = 5 Hz) corresponding to protons of the methyl group (Figure S14).
The regioisomer 7b was furnished as a beige amorphous solid in 30% yield (202.9 mg) (mp 93–94°C). In the IR (KBr) spectrum, the absorptions bands [υ (cm-1)] consisted of: two very strong bands at 2,917 and 2,850 due to C-H of the aliphatic chain, a weak band at 1,614 owing to C=N, and two bands at 1,470 and 1,331 caused by the NO2 group. The 1H NMR spectrum displayed the following signals at δ (ppm): a broad singlet at 8.39 attributed to H4, a broad triplet of doublets at 8.28 (J = 5 and 10 Hz) assigned to H5, a doublet at 7.88 (J = 10 Hz) corresponding to H7, two doublets at 7.73 and 7.69 (J = 5 Hz) ascribed to and , a triplet at 3.98 (J = 5 Hz) owing to H2CN, a very broad singlet from 1.41–1.31 due to aliphatic chain protons, and finally, a triplet at 0.93 (J = 5 Hz) produced by methyl group protons (Figure S15).
Benzimidazoles 11 and 12 were synthesized by adopting recently published environmentally friendly protocols (Cardozo et al., 2015) with 9 as a key intermediate. Thus, nitro compound 8 was reduced with sodium metabisulfite in aqueous medium and heated to reflux to give 4-chloro-1,2-phenylenediamine 9. Compound 9 was obtained as an amorphous beige solid (mp 173–174°C) (Park et al., 2014) in quantitative yield. The spectroscopic data were similar to results documented in the literature (Cantillo et al., 2013). 4-Chloro-1,2-phenylenediamine 9 (without purification) was reacted with 3-nitrobenzaldehyde 10 and bentonite clay, the latter as a heterogeneous catalyst, under constant stirring at rt (Scheme 2) (Mckinney et al., 1962; Naeimi and Alishahi, 2014). 2-(3′-Nitrophenyl)-5-chlorobenzimidazole (11) was produced as an amorphous beige solid in 64% yield (mp 243–244°C) as described by Keurulainen et al. (2010) (mp 243°C).
Scheme 2. Synthesis of benzimidazoles 11 and 12 with bentonite clay serving as a heterogeneous catalyst.
Its FT-IR spectrum showed strong bands at υ: 3,322, 1,513, and 1,347 cm−1, corresponding to the N-H and NO2 groups. In the 1H NMR spectrum of benzimidazole 11, the phenyl group exhibited triplets (δ) at 8.96 (J = 1.2 Hz for ) and 7.82 (J = 7.8 Hz for ), and doublets of triplets at 8.55 (J = 7.8 and 1.2 Hz for ) and 8.31 (J = 7.8 and 1.2 Hz for ). On the other hand, the protons of the benzimidazole ring generated a triplet at (δ) 7.64 (J = 9.0 Hz for H4 and H7) and a doublet of doublets at 7.24 (J = 8.4 Hz for H6). In the 13C NMR spectrum, there were eight resonances for 13 carbons. The LR-MS spectrum of benzimidazole 11 displayed the expected pattern with molecular ions at 273 (M) and 275 (M+2). Spectroscopic and spectrometric properties were similar to those reported previously (Keurulainen et al., 2010).
The trisubstituted benzimidazole 12 was prepared by N-alkylation of 11 in hexadecyl methanesulfonate 6 basic medium and heating at 120°C for 4 h to provide an amorphous beige solid in 18% yield (36 mg) (mp 95–96°C). In the 1H NMR spectrum, the following signals can be appreciated at δ (ppm): a broad singlet at 8.59 assigned to , a broad doublet of doublets at 8.39, (J = 5 Hz) attributed to , two broad doublets at 8.13 (J = 5 Hz) and 7.81 (J = 5 Hz) ascribed to and H4, respectively, a triplet at 7.75 (J = 5 Hz) corresponding to , a triplet at 7.36 (J = 10 Hz) and 7.34 (10 Hz) owing to and H7, a triplet at 3.64 (J = 5 Hz) associated with the methylene protons next to the benzimidazole nitrogen, a quintuplet at 1.57 (J = 5 Hz) due to β-methylene protons close to the benzimidazole nitrogen, a very broad singlet at 1.26 caused by protons of the aliphatic chain, and finally, a triplet at 0.88 (J = 5 Hz) generated by methyl group protons (Figure S16).
The benzimidazole scaffold is attractive for drug design because of the diverse activities of its derivatives. Benzimidazoles substituted with electron-withdrawing groups, such as nitro (Pytela and Klimešová, 2011; Roh et al., 2017) and halogen groups (Cl, F, and Br), have shown enhanced biological activity (Klimesová et al., 2002). The disubstituted and trisubstituted benzimidazole molecules herein synthesized were evaluated for their in vitro antimicrobial effect on M. tuberculosis H37Rv. The in vitro data revealed that the 2,5-disubstituted benzimidazoles were more efficient than the 1,2,5-trisubstituted compounds in their capacity to kill the mycobacterium. The benzimidazole derivatives capable of effectively inhibiting the growth of M. tuberculosis were 5a, 5b, and 11, with MIC values of 89.6, 19.4, and 22.9 nM, respectively (Table 1). Contrarily, low antimycobacterial activity was detected for the molecules trisubstituted at the 1-position with the aliphatic chain, 7b, 7a, and 12, with MIC values of >182, >198, and >201 nM, respectively.
Based on the in vitro results, the toxicity of 5a, 5b, and 11 was tested on murine macrophages infected with M. tuberculosis (Figure 1). This intracellular bacillus mainly resides in alveolar macrophages and type II pneumocytes. Although only moderate antimycobacterial activity was found for 5a in vitro (25 μg/mL MIC), it displayed a good intracellular effect. After 48 h of treatment, only 10% of mycobacteria were viable, demonstrating the inhibition of mycobacterial replication, similar to the type of activity reported for isoniazid. Compounds 5b and 11 exhibited a moderate intracellular effect. After exposure to 5b and 11, only 30% of the mycobacteria could recover. Hence, these compounds also impeded bacterial replication, and their toxicity toward mycobacteria was lower than that of 5a. The data published for 11 on the inhibition of the intracellular C. pneumoniae are similar to the current findings (Keurulainen et al., 2010).
Figure 1. Survival of intracellular mycobacteria in macrophages treated with each of the three 2,5-disubstituted benzimidazole derivatives. In independent assays, macrophages were infected for 2 h and one of the benzimidazole derivatives or isoniazid was added. Intracellular growth was then monitored at 96 h post-infection. The data are expressed as the mean ± standard error of two measurements.
For the cytotoxicity assays, macrophages were exposed to the benzimidazole derivatives at concentrations higher than the MIC for 24 h. Subsequently, the viability of the cells was determined with Alamar Blue. None of the compounds showed any significant cytotoxic effect on macrophages (Figure 2). Even 5a, with the lowest viability (86%), is not precluded from being used as an antimycobacterial agent.
Figure 2. Cytotoxicity was evaluated for the 2,5-disubstituted benzimidazole derivatives on macrophages. In independent assays, macrophage monolayers were exposed during 24 h to 50 μg/ml of each one of the benzimidazole solutions, 40 μg/ml ursolic acid as the death control, or left untreated for the viability control. Values represent the mean ± standard deviation of two independent experiments. No significant differences were found between benzimidazole derivatives.
Since nitro and halogen groups are known to enhance the biological activity of pharmacophores (Salar et al., 2017), examination was presently made of the effect of different electron-withdrawing groups (2-haloheteroaryl or nitroaryl, 5-chloro or nitro) at positions two and five of the benzimidazole core. The disubstituted (5a, 5b, and 11) but not the trisubstituted (7a, 7b, and 12) benzimidazoles produced good antimycobacterial activity against M. tuberculosis. Compounds 5b and 11 induced a better effect than 5a (19.4 nM and 22.9 nM, respectively, versus 89.6 nM). The former two molecules each contain two electron-withdrawing groups, and a cooperative effect between them may explain the robust antimycobacterial activity. In relation to the two electron-withdrawing groups of 5b, there is one bromo at the C-5′ position of the 2-(thien-2′-yl) and a nitro group at the C-5 position of the benzimidazole ring. On the other hand, 11 bears a nitro group at the C-3′ position of 2-(p-3'-nitrophenyl-1'-yl) and a chloro group at the C-5 position of the benzimidazole ring. Compound 5a, an analog of 5b, has a chloro group at the C-5'position of 2-(thien-2'-yl) instead of a bromo group, and a nitro group at the C-5 position of benzimidazole ring. Its lower antimycobacterial potency possibly owes itself to a less efficient cooperative effect of the substituents against M. tuberculosis. The charge of the cell wall of M. tuberculosis is negative (Hoque et al., 2012; Phillips et al., 2017), while that of the 2,5-disubstituted benzimidazoles is likely to be positive, due to the acceptor ability of these compounds for nitrogen atoms. Hence, another plausible hypothesis about the efficient antimycobacterial activity of the 2,5-disubstituted benzimidazoles is their potential ability to interact with the cell wall of mycobacteria through electrostatic charges.
In the case of 1,2,5-trisubstituted benzimidazoles, we hypothesized that the long aliphatic chain of sixteen carbon atoms bonded on the 1-position of the benzimidazole ring might improve the non-covalent intermolecular interaction with the highly lipophilic cell wall of the mycobacterium (Daffé, 2015), leading to an increase in antimycobacterial activity. According to the current data, however, the introduction of the aliphatic chain was detrimental to such activity. Similarly, the alkyl chains in novel aminopyrimidinyl benzimidazole were reported to reduce antibacterial potency (Liu et al., 2018).
The difference in the biological activity between the two series of benzimidazoles may stem from their distinct solubility properties. The 2,5-disubstituted benzimidazoles were soluble in dimethylsulfoxide and the 1,2,5-trisubstituted benzimidazoles in chloroform, owing to the strong influence in the latter compounds of a hydrophilic/hydrophobic imbalance, being predominant the hydrophobic effect. In this sense, a shorter aliphatic chain for the 1,2,5-trisubstituted benzimidazoles could possibly generate better antimicrobial activity against M. tuberculosis, considering greater equilibrium in the hydrophobic/hydrophilic effect.
In order to explore the target of the two series of compounds and provide insights into why they showed disparate antimycobacterial effects, molecular docking was carried out on the FtsZ protein of M. tuberculosis. This docking study was based on the binding site previously reported by Li et al. (2017). The protein subunit consists of two globular N- and C-terminal subdomains linked by a central H7 helix having an adjacent T7 loop. FtsZ, a prokaryote tubulin homolog protein, plays a major role in the cytokinesis of mycobacteria. In the presence of guanosine triphosphate (GTP), FtsZ polymerizes to form a dynamic Z-ring inside the cell on the inner membrane and then recruits other cell division proteins (Hong et al., 2013). There is evidence that the disturbance of proper FtsZ assembly would block septum formation and consequently prevent cell division, which should lead to the inhibition of bacterial growth and the promotion of cell death (Slayden et al., 2006; Respicio et al., 2008; Schaffner-Barbero et al., 2012).
Molecular docking was performed on the six test compounds, which had been found to exhibit different antimycobacterial activity. The compounds were docked to get the best molecular confirmations in the interdomain cleft, which is crucial to GTP-dependent polymerization of the FtsZ protein. The binding energy and H-bonding were taken as two important parameters of the interactions (Figure 3).
Figure 3. (A) Docking score of the binding energy and analysis of the amino acids involved in the interaction. (B) Binding pose for the six compounds within the interdomain cleft of FtsZ. Whereas, 5a, 5b, and 11 docked into the interdomain cleft site, showing two hydrogen bonds for 11 and one for 5a and 5b (red line), 7a, 7b, and 12 docked near the GTP binding site. (C) Close-up of the binding conformations of compounds 5a, 5b, and 11, which all adopted a similar structure when docking at the interdomain cleft of the FtsZ protein. (D) Close-up of the binding domain of compounds 7a, 7b, and 12. They docked between Helix 4 and H5 of the FTsZ protein with a less favorable binding energy than the 2,5-disubstituted compounds. The hydrogen bonds are represented by red lines.
Based on the molecular docking study, the three 2,5-disubstituted benzimidazoles, 5a, 5b, and 11, adopt a similar arrangement. The halogens are exposed to FtsZ and the hydrophobic groups interact with the T7 loop of the protein. Two hydrogen bonds were detected for 11 and only one for 5a and 5b. Thus, the former showed a better binding energy (−6.2 kcal/mol). The main amino acids in the protein that established hydrogen bonds with the benzimidazoles were I 288 and D 290 (Figure 3). The molecular docking results for 5a, 5b, and 11 are consistent with previous reports. The benzimidazole scaffold and cyclohexyl group were observed inside the hydrophobic environment, with the halogen groups exposed to the cleft of FtsZ (Ding et al., 2016).
Contrarily, the three 1,2,5-trisubstituted derivatives containing hydrocarbon chains, 7a, 7b, and 12, do not dock at the same domain nor show strong affinity. Rather, they dock to the N-terminal side of the protein close to the GTP binding domain. Structurally, they interact with the domain formed by helices four and five (H4 and H5). Because of their size, they are not able to position themselves in any cavity of the protein. Both binding affinity and docking results correlated with the antimicrobial effective dose.
4-Nitro-1,2-phenylenediamine, 4-chloro-2-nitroaniline, 2-thiophenecarboxaldehyde, 3-nitrobenzaldehyde, 2-pyridinecarboxaldehyde, N-bromosuccinimide, and N-chlorosuccinimide (Sigma-Aldrich, St. Louis, MO, USA), were used for syntheses without additional purification. The organic solvents were purified by the standard methods. The progress of each reaction was followed by thin-layer chromatography (TLC) on silica plates (silica gel 60 F254, Merck) and with UV light, iodine vapor, or an acidic aqueous solution of KMnO4 for visualization. Column chromatography was performed on silica gel (70–230 mesh, Sigma-Aldrich) with freshly distilled hexane/ethyl acetate as the eluent. Dimethylformamide (DMF) was dried with CaH2 at reflux. Acetonitrile was dried with phosphorous pentoxide under constant stirring at rt. Melting points were determined on an electrothermal melting point apparatus. Infrared spectra (FT-IR) were recorded on a double-beam Perkin-Elmer Model 1605 FT-IR spectrometer. NMR spectra were recorded in acetone-d6, DMSO-d6, or CDCl3 solution on Gemini 200, Eclipse 300, or Agilent 500 spectrometers operating at 200, 300, and 500 MHz for 1H NMR and 50, 75, and 125 MHz for 13C NMR, respectively. Chemical shifts are described in parts per million (ppm), employing Me4Si as the internal standard. Coupling constants (J) are expressed in Hz. Mass spectra were recorded on a double-beam JEOL JMS AX505HA spectrometer in the electron impact mode and high-resolution mass spectrometry (HR-MS) with electrospray ionization (Bruker Daltonics, Billerica, MA, USA). All experiments were carried out at rt.
The benzimidazole derivatives were synthesized by two different chemical pathways, one of which was a modification of the method proposed by Abdelhamid et al. (1988), who used phenylenediamine or phenylenediamine with electron-donating substituents. Briefly, 2-thiophenebenzaldoxime (2) was synthesized by stirring a mixture of 2-thiophencarboxaldehyde and hydroxylamine hydrochloride in basic medium for 2 h. Subsequently, 2 was treated with NCS or NBS in dry DMF at 15–20°C for 2 h to form 3a or 3b.
For the synthesis of the new benzimidazoles, the key intermediate (2-thiophenhydroxymoyl halides 3) was reacted without purification with 4-nitro-1,2-phenylenediamine (4) in dry DMF at 80°C for 6 h to obtain 5a and 5b. The novel benzimidazoles 7a, 7b, and 12 were synthesized by N-alkylation of 5a, 5b, and 11 with hexadecyl methanesulfonate 6 and sodium bicarbonate in dry acetonitrile and heating at reflux for 4 h.
The second method was only for the synthesis of 11 and 12. 4-Chloro-1,2-phenylenediamine 9 served as a key intermediate, prepared from the reduction of 4-chloro-2-nitroaniline 8 with sodium metabisulfite (Mckinney et al., 1962; Naeimi and Alishahi, 2014). Compound 9 was mixed with 3-nitrobenzaldehyde 10 in dry acetonitrile at rt, using bentonite clay as a heterogeneous catalyst, to give 11 (Cardozo et al., 2015). The latter was N-alkylated with hexadecyl methanesulfonate 6 basic medium and heated at 120°C for 4 h to afford the trisubstituted benzimidazole 12.
The mycobacterial effect of benzimidazole derivatives was evaluated on the M. tuberculosis H37Rv strain by performing microcolorimetric assays with Alamar Blue dye, according to Collins and Franzblau (1997). Briefly, the four benzimidazoles at concentrations in the range of 200–3.125 μg/mL were tested in 96-well sterile microplates. Isoniazid was included as the reference drug. Stock solutions of the benzimidazoles were prepared in dimethylsulfoxide at 20 mg/mL in sterile conditions and stored at −70°C to await further use. Standard solutions were obtained by consecutive twofold dilutions of the stock solutions in 7H9 Middlebrook medium until reaching 800 μg/mL, followed by the addition of 100 μL of Middlebrook 7H9 broth, 100 μL of the benzimidazole solution and 100 μL of mycobacterial suspension to each well. A 1:10 dilution was made for the control from the bacterial suspension, representing the growth of 10% of the bacterial population assayed. After incubating the plates at 37°C for 5 days, 20 μL of Alamar Blue solution (AbD Serotec) and 12 μL of sterile 10% Tween 80 were added. The plates were incubated at 37°C for another 24 h before the determination was made, considering wells with a pink color darker than that of the 10% control well to be positive for growth. The minimal inhibitory concentration (MIC) was defined as the lowest at which benzimidazole impeded development of the pink color.
In order to examine the intracellular antimycobacterial activity, monolayers of mouse macrophages (J774A.1) were infected and then treated in independent assays with each benzimidazole or isoniazid. Macrophage monolayers were prepared by utilizing 2.5 × 105 cells/mL in RPMI-1640 medium (Sigma-Aldrich) supplemented with 10% fetal bovine serum (HyClone®, Thermo SCIENTIFIC) and placed in 24-well plates. The plates were incubated at 37°C in a humidified 5% CO2 atmosphere until the monolayers reached 90% cell confluency. Subsequently, the monolayers were washed with Hanks' balanced salt solution (HBSS) to remove the non-adhered cells. To infect the cells, the bacterial suspensions were adjusted to a multiplicity of infection (MOI) ratio of 1:10, and 1 mL of the suspension was added to each well. After infected monolayers were incubated at 37°C under 5% CO2 for 2 h, monolayers were washed three times with HBSS to eliminate the bacteria not internalized, followed by the addition of a 1-mL solution of one of the compounds or isoniazid diluted in RPMI-1640 medium. Whereas, all test compounds were evaluated at MIC, +MIC, and –MIC, isoniazid was assayed at 0.5, 0.25, and 0.125 μg/mL. Untreated infected cells were included as controls. The cells were incubated at 37°C under 5% CO2, and the kinetics of the activity was monitored at 24, 48, 72, and 96 h. At each time point, cells were lysed by the adding of 500 μL of 0.25% SDS and neutralized with 500 μL of 5% bovine serum albumin (BSA) solution. Serial dilutions of the cell lysates were plated on 7H11 Middlebrook agar supplemented with 10% OADC (oleic acid, albumin, dextrose and catalase) and incubated at 37°C for 3 weeks to determine the number of colony-forming units (CFUs).
In order to assess the possible toxicity of benzimidazole derivatives to macrophages, a viability assay was performed. Macrophage monolayers were prepared as aforementioned (in subsection intracellular activity of the benzimidazoles) up to washing with HBSS to remove the non-adhered cells. Subsequently, 1 mL of the 50 μg/mL solution of one of the benzimidazoles was added. Some wells were maintained without treatment (viability control), and to others were added 40 μg/mL ursolic acid (death control). The cells were incubated for 24 h at 37°C under 5% CO2. Upon completion of this time, 100 μL of Alamar Blue solution was added, the monolayers were incubated again until the viability control wells turned pink. The relative fluorescence units were quantified in a Fluoroskan Ascent FL Microplate fluorometer, and the RFUs of the control wells without treatment were taken as 100% viability.
The molecular docking simulations were carried out on Autodock Vina 4.2.6, which has been calibrated and tested with molecular dynamics by using a set of small-protein ligands of medicinal interest (Perryman et al., 2015; Forli et al., 2016). This software maximizes the accuracy of predicting the binding of small molecules to proteins, assessing the veracity of the predictions by comparing them to the experimental structure. Evaluation of the difference between the experimental and predicted structures considers symmetry, partial symmetry, and near-symmetry (Trott and Olson, 2010).
The X-ray structure of M. tuberculosis eukaryotic tubulin homolog protein FtsZ (2.08Å resolution, PDBID 1RLU) (Leung et al., 2004) was obtained from the Protein Data Bank database (http://www.pdb.org). The three-dimensional structures of the ligands were built and their energy minimized with MarvinSketch 18.24.0 ChemAxon software (http://www.chemaxon.com). The ligands and protein were then prepared for molecular docking by adding hydrogens and Gasteiger charges with Autodock tools 1.5.6 (Sanner, 1999). A grid box of 30 Å on each side was placed into the dimer interdomain with grid points separated by 1.0 Å centered at the middle of the protein at x = 6.0, y = 29, and z = 27. The binding energies of the docked complexes were provided by the output Autodock Vina file and the ligand-protein binding interactions at the binding sites were analyzed on UCFS Chimera software (Pettersen et al., 2004).
The new 2,5-disubstituted benzimidazole derivatives were successfully synthesized by simple, efficient and inexpensive procedures. The synthetic procedure for the 1,2,5-trisubstituted compounds was longer and led to low yields. The structures of all test compounds were fully characterized by FT-IR, NMR, and HR-MS. According to the in vitro antimycobacterial assays, the 2,5-disubstituted benzimidazoles exhibited excellent antimycobacterial activity. After adding the hydrocarbon chain in the 1-position of the benzimidazole ring to form the 1,2,5-trisubstituted benzimidazoles, contrarily, a poor antimycobacterial effect was found. The docking analysis of the possible molecular target and mechanism of action of the test compounds suggests that the 2,5-disubstituted benzimidazoles could effectively interact with a crucial domain for the polymerization of the FtsZ protein. On the other hand, the larger molecular size of the 1,2,5-trisubstituted benzimidazoles apparently caused steric restriction, impeding their approach to the putative binding site of the FtsZ protein to block septum formation and cell division. Rather, the 1,2,5-trisubstituted derivatives docked to N-terminal side of the protein, closer to the GTP binding domain. Overall, these results point to the promising antimycobacterial activity of 2,5-disubstituted benzimidazoles. Further in vivo studies are needed to determine their potential for treating tuberculosis.
RJ-J and BG-P conceptualized and designed the study. RJ-J, NJ-R, GC-R, IU-G, and GM-M carried out the chemical synthesis and characterization of the new compounds. WC-C, RR-N, and NC-J performed the biological activity assays. CA-A was responsible for the docking study. RJ-J, BG-P, NC-J, and CA-A analyzed the results of the investigation. RJ-J, NC-J, CA-A, and BG-P took charge of writing the original draft, which was analyzed for modification by all authors. BG-P edited the following versions of the manuscript, administered the project, and acquired funding.
This research was financially supported by grants from the Proyecto aprobado por el Fondo Sectorial de Investigación para la Educación CONACyT (222001) and the Secretaría de Investigación y Posgrado, Instituto Politécnico Nacional (SIP/IPN 20181506, 20181396, 20196609, 20196611, 20201009, 20201440).
The authors declare that the research was conducted in the absence of any commercial or financial relationships that could be construed as a potential conflict of interest.
RJ-J and BG-P received fellowships from COFAA, EDI, and SNI. CA-A and NC-J are members of the Sistema Nacional de Investigadores (SNI). Molecular graphics were made and analyses performed with the UCSF Chimera package. Chimera is developed by the Resource for Biocomputing, Visualization and Informatics at the University of California, San Francisco (supported by NIGMS P41-GM103311).
The Supplementary Material for this article can be found online at: https://www.frontiersin.org/articles/10.3389/fchem.2020.00433/full#supplementary-material
Abdelhamid, A. O., Párkányi, C., Rashid, S. M. K., and Lloyd, W. D. (1988). Synthesis of fused ring heterocycles from aromatic amines with hydroximoyl chlorides. J. heterocycle. Chem. 25, 403–405.
Aghapoor, K., Mohsenzadeh, F., Darabi, H. R., and Jalali, M. R. (2019). ZnCl2/urea eutectic solvent as stable carbonylation source for benign synthesis of 2-benzimidazoles and 2-imidazolones: an effective strategy for preventing NH2 gas evolution. ChemistrySelect 4, 11093–11097. doi: 10.1002/slct.201902706
Alp, M., Göker, H., Brun, R., and Yildiz, S. (2009). Synthesis and antiparasitic and antifungal evaluation of 2'-arylsubstituted-1H,1'H-[2,5']bisbenzimidazolyl-5-carboxamidines. Eur. J. Med. Chem. 44, 2002–2008. doi: 10.1016/j.ejmech.2008.10.003
Arjmand, F., Mohani, B., and Ahmad, S. (2005). Synthesis, antibacterial, antifungal activity and interaction of CT-DNA with a new benzimidazole derived Cu(II) complex. Eur. J. Med. Chem. 40, 1103–1110. doi: 10.1016/j.ejmech.2005.05.005
Awasthi, D., Kumar, K., Knudson, S. E., Slayden, R. A., and Ojima, I. (2013). SAR studies on trisubstituted benzimidazoles as inhibitors of Mtb FtsZ for the development of novel antitubercular agents. J. Med. Chem. 56, 9756–9770. doi: 10.1021/jm401468w
Cantillo, D., Moghaddam, M. M., and Kappe, O. (2013). Hydrazine-mediated reduction of nitro and azide functionalities catalyzed by highly active and reusable magnetic iron oxide nanocrystals. J. Org. Chem. 78, 4530–4542. doi: 10.1021/jo400556g
Cardozo, V. A., Sánchez-Obregón, R., Salgado-Zamora, H., and Jiménez-Juárez, R. (2015). Bentonite clay: an efficient catalyst for the synthesis of 2-substituted benzimidazoles. Monatsh. Chem. 146, 1335–1337. doi: 10.1007/s00706-015-1423-x
Collins, L., and Franzblau, S. G. (1997). Microplate alamar blue assay versus BACTEC 460 system for high-throughput screening of compounds against Mycobacterium tuberculosis and Mycobacterium avium. Antimicrob. Agents Chemother. 41, 1004–1009.
Daffé, M. (2015). The cell envelope of tubercle bacilli. Tuberculosis 95(Suppl.1), S155–S158. doi: 10.1016/j.tube.2015.02.024
Ding, L., Chi, B., Wei-Wei, W., Jin-Ming, G., and Jian, W. (2016). Exploring the possible binding mode of trisubstituted benzimidazoles analogues in silico for novel drug design targeting Mtb FtsZ. Med. Chem. Res. 26, 153–169. doi: 10.1007/s00044-016-1734-4
Forli, S., Huey, R., Pique, M. E., Sanner, M. F., Goodsell, D. S., and Olson, A. J. (2016). Computational protein-ligand docking and virtual drug screening with the autoDock suite. Nat. Protoc. 11, 905–919. doi: 10.1038/nprot.2016.051
Gobis, K., Foks, H., Serocki, M., Augustynowics-Kopec, E., and Napiórkowska, A. (2015). Synthesis and evaluation of in vitro antimycobacterial activity of novel 1H-benzo[d]imidazole derivatives and analogues. Eur. J. Med. Chem. 89, 13–20. doi: 10.1016/j.ejmech.2014.10.031
Gong, Y., Somersan Karakaya, S., Guo, X., Zheng, P., Gold, B., Ma, Y., et al. (2014). Benzimidazole-based compounds kill Mycobacterium tuberculosis. Eur. J. Med. Chem. 75, 336–353. doi: 10.1016/j.ejmech.2014.01.039
Goverdhan, S., Perumal, Y., Dharmarajan, S., and Srinivas, K. (2015). Rational design, synthesis and evaluation of novel-substituted 1,2,3-triazolylmethyl carbazol as potent inhibitors of Mycobacterium tuberculosis. Med. Chem. Res. 24, 1298–1309. doi: 10.1007/s00044-014-1210-y
Hong, W., Deng, W., and Xie, J. (2013). The structure, function, and regulation of Mycobacterium FtsZ. Cell. Biochem. Biophys. 6597:105. doi: 10.1007/s12013-012-9415-5
Hoque, J., Akkapeddi, P., Yarlagadda, V., Uppu, D. S., Kumar, P., and Haldar, J. (2012). Cleavable cationic antibacterial amphiphiles: synthesis, mechanism of action, and cytotoxicities. Langmuir 28, 12225–12234. doi: 10.1021/la302303d
Iwakura, Y., Uno, K., Shiraishi, S., and Hongu, T. (1968). 1,3-Dipolar cycloaddition reaction of thiophenecarbonitrile N-oxide with various dipolarophiles. Bull Chem. Soc. Jpn. 41, 2954–2959. doi: 10.1246/bcsj.41.2954
Kanemasa, S., Matsuda, H., Kamimura, A., and Kakinami, T. (2000). Synthesis of hydroximoyl chlorides from aldoximes and benzyltrimethylammonium tetrachloroiodate (BTMA ICl4). Tetrahedron 56, 1057–1064. doi: 10.1016/S0040-4020[99]01047-9
Kazimierczuk, Z., Andrzejewska, M., Kaustova, J., and Klimesova, V. (2005). Synthesis and antimycobacterial activity of 2-substituted halogenobenzimidazoles. Eur. J. Med. Chem. 40, 203–208. doi: 10.1016/j.ejmech.2004.10.004
Keri, R. S., Rajappa, C. K., Patil, S. A., and Nagaraja, B. M. (2016). Benzimidazole-core as an antimycobacterial agent. Pharmacol. Rep. 68, 1254–1265. doi: 10.1016/j.pharep.2016.08.002
Keurulainen, L., Salin, O., Siiskonen, A., Kern, J. M., Alvesalo, J., Kiuru, P., et al. (2010). Design and synthesis of 2-arylbenzimidazoles and evaluation of their inhibitory effect against chlamydia pneumoniae. J. Med. Chem. 53, 7664–7674. doi: 10.1021/jm1008083
Klimesová, V., Kocí, J., Pour, M., Stachel, J., Waisser, K., and Kaustová, J. (2002). Synthesis and preliminary evaluation of benzimidazole derivatives as antimicrobial agents. Eur. J. Med. Chem. 37, 409–418. doi: 10.1016/S0223-5234(02)01342-9
Lam, T., Hilgers, M. T., Cunningham, M. L., Kwan, B. P., Nelson, K. J., Brown-Driver, V., et al. (2014). Structure-based design of new dihydrofolate reductase antibacterial agents: 7-[benzimidazol-1-yl]-2,4-diaminoquinazolines. J. Med. Chem. 57, 651–668. doi: 10.1021/jm401204g
Langa, F., de la Cruz, P., Espíldora, E., Gonzalez-Cortés, A., de la Hoz, A., and López-Arza, V. (2000). Synthesis and Properties of Isoxazolo[60]fullerene–Donor Dyads. J. Org. Chem. 65, 8675–8684. doi: 10.1021/jo0010532
Leung, A. K., Lucile White, E., Ross, L. J., Reynolds, R. C., DeVito, J. A., and Borhani, D. W. (2004). Structure of Mycobacterium tuberculosis FtsZ reveals unexpected, G protein-like conformational switches. J. Mol. Biol. 342, 953–970. doi: 10.1016/j.jmb.2004.07.061
Leutbecher, H., Constantin, M.-A., Mika, S., Conrad, J., and Beifuss, U. (2011). A new laccase-catalysed domino process and its application to the efficient synthesis of 2-aryl-1H-benzimidazoles. Tetrahedron Lett. 52, 604–607. doi: 10.1016/j.tetlet.2010.11.145
Li, D., Chi, B., Wang, W., Gao, J., and Wan, J. (2017). Exploring the possible binding mode of trisubstituted benzimidazoles analogues in silico for novel drug design targeting Mtb FtsZ. Med. Chem. Res. 26, 153–169. doi: 10.1007/s00044-016-1734-4
Liu, H. B., Gao, W. W., Tangadanchu, V. K. R., Zhou, C. H., and Geng, R. X. (2018). Novel aminopyrimidinyl benzimidazoles as potentially antimicrobial agents: Design, synthesis and biological evaluation. Eur. J. Med. Chem. 14, 366–384. doi: 10.1016/j.ejmech.2017.11.027
Lokesh, P., Perumal, Y., Dharmarajan, S., and Srinivas, K. (2016). Click-based synthesis and antitubercular evaluation of novel dibenzo[b,d]thiophene-1,2,3-triazoles with piperidine, piperazine, morpholine and thiomorpholine appendages. Bioorg. Med. Chem. Lett. 26, 2649–2654. doi: 10.1016/j.bmcl.2016.04.015
Mader, M., de Dios, A., Shih, C., Bonjouklian, R., Li, T., White, W., et al. (2008). Imidazolyl benzimidazoles and imidazo (4,5-b) pyridines as potent p38alpha MAP kinase inhibitors with excellent in vivo antiinflammatory properties. Bioorg. Med. Chem. Lett. 18, 179–183. doi: 10.1016/j.bmcl.2007.10.106
Mckinney, R. M., Spillane, J. T., and Pearce, G. W. (1962). Amino- and nitrofluorescein derivatives. J. Org. Chem. 27, 3986–3988. doi: 10.1021/jo01058a055
Mederski, W. W., Dorsch, D., Anzali, S., Gleitz, J., Cezanne, B., and Tsaklakidis, C. (2004). Halothiophene benzimidazoles as P1 surrogates of inhibitors of blood coagulation factor Xa. Bioorg. Med. Chem. Lett. 14, 3763–3769. doi: 10.1016/j.bmcl.2004.04.097
Naeimi, H., and Alishahi, N. (2014). An efficient and one-pot reductive cyclization for synthesis of 2-substituted benzimidazoles from o-nitroaniline under microwade conditions. J. Ind. Eng. Chem. 20, 2543–2547. doi: 10.1016/j.jiec.2013.10.038
Naik, P., Murumkar, P., Giridhar, R., and Yadav, M. R. (2010). Angiotensin II receptor type 1 (AT1) selective nonpeptidic antagonists -a perspective. Bioorg. Med. Chem. 18, 8418–8456. doi: 10.1016/j.bmc.2010.10.043
Nimesh, H., Sur, S., Sinha, D., Yadav, P., Anand, P., Bajaj, P., et al. (2014). Synthesis and biological evaluation of novel bisbenzimidazoles as Escherichia coli topoisomerase IA inhibitors and potential antibacterial agents. J. Med. Chem. 57, 5238–5257. doi: 10.1021/jm5003028
Park, B., Awasthi, D., Chowdhury, S. R., Melief, E. H., Kumar, K., Knudson, S. E., et al. (2014). Design, synthesis and evaluation of novel 2,5,6-trisubstituted benzimidazoles targeting FtsZ as antitubercular agents. Bioorg. Med. Chem. 22, 2602–2612. doi: 10.1016/j.bmc.2014.03.035
Patil, A., Ganguly, S., and Surana, S. (2008). A systematic review of benzimidazole derivatives as an antiulcer agent. Rasayan J. Chem. 1, 447–460.
Perryman, A. L., Yu, W., Wang, X., Ekins, S., Forli, S., Li, S. G., et al. (2015). A virtual screen discovers novel, fragment-sized inhibitors of Mycobacterium tuberculosis InhA. J. Chem. Inf. Model. 55, 645–659. doi: 10.1021/ci500672v
Pettersen, E. F., Goddard, T. D., Huang, C. C., Couch, G. S., Greenblatt, D. M., Meng, E. C., et al. (2004). UCSF Chimera–a visualization system for exploratory research and analysis. J. Comput. Chem. 25, 1605–12. doi: 10.1002/jcc.20084
Phillips, D. J., Harrison, J., Richards, S. J., Mitchell, D. E., Tichauer, E., Hubbard, A. T. M., et al. (2017). Evaluation of the antimicrobial activity of cationic polymers against mycobacteria: toward antitubercular macromolecules. Biomacromolecules 18, 1592–1599. doi: 10.1021/acs.biomac.7b00210
Pytela, O., and Klimešová, V. (2011). Effect of substitution on the antimycobacterial activity of 2-[substituted benzyl] sulfanyl benzimidazoles, benzoxazoles, and benzothiazoles–a quantitative structure-activity relationship study. Chem. Pharm. Bull. 59, 179–184. doi: 10.1248/cpb.59.179
Respicio, L., Nair, P. A., Huang, Q., Anil, B., Tracz, S., Truglio, J. J., et al. (2008). Characterizing septum inhibition in Mycobacterium tuberculosis for novel drug discovery. Tuberculosis 88, 420–429. doi: 10.1016/j.tube.2008.03.001
Rode, H. B., Lade, D. M., Grée, R., Mainkar, P. S., and Chandrasekhar, S. (2019). Strategies towards the synthesis of anti-tuberculosis drugs. Org. Biomol. Chem.17, 5428–5459. doi: 10.1039/c9ob00817a
Roh, J., Karabanovich, G., Vlčková, H., Carazo, A., Němeček, J., Sychra, P., et al. (2017). Development of water-soluble 3,5-dinitrophenyl tetrazole and oxadiazole antitubercular agents. Bioorg. Med. Chem. 25, 5468–5476. doi: 10.1016/j.bmc.2017.08.010
Salar, U., Khan, K. M., Taha, M., Ismail, N. H., Ali, B., Qurat-Ul-Ain, P. S., et al. (2017). Biology-oriented drug synthesis (BIODS): In vitro β-glucuronidase inhibitory and in silico studies on 2-(2-methyl-5-nitro-1H-imidazol-1-yl)ethyl aryl carboxylate derivatives. Eur. J. Med. Chem. 5, 1289–1299. doi: 10.1016/j.ejmech.2016.11.031
Sanner, M. F. (1999). Python: a programming language for software integration and development. J. Mol. Graph. Model. 17, 57–61.
Schaffner-Barbero, C., Martín-Fontecha, M., Chacón, P., and Andreu, J. M. (2012). Targeting the assembly of bacterial cell division protein FtsZ with small molecules. ACS Chem. Biol. 7, 269–277. doi: 10.1021/cb2003626
Slayden, R. A., Knudson, D. L., and Belisle, J. T. (2006). Identification of cell cycle regulators in Mycobacterium tuberculosis by inhibition of septum formation and global transcriptional analysis. Microbiology. 152, 1789–97. doi: 10.1099/mic.0.28762-0
Sriramoju, V., Kurva, S., and Madabhushi, S. (2018). Oxame-mediated annulation of 2-aminobenzamides and 1,2-diaminobenzenes with sec-amines via imine-N-oxides: new synthesis of 2,3-dihyquinazolin-4(1H)-ones and 1H-benzimidazoles. New J. Chem. 42, 3188–3191. doi: 10.1039/C7NJ04939K
Stanley, S. A., Grant, S. S., Kawate, T., Iwase, N., Shimizu, M., Wivagg, C., et al. (2012). Identification of novel inhibitors of M. tuberculosis growth using whole cell based high-throughput screening. ACS Chem. Biol. 7, 1377–1384. doi: 10.1021/cb300151m
Sutherland, H. S., Tong, A. S. T., Choi, P. J., Conole, D., Blaser, A., Franblau, S. G., et al. (2018). Structure-Activity relationships for analogs of the tuberculosis drug bedaquiline with the napfthalene unit replaced by bicyclic heterocycles. Bioorgan. Med. Chem. 26, 1797–1809. doi: 10.1016/j.bmc.2018.02.026
Thiagarajan, P., Kumar, N. H. S., Pazilah, I., Amirin, S., and Suriyati, M. (2013). Anti-tuberculosis activity of lipophilic isoniazid derivatives and interactions with first-line anti-tuberculosis drugs. J. Phar. Res. 7, 313–317. doi: 10.1016/j.jopr.2013.04.039
Trott, O., and Olson, A. J. (2010). AutoDock vina: improving the speed and accuracy of docking with a new scoring function, efficient optimization, and multithreading. J. Comput. Chem. 31, 455–461. doi: 10.1002/jcc.21334
van den Ent, F., Amos, L., and Löwe, J. (2001). Bacterial ancestry of actin and tubulin. Curr. Opin. Microbiol. 4, 634–8. doi: 10.1016/s1369-5274(01)00262-4
World Health Organization (WHO) (2019). Global Tuberculosis Report 2019. Available online at: https://apps.who.int/iris/bitstream/handle/10665/329368/9789241565714-eng.pdf?ua=2019 (accessed 06 January, 2019).
Zhang, H. Z., Damu, G. L., Cai, G. X., and Zhou, C. H. (2013). Design, synthesis and antimicrobial evaluation of novel benzimidazole type of Fluconazole analogues and their synergistic effects with chloromycin, norfloxacin and fluconazole. Eur. J. Med. Chem. 64, 329–344. doi: 10.1016/j.ejmech.2013.03.049
Keywords: benzimidazole derivatives, Mycobacterium tuberculosis, mycobacterial intracellular activity, FtsZ protein, docking study
Citation: Jiménez-Juárez R, Cruz-Chávez W, de Jesús-Ramírez N, Castro-Ramírez GI, Uribe-González I, Martínez-Mejía G, Ruiz-Nicolás R, Aguirre-Alvarado C, Castrejón-Jiménez NS and García-Pérez BE (2020) Synthesis and Antimycobacterial Activity of 2,5-Disubstituted and 1,2,5-Trisubstituted Benzimidazoles. Front. Chem. 8:433. doi: 10.3389/fchem.2020.00433
Received: 17 February 2020; Accepted: 27 April 2020;
Published: 19 June 2020.
Edited by:
Tara Louise Pukala, University of Adelaide, AustraliaReviewed by:
Umer Rashid, COMSATS University Islamabad, PakistanCopyright © 2020 Jiménez-Juárez, Cruz-Chávez, de Jesús-Ramírez, Castro-Ramírez, Uribe-González, Martínez-Mejía, Ruiz-Nicolás, Aguirre-Alvarado, Castrejón-Jiménez and García-Pérez. This is an open-access article distributed under the terms of the Creative Commons Attribution License (CC BY). The use, distribution or reproduction in other forums is permitted, provided the original author(s) and the copyright owner(s) are credited and that the original publication in this journal is cited, in accordance with accepted academic practice. No use, distribution or reproduction is permitted which does not comply with these terms.
*Correspondence: Rogelio Jiménez-Juárez, cm9nZWxpb2pqQGdtYWlsLmNvbQ==; Blanca Estela García-Pérez, YWJyaWxlc3RlbGFAaG90bWFpbC5jb20=; YmxnYXJjaWFwQGlwbi5teA==
Disclaimer: All claims expressed in this article are solely those of the authors and do not necessarily represent those of their affiliated organizations, or those of the publisher, the editors and the reviewers. Any product that may be evaluated in this article or claim that may be made by its manufacturer is not guaranteed or endorsed by the publisher.
Research integrity at Frontiers
Learn more about the work of our research integrity team to safeguard the quality of each article we publish.