- 1Institute of Biotechnology, University of Helsinki, Helsinki, Finland
- 2Department of Agricultural Sciences, University of Helsinki, Helsinki, Finland
- 3National Center for Biotechnology, Nur-Sultan, Kazakhstan
Cytochrome c oxidase is terminal enzyme in the respiratory chain of mitochondria and many aerobic bacteria. It catalyzes reduction of oxygen to water. During its catalysis, CcO proceeds through several quite stable intermediates (R, A, PR/M, O/OH, E/EH). This work is concentrated on the elucidation of the differences between structures of oxidized intermediates O and OH in different CcO variants and at different pH values. Oxidized intermediates of wild type and mutated CcO from Paracoccus denitrificans were studied by means of static and time-resolved Fourier-transform infrared spectroscopy in acidic and alkaline conditions in the infrared region 1800–1000 cm−1. No reasonable differences were found between all variants in these conditions, and in this spectral region. This finding means that the binuclear center of oxygen reduction keeps a very similar structure and holds the same ligands in the studied conditions. The further investigation in search of differences should be performed in the 4000–2000 cm−1 IR region where water ligands absorb.
Introduction
Cytochrome c oxidase is the terminal complex of the respiratory chain of mitochondria, and some aerobic bacteria and archaea. CcO possesses four redox centers, in the direction of electron flow: CuA, heme a, heme a3, and CuB. The last two centers form the binuclear center (BNC) where catalysis takes place. CcO receives electrons from a small soluble enzyme Cytochrome c from the positively charged side of the membrane (P-side). Cytochrome c passes electrons to CuA one by one. For oxygen reduction catalysis, the BNC of CcO must receive four protons (“chemical” protons; because they go for the chemistry of the catalytic cycle) from negatively charged side of membrane (N-side). This reaction is very exergonic, and the energy released is used to translocate four more protons across the membrane (these are called “pumped” protons) (Wikstrom, 1977, 2004; Babcock and Wikstrom, 1992; Brzezinski, 2004). Two H-conductive channels serve to transfer the protons needed for the chemistry and pumping: D- and K- channels, named after the conserved residues D-124 and K-354 (here Paracoccus denitrificans numbering used because this work was performed on P. denitrificans CcO enzyme), respectively (Hosler et al., 1993; Thomas et al., 1993; Fetter et al., 1995; Iwata et al., 1995; Tsukihara et al., 1996; Ostermeier et al., 1997; Yoshikawa et al., 1998). The catalytic cycle includes six relatively stable at room temperature intermediates determined mainly by time-resolved visible and Raman spectroscopies: R (fully-reduced) → A (ferrous-oxy) → PR/M (“peroxy”; PR formed from fully reduced and PM formed from two electron reduced enzyme, electrons are located at the BNC) → F (ferryl) → O/OH (fully-oxidized “resting”/”pulsed”) → E/EH (one-electron reduced “resting”/”pulsed” states). [Figure 1, reproduced from Gobrikova, 2009]. The catalytic cycle can be speculatively divided into two halves: oxidative (R → O) and reductive (O → R) halves (Figure 1). This work focuses on the fully-oxidized intermediate that is measured from both sides of the catalytic circle in experiments. In this intermediate all four redox centers are present in a fully-oxidized state. The fully-oxidized intermediate may exist in different forms with different rates of reduction and reactivity toward external ligands (Moody et al., 1991; John Moody, 1996). The “slow” form has a maximum in the Soret region below 418 nm and is characterized by the slow kinetic of cyanide binding to the oxidized heme a3. The absorption maximum of the “fast” form red-shifted by several nm. The enzyme in the “slow” form can be obtained when the protein is incubated or isolated at a low pH value. When CcO is extracted from the membrane, it exists in the slow or “resting” O form. Moreover, this form is heterogenic and is thought of as simply an artifact of preparation method. Incubation of “fast” oxidase with chloride or bromide at low pH produces forms of the enzyme that are similar to the “slow” form of the enzyme (Moody et al., 1991). Reduction of the enzyme in O state does not couple to proton pumping (Verkhovsky et al., 1999, 2001; Ruitenberg et al., 2000). “Slow” oxidase shows characteristic EPR signal arising from the BNC, at g ≈ 12 and g = 2.95, that are not seen in the fast form of enzyme. Reduction of this enzyme and its re-oxidation produces a fast or “pulsed” OH state. The reduction of this intermediate is accompanied by the pumping of two protons across the membrane (Verkhovsky et al., 1999; Bloch et al., 2004). It was predicted that OH state relaxes to the O state within about ~30 s at room temperature (Bloch et al., 2004). It was thought that the “resting” and “pulsed” states differ in their redox properties or ligands of the BNC (Bloch et al., 2004). Later it was proposed that the difference between these intermediates are in the presence of water molecules or their reorientation in the BNC or in close proximity of it (Jancura et al., 2006; Gorbikova et al., 2008). Later, Jancura et al. (2006) characterized O and OH protein states by visible kinetic and EPR spectroscopies and found no difference between these two states. They concluded that the reduction potentials and the ligation states of heme a3and CuB are the same for CcO in the O and OH states. Nowadays, the structure of the BNC in O and OH states are supposed to be as follows: FeIII-OH- CuII-HOH Tyr(280)-O- (as in Figure 1). This paper presents a study of O→R transition on statically-resolved FTIR redox spectra of wild type (WT) enzyme at pH 6.0 and 9.0, and R→O transition of WT at two pH values (6.0 and 9.0); on D124N variant at pH 9.0; and N131V mutant at pH 6.5 and 9.0. R intermediate is assumed to be the same in all studied cases and only oxidized intermediates may vary. Here, static and time-resolved FTIR spectroscopy demonstrates that there are no clear differences in the oxidized intermediate irrespective of type of the mutant enzyme or WT and the way the O/OH intermediate is produced and measured, i.e., there is no difference between “resting” and “pulsed” fully-oxidized intermediate within the measured IR spectral range.
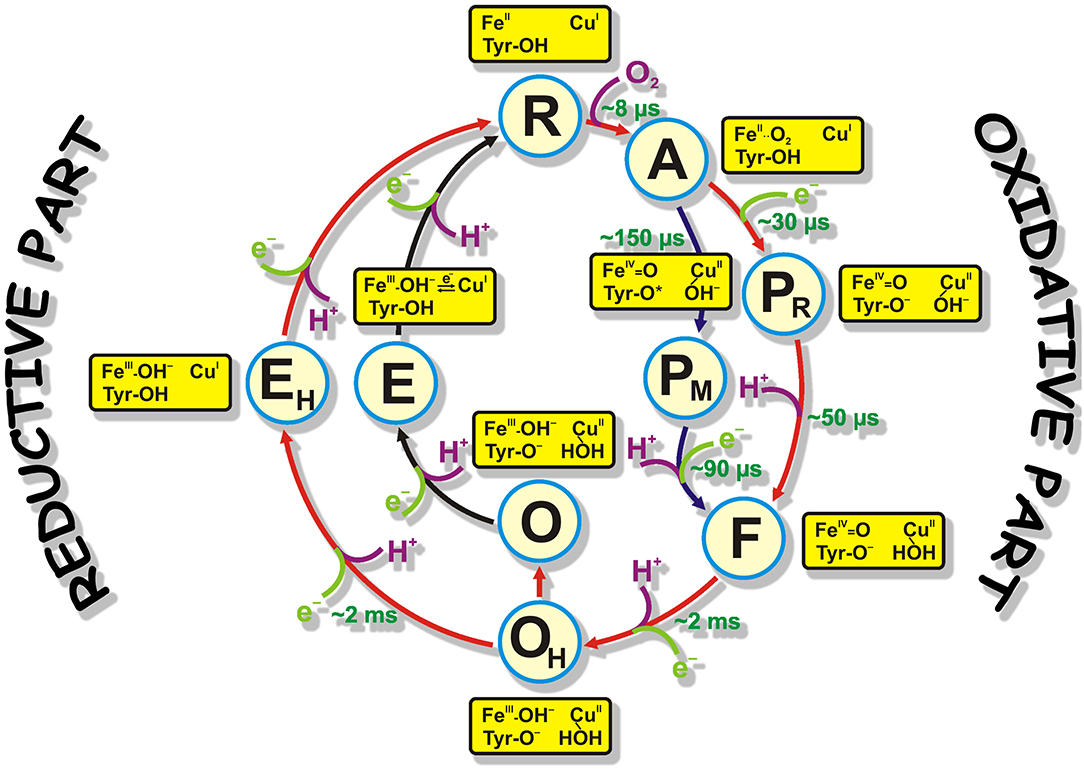
Figure 1. The catalytic cycle of CcO with structures of resolved intermediates. Protons taken by the BNC for the reaction chemistry are shown in magenta. Electrons taken by the BNC are marked in light green. Time constants of the appearance of reaction intermediates are also shown in green. The proposed structures of the catalytic site represent only the BNC structure and provide no information about the other two redox centers (CuA and heme a).
Materials and Methods
Sample Preparation
For the preparation of “ATR-ready” sample, the variants of the enzyme were depleted of the detergent as originally described in Rich and Breton (2002) with some modifications (Ribacka et al., 2005; Gorbikova et al., 2006, 2007b). The “ATR-ready” sample of CcO was placed on a microprism slightly polished by aluminum powder ATR (Attenuated total reflectance) (Sens IR Technologies, three-bounce version, surface diameter 3 mm). The sample was dried under soft N2 flow or under a tungsten fiber lamp positioned at a distance of ~30 cm from the sample film, then, rewetted with “working” buffer (= buffer in which the experiments are undertaken). In all experiments, the enzyme film was stabilized overnight, then covered with a custom designed chamber either for equilibrium FTIR measurements (Gorbikova et al., 2006) or for a time-resolved approach (Gorbikova et al., 2007b) (also see two sections below). Sample preparation for the static experiment included pumping of “working buffer” overnight in order to stabilize the protein film. In the morning, such a sample was ready for static experiments. Sample preparation for the kinetic FTIR RO measurements also included overnight incubation but without pumping and FRCO (Fully-reduced CO-inhibited) complex preparation with buffer contained 100% CO gas. Formation of FRCO complex was followed by the appearance of heme a3-C≡O band at 1,965 cm−1 and took ~40–80 min.
Equilibrium O → R FTIR Spectra
Equilibrium redox titration setup built in Helsinki Bioenergetics Group (Gorbikova et al., 2006; Gobrikova, 2009; Figures 9, 10 therein) allows to measure the reductive half of the catalytic cycle (Figure 1). Equilibrium redox FTIR spectra have been measured in a broad pH region before (Iwaki et al., 2004; Gorbikova et al., 2007a). The buffer composition was the following: 400 mM of K2SO4, 25 mM of potassium acetate, 25 mM of potassium phosphate, and 25 mM of boric acid. The spectra were produced electrochemically with a homemade flow-by electrochemical cell (Vuorilehto et al., 2004). The picture of the cell with its description can be found in Gorbikova et al. (2006) and Vuorilehto et al. (2004). In short, gold grains were used as the working electrode, a platinum-plated titanium grid as the counter electrode and Ag/AgCl/saturated KCl as the reference electrode. The “working buffer” with mediators was continuously pumped through the working electrode to the ATR chamber. The cell was connected to a Princeton Applied Research potentiostat (Oak Ridge, Tennessee, USA). To equilibrate the enzyme film with the potential of the working electrode, two mediators were used: 1,2-diaminocyclohexane-N,N,N‘,N‘-tetraacetic acid + Fe, midpoint potential +95 mV; and ferrocene acetate, +370 mV. The kinetics of equilibration of the enzyme film with the potential of the working electrode was controlled in the visible region spectrophotometrically. The O → R spectra measurements were performed as follows. First, the enzyme film was equilibrated at acidic pH at −300 mV (vs NHE, Normal Hydrogen Electrode) for 15 min. Then, a background (BG) spectrum in the infrared (IR) region was measured. Then, the potential was set at +480 mV, again allowing 15 min for equilibration. At this point, a spectrum in the IR region was taken. Immediately after that, BG in the IR region was collected. The potential was then switched back to −300 mV and the procedure was repeated many times to collect FTIR spectra with good signal-to-noise ratio. Then, the pH of the “working buffer” was changed to another value (in this case to pH 9.0) and a series of R → O and O → R spectra were measured and summed up. To follow oxidoreduction, an FTIR spectrometer Bruker IFS 66/s (Ettlingen, Germany) equipped with a fast MCT detector and a visible spectrophotometer USB2000 (Ocean Optics) (Largo, USA) were used. FTIR spectra were measured in the spectral range 4000–5000 cm−1 with 4 cm−1 spectral resolution and IR scanner frequency 80 kHz. The number of scans in BG and sample spectra was 2000; apodization function, Blackman-Harris 3-Term. The control of the status of oxidoreduction was achieved by visible spectroscopy at 445 nm. The light guide from a visible spectrophotometer was connected to the ATR-chamber. The data acquisition system described above produced one R → O and one O → R spectrum that were summed up. All measurements were performed at room temperature. For more detailed description of the procedure of spectra collection see (Gorbikova et al., 2007a). The whole data acquisition process initializing, sending commands to/from Ocean Optics and FTIR Opus software, and collecting data was controlled by Titrator software designed in the Helsinki Bioenergetics Group by Dr. Nikolay Belevich.
Time-Resolved R → O FTIR Spectra
With help of time-resolved R → O FTIR spectra, the oxidative part of the catalytic cycle of CcO was investigated. Time-resolved R → O FTIR spectra were measured in the following conditions: WT at pH 6.0 and 9.0; N131V at pH 6.5 and 9.0; and D124N at pH 9.0. These spectra were measured in order to distinguish similarities and dissimilarities between O and OH states in different conditions as much as possible. To perform time-resolved measurements of the R → O transition, a special setup was constructed. The core of this setup is an FTIR spectrometer Bruker IFS 66/s equipped with a fast MCT detector and an ATR-cell. The ATR-cell is covered with a special chamber for time-resolved oxygen reaction measurements. Enzyme on ATR-prism is prepared in FRCO form. CO is photolyzed from CcO FRCO enzyme by a laser that is delivered by a laser guide connected to the chamber. Oxygenated buffer is injected very close to the enzyme film. A light guide from a visible spectrophotometer is constructed in the ATR-chamber as well as an inlet and outlet of the pump. For more details and for pictures see [Gorbikova et al., 2007b and Figure 1 therein and Gobrikova, 2009, Figures 12–14 therein]. The “working buffer” for time-resolved FTIR measurements included 100 mM glucose [Merck (Darmstadt, Germany)], 260 μg/mL catalase [Sigma-Aldrich (St. Louis, Missouri, United States)], 3.3 mM ascorbate [Prolabo (Leicestershire, UK)], 10–100 μM hexaaminruthenium (III) chloride [Sigma-Aldrich (St. Louis, Missouri, United States)] (the amount of the last component depended on CcO variant), and 670 μg/mL glucose oxidase [Roche (Basel, Switzerland)]. Before each experiment, the sample quality control was conducted (Gorbikova et al., 2007b). Four procedures were applied in order to test the stability and functionality of the BNC of CcO. First, the intensity and the shape of the band at 1965 cm−1 was analyzed. Second, CO dissociation from heme a3was followed by visible time-resolved spectroscopy with 1 ms temporal resolution. Third, CO dissociation in the dark from heme a3was followed by time-resolved (TR) rapid-scan FTIR in the region around the band at 1965 cm−1. Finally, CO dissociation from FRCO compound at 1965 cm−1 was measured after oxygenated buffer addition, delay of 3 s, and laser flash. This last test was performed before, in the middle, and at the end of each experiment. It showed the amount of enzyme capable of performing the oxygen reaction. FRCO → O spectra were acquired as initially described in (Gorbikova et al., 2007b). Spectra were measured in 1850-950 cm−1 region, which was cut out by an interference filter. The spectra were collected in the rapid-scan mode with ~46 ms temporal resolution and 8 cm−1 spectral resolution. Together with oxygen injection, rapid-scan acquisition started and was followed by a 3 s delay and the laser flash. The flow pump was stopped during the kinetic data collection and switched on immediately after to speed up the CcO re-reduction. This cycle was repeated many times to get spectra of a good signal-to-noise-ratio. A global fitting procedure in Matlab software (Natick, MA, USA) was applied to extract the slow part of kinetical spectra. The fast part of kinetical spectra was calculated as a difference between the spectrum obtained by the average of several time points before the laser flash and the spectrum obtained immediately after it. The sum of the fast and the slow components gave resulting kinetic spectrum in case of mutated CcO. The kinetic spectrum in case of WT enzyme was calculated as a spectral jump after the laser flash because oxygen reaction in WT enzyme proceeds faster than temporal resolution of the measurement setup. FRCO photolysis was measured separately at pH 6.5 on WT variant and subtracted from each FRCO → O spectra to produce R → O spectra presented in the figures in the section “Results”. It was shown in (Heitbrink et al., 2002) that FRCO photolysis on WT does not depend on pH values. All measurements of kinetic R → O transitions and FRCO photolysis were performed in ice- cooled conditions in order to slow-down the reaction rate and increase concentration of oxygen until reaching 2.4 mM. For more details see (Gorbikova et al., 2007b, 2008).
Data Analysis
All data analysis and figure preparations were performed in Matlab software.
Results and Discussions
Here, the IR spectra are compared in acidic conditions on static and time-resolved redox transitions (O → R for static transitions and R → O for the time-resolved). Altogether 6000 FTIR O → R static spectra were collected at pH 6.0 on WT (Figure 2, red spectrum), 173 kinetic spectra of WT at pH 6.0 (Figure 2, dark green spectrum), and 338 kinetic spectra of N131V at pH 6.5 (Figure 2, dark blue spectrum). Small differences between static and kinetic spectra are due to difference in their spectral resolution which is 4 cm−1 for static and 8 cm−1 for the kinetics spectra. Similar results were observed under alkaline conditions: 296 kinetic spectra for D124N at pH 9.0 (Figure 3, dark blue), 218 for N131V 9.0 (Figure 3, dark green), 82 for WT (Figure 3, red), and 6000 co-additions for WT in static conditions (Figure 3, light-green). No differences were found between oxidized intermediates in the IR region 1800–1000 cm−1 for all studied cases in both acidic and alkaline conditions. There are no visible differences between static and time-resolved R → O FTIR spectra of WT and mutated enzymes in the IR region 1800–1000 cm−1 that prove that the BNC of these variants and in these pH conditions are very similar. It does not matter what side we go R → O or O → R, oxidized intermediate provides the same findings and no difference between O and OH is observed in the 1800–1000 cm−1 infrared window for all conditions studied. Further, the difference should be sought in the so-called “water region.”
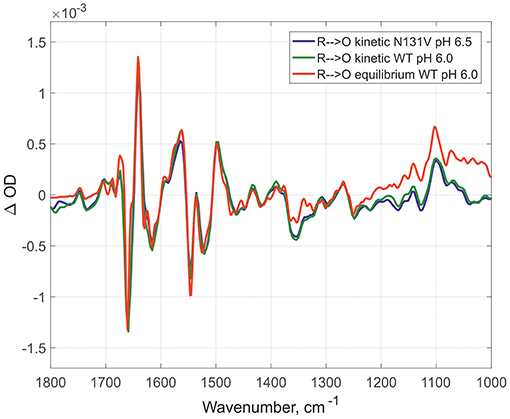
Figure 2. Comparison of the R → O transitions in three different conditions. Time-resolved mode for N131V at pH 6.5 in dark blue and for WT at pH 6.0 in dark green; equilibrium mode for WT at pH 6.0 in red. All spectra normalized to 1 mM (kinetic—by amplitude of 1,965 cm-1 band, equilibrium—by 1661/1641 peaks difference).
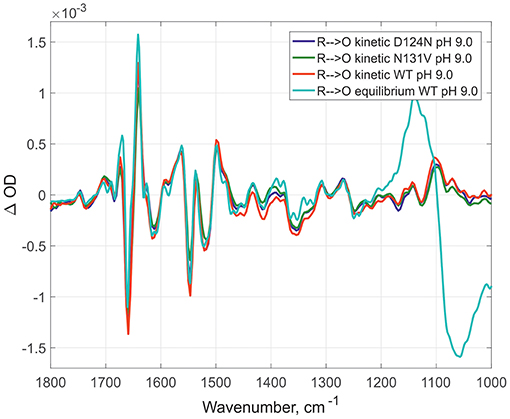
Figure 3. Comparison of R → O transitions in four different conditions at pH 9.0. Time- resolved spectra for D124N (in dark blue), N131V (in dark green), and WT (in red); equilibrium spectrum for WT (light green). All spectra were measured at pH 9.0 and normalized to 1 mM (kinetic—by amplitude of 1,965 cm-1 band, equilibrium—by 1661/1641 peaks difference).
Conclusions
This paper compared the oxidized intermediates derived from different variants (WT, D124N, and N131V) and using different approaches (static O → R spectra acquisition and time-resolved R → O spectra). To perform this work, two special setups were constructed: one for static measurements and one for time-resolved measurements. This work provides an overview of the oxidized intermediates prepared under very different conditions: different mutants and pH values. No differences are evident between all of the conditions tested, except that for static spectra spectral resolution was set at 4 cm−1 and for time-resolved at 8 cm−1. This similarity in oxidized intermediates under different conditions means that the structures of BNC in all these cases (O and OH states) are very similar from the FTIR fingerprint point of view. Further investigation in the IR region 4000–2000 cm−1 is required to search for differences between various oxidized intermediates.
Data Availability Statement
The raw data supporting the conclusions of this article will be made available by the authors, without undue reservation, to any qualified researcher.
Author's Note
This manuscript has been released as a pre-print at bioRxiv (Gorbikova and Kalendar, 2018).
Author Contributions
EG designed research, performed research, analyzed the data, and wrote the paper. RK analyzed the data, wrote the paper.
Funding
EG was supported by Center of International mobility (CIMO) and Informational and Structural Biology (ISB) Graduate School. The work was supported by the Sigrid Juselius foundation, Biocentrum Helsinki and the Academy of Finland (project numbers 200726, 44895, and 115108).
Conflict of Interest
The authors declare that the research was conducted in the absence of any commercial or financial relationships that could be construed as a potential conflict of interest.
Acknowledgments
We warmly thank Dr. Nikolai Belevich for an excellent technical assistance, Dr. Timur Nikitin for critical reading of the manuscript and Dr. Jennifer Rowland (University of Helsinki) for outstanding editing and proofreading of the manuscript.
Abbreviations
A, ferrous-oxy intermediate; ATR, Attenuated total reflectance; BG, background; BNC, binuclear center; CcO, Cytochrome c oxidase; E, one-electron reduced intermediate; F, ferryl intermediate; FRCO, fully-reduced CO-inhibited enzyme; FTIR, Fourier transform infrared; IR, infrared; NHE, Normal Hydrogen Electrode; N-side, negatively charged side of the membrane; PR/M, “peroxy” intermediates; P-side, positively charged side of the membrane; R, fully-reduced intermediate; TR, time-resolved; WT, wild type.
References
Babcock, G. T., and Wikstrom, M. (1992). Oxygen activation and the conservation of energy in cell respiration. Nature 356, 301–309. doi: 10.1038/356301a0
Bloch, D., Belevich, I., Jasaitis, A., Ribacka, C., Puustinen, A., Verkhovsky, M. I., et al. (2004). The catalytic cycle of cytochrome c oxidase is not the sum of its two halves. Proc. Natl. Acad. Sci. U.S.A. 101, 529–533. doi: 10.1073/pnas.0306036101
Brzezinski, P. (2004). Redox-driven membrane-bound proton pumps. Trends Biochem. Sci. 29, 380–387. doi: 10.1016/j.tibs.2004.05.008
Fetter, J. R., Qian, J., Shapleigh, J., Thomas, J. W., Garcia-Horsman, A., Schmidt, E., et al. (1995). Possible proton relay pathways in cytochrome c oxidase. Proc. Natl. Acad. Sci. U.S.A. 92, 1604–1608. doi: 10.1073/pnas.92.5.1604
Gobrikova, E. (2009). Oxygen Reduction and Proton Translocation by Cytochrome C Oxidase (Ph.D. thesis). University of Helsinki, Department of Biological and Environmental Sciences & Institute of Biotechnology & National Graduate School in Informational and Structural Biology Åbo Akademi, Helsinki, Finland.
Gorbikova, E., and Kalendar, R. (2018). Comparison between O and OH intermediates of cytochrome c oxidase studied by FTIR spectroscopy. bioRxiv. 275099. doi: 10.1101/275099
Gorbikova, E. A., Belevich, N. P., Wikstrom, M., and Verkhovsky, M. I. (2007a). Protolytic reactions on reduction of cytochrome c oxidase studied by ATR-FTIR spectroscopy. Biochemistry 46, 4177–4183. doi: 10.1021/bi602523a
Gorbikova, E. A., Belevich, N. P., Wikstrom, M., and Verkhovsky, M. I. (2007b). Time-resolved ATR-FTIR spectroscopy of the oxygen reaction in the D124N mutant of cytochrome c oxidase from Paracoccus denitrificans. Biochemistry 46, 13141–13148. doi: 10.1021/bi701614w
Gorbikova, E. A., Vuorilehto, K., Wikstrom, M., and Verkhovsky, M. I. (2006). Redox titration of all electron carriers of cytochrome c oxidase by Fourier transform infrared spectroscopy. Biochemistry 45, 5641–5649. doi: 10.1021/bi060257v
Gorbikova, E. A., Wikstrom, M., and Verkhovsky, M. I. (2008). The protonation state of the cross-linked tyrosine during the catalytic cycle of cytochrome c oxidase. J. Biol. Chem. 283, 34907–34912. doi: 10.1074/jbc.M803511200
Heitbrink, D., Sigurdson, H., Bolwien, C., Brzezinski, P., and Heberle, J. (2002). Transient binding of CO to CuB in cytochrome c oxidase is dynamically linked to structural changes around a carboxyl group: a time-resolved step-scan fourier transform infrared investigation. Biophys. J. 82, 1–10. doi: 10.1016/S0006-3495(02)75368-X
Hosler, J. P., Ferguson-Miller, S., Calhoun, M. W., Thomas, J. W., Hill, J., Lemieux, L., et al. (1993). Insight into the active-site structure and function of cytochrome oxidase by analysis of site-directed mutants of bacterial cytochrome aa3 and cytochrome bo. J. Bioenerg. Biomembr. 25, 121–136. doi: 10.1007/BF00762854
Iwaki, M., Puustinen, A., Wikstrom, M., and Rich, P. R. (2004). ATR-FTIR spectroscopy and isotope labeling of the PM intermediate of Paracoccus denitrificans cytochrome c oxidase. Biochemistry. 43, 14370–14378. doi: 10.1021/bi048545j
Iwata, S., Ostermeier, C., Ludwig, B., and Michel, H. (1995). Structure at 2.8 a resolution of cytochrome c oxidase from Paracoccus denitrificans. Nature 376, 660–669. doi: 10.1038/376660a0
Jancura, D., Berka, V., Antalik, M., Bagelova, J., Gennis, R. B., Palmer, G., et al. (2006). Spectral and kinetic equivalence of oxidized cytochrome C oxidase as isolated and “activated” by reoxidation. J. Biol. Chem. 281, 30319–30325. doi: 10.1074/jbc.M605955200
John Moody, A. (1996). ‘As prepared' forms of fully oxidised haem/Cu terminal oxidases. Biochim. Biophys. Acta. 1276, 6–20. doi: 10.1016/0005-2728(96)00035-7
Moody, A. J., Cooper, C. E., and Rich, P. R. (1991). Characterisation of ‘fast' and ‘slow' forms of bovine heart cytochrome-c oxidase. Biochim. Biophys. Acta. 1059, 189–207. doi: 10.1016/S0005-2728(05)80204-X
Ostermeier, C., Harrenga, A., Ermler, U., and Michel, H. (1997). Structure at 2.7 A resolution of the Paracoccus denitrificans two-subunit cytochrome c oxidase complexed with an antibody FV fragment. Proc. Natl. Acad. Sci. U.S.A. 94, 10547–10553. doi: 10.1073/pnas.94.20.10547
Ribacka, C., Verkhovsky, M. I., Belevich, I., Bloch, D. A., Puustinen, A., and Wikstrom, M. (2005). An elementary reaction step of the proton pump is revealed by mutation of tryptophan-164 to phenylalanine in cytochrome c oxidase from Paracoccus denitrificans. Biochemistry 44, 16502–16512. doi: 10.1021/bi0511336
Rich, P. R., and Breton, J. (2002). Attenuated total reflection Fourier transform infrared studies of redox changes in bovine cytochrome c oxidase: resolution of the redox Fourier transform infrared difference spectrum of heme a(3). Biochemistry. 41, 967–973. doi: 10.1021/bi0109717
Ruitenberg, M., Kannt, A., Bamberg, E., Ludwig, B., Michel, H., and Fendler, K. (2000). Single-electron reduction of the oxidized state is coupled to proton uptake via the K pathway in Paracoccus denitrificans cytochrome c oxidase. Proc. Natl. Acad. Sci. U.S.A. 97, 4632–4636. doi: 10.1073/pnas.080079097
Thomas, J. W., Puustinen, A., Alben, J. O., Gennis, R. B., and Wikstrom, M. (1993). Substitution of asparagine for aspartate-135 in subunit I of the cytochrome bo ubiquinol oxidase of Escherichia coli eliminates proton-pumping activity. Biochemistry 32, 10923–10928. doi: 10.1021/bi00091a048
Tsukihara, T., Aoyama, H., Yamashita, E., Tomizaki, T., Yamaguchi, H., Shinzawa-Itoh, K., et al. (1996). The whole structure of the 13-subunit oxidized cytochrome c oxidase at 2.8 A. Science 272, 1136–1144. doi: 10.1126/science.272.5265.1136
Verkhovsky, M. I., Jasaitis, A., Verkhovskaya, M. L., Morgan, J. E., and Wikstrom, M. (1999). Proton translocation by cytochrome c oxidase. Nature 400, 480–483. doi: 10.1038/22813
Verkhovsky, M. I., Tuukkanen, A., Backgren, C., Puustinen, A., and Wikstrom, M. (2001). Charge translocation coupled to electron injection into oxidized cytochrome c oxidase from Paracoccus denitrificans. Biochemistry 40, 7077–7083. doi: 10.1021/bi010030u
Vuorilehto, K., Lutz, S., and Wandrey, C. (2004). Indirect electrochemical reduction of nicotinamide coenzymes. Bioelectrochemistry 65, 1–7. doi: 10.1016/j.bioelechem.2004.05.006
Wikstrom, M. (2004). Cytochrome c oxidase: 25 years of the elusive proton pump. Biochim Biophys Acta 1655, 241–247. doi: 10.1016/j.bbabio.2003.07.013
Wikstrom, M. K. (1977). Proton pump coupled to cytochrome c oxidase in mitochondria. Nature 266, 271–273. doi: 10.1038/266271a0
Keywords: cell respiration, cytochrome c oxidase, oxidized state, FTIR spectroscopy, oxidoreduction
Citation: Gorbikova E and Kalendar R (2020) Comparison Between O and OH Intermediates of Cytochrome c Oxidase Studied by FTIR Spectroscopy. Front. Chem. 8:387. doi: 10.3389/fchem.2020.00387
Received: 25 January 2020; Accepted: 14 April 2020;
Published: 05 May 2020.
Edited by:
Maria Luisa Mangoni, Sapienza University of Rome, ItalyReviewed by:
Sven Dennerlein, University Medical Center Göttingen, GermanyZufeng Guo, Johns Hopkins University, United States
Copyright © 2020 Gorbikova and Kalendar. This is an open-access article distributed under the terms of the Creative Commons Attribution License (CC BY). The use, distribution or reproduction in other forums is permitted, provided the original author(s) and the copyright owner(s) are credited and that the original publication in this journal is cited, in accordance with accepted academic practice. No use, distribution or reproduction is permitted which does not comply with these terms.
*Correspondence: Ruslan Kalendar, cnVzbGFuLmthbGVuZGFyQGhlbHNpbmtpLmZp
†ORCID: Elena Gorbikova orcid.org/0000-0002-6078-6012 Ruslan Kalendar orcid.org/0000-0002-3986-2460