- Future Energy Laboratory, School of Materials Science and Engineering, Hefei University of Technology, Hefei, China
Zn–air battery is considered as one of the most promising candidates for next-generation batteries for energy storage due to safety, high energy density, and low cost. There are many challenges in electrolytes for developing high-performance rechargeable Zn–air cells as well as electrocatalysts. An electrolyte is the crucial part of the rechargeable Zn–air batteries that determine their capacity, cycling stability, and lifetime. This paper reviews the most recent progress in designing and fabricating electrolytes in aqueous and flexible Zn–air batteries. The discussion on the surface reaction relationships was covered between air–catalyst–electrolyte and electrolyte–zinc reaction mechanism. We highlight the recent developments of three different electrolytes in zinc–air battery: aqueous electrolyte, room temperature ionic liquid, and quasi-solid flexible electrolyte. Furthermore, the general perspective is proposed for designing and fabricating electrolytes to improve the performance and prolong the lifetime of Zn–air batteries.
Introduction
Zn–air battery has high specific energy (1,218 Wh·kg−1). Meanwhile, its inherent features, including safety and lower cost, make it one of the most promising next-generation batteries (Fu et al., 2017; Tan et al., 2017; Han et al., 2019). The role of electrolytes has been overlooked compared to the hot research on bifunctional air electrodes for Zn–air batteries. The performance of electrolytes directly determines the ionic conductivity and interfacial properties of the Zn–air battery in operation. Furthermore, it further affects the capacity, cycling stability, and charging and discharging efficiency of the cell (Pei et al., 2014). Zn–air batteries are developing toward high efficiency and durability, which cannot separate from the support of electrolyte with excellent performance in all aspects (R. Mainar et al., 2016). Thus, it is urgently significant to delve into the workings of electrolytes in Zn–air batteries (Mainar et al., 2018).
At present, the alkaline electrolyte is still widely used in zinc-based batteries to meet the requirements of low cost and high ionic conductivity and ensure the stability of the zinc electrode (R. Mainar et al., 2016; Xu et al., 2020). However, it is susceptible to environmental CO2 and relative humidity. Zn–air battery is mainly dependent on the performance of the air electrode. Unfortunately, CO2 can lead to the generation of K2CO3 in the electrolyte, which adversely affects the void in the air electrode (Wang et al., 2014; Fu et al., 2017). Zn–air batteries must solve the problem of evaporation of electrolyte or absorption of water from the external environment to work well in the complex external environment. The former makes the battery expand, and the latter affects the transfer of OH− (Chakkaravarthy et al., 1981; Mainar et al., 2018). Room temperature ionic liquids (RTILs), and solid electrolytes are alternative and effective solutions to solve the above problem. However, their performance was limited by their low ionic conductivity and unqualified interface. Therefore, in the following parts, we will discuss the research status of alkaline electrolytes and non-aqueous electrolytes in Zn–air batteries.
Aqueous Electrolyte
LiOH, NaOH, and KOH are the common electrolytes for Zn–air batteries. Compared with neutral and acidic electrolytes, the alkaline electrolytes are well-matched with zinc electrodes and catalyst materials. Meanwhile, there is high ionic conductivity and low viscosity in KOH electrolyte. When Zn–air battery discharges, the external oxygen enters the battery and reacts (Equation 1) (oxygen reduction reaction) at the gas–liquid–solid interface (oxygen, electrolyte, electrocatalyst). The zinc electrode transports electrons to the air electrode through the external load, and the OH− at the reaction site generates (Equation 2). When the concentration of reaches the maximum, it will further decompose into ZnO (Equation 3). The complete reaction of the zinc electrode is shown in Equation 4. During the charging process, backward reaction (Equation 1) (oxygen evolution reaction) is performed at the zinc–electrolyte interface, and electrical energy is stored, while zinc deposits by backward reaction (Equation 3).
When the concentration of KOH is 6 M, the current exchange density of Zn/Zn2+ reaches 0.21 A cm−2, and the solubility of ZnO increases with the concentration of KOH (See and White, 1997; Dyer et al., 2009). Therefore, we must pay attention to the adverse effect of high concentration KOH electrolyte on the zinc electrode. The high concentration of ZnO produces excess and precipitates after the discharge, which increases the passivation resistance of the zinc electrode. Besides, the oxygen reduction kinetic parameters of zinc were very high, resulting in the dissolution, migration, and redeposition of zinc under various conditions (R. Mainar et al., 2016).
There are two main strategies to solve this problem. One is to change the composition and structure of the zinc electrode, and the other is to find the appropriate electrolyte additives. Reported methods such as making the zinc electrode have a 3-D structure (Parker et al., 2014; Chamoun et al., 2015; Yan et al., 2015) or the efficient additive for the zinc electrode (Fan et al., 2013; Masri and Mohamad, 2013; Huang et al., 2015) have proven to be an effective solution strategy. It is an urgent task to accurately measure the potential and concentration of zinc ions on the surface of the zinc electrode to provide adequate theoretical support for improving the living conditions of the zinc electrode in the alkaline electrolyte. In Table 1, we summarized the recent work on alkaline electrolyte additives. Suitable additives in electrolytes can improve the shape change of the zinc electrode and the performance of the Zn–air battery. If we can reduce the concentration of KOH as far as possible without affecting the ionic conductivity of the electrolyte, we believe that the performance of the Zn–air battery will be further improved. By adding K2CO3 to high-concentration KOH solution and optimize the structure of the battery, Schröder et al. (2015) not only obtained stable electric potential but also improved the actual energy density and long-term stability of the Zn–air battery. Besides, the inhibition of dendrite growth and hydrogen evolution of zinc electrode is also reported in Zn–air battery with the alkaline electrolytes using sodium dodecylbenzene sulfonate (SDBS) (Yang et al., 2004), polyethylene glycol (PEG) (Banik and Akolkar, 2013), tartaric/succinic/citric acid (Lee et al., 2006), and tetra-alkyl ammonium hydroxides (Lan et al., 2007).
Zn–air battery is a semi-open system that requires the rich oxygen from the outer environment to participate in the reaction process. Carbon dioxide (CO2) is difficult to avoid in a moist atmosphere. CO2 from the outer atmosphere enters the battery through the air electrode and reacts with OH− in the electrolyte (Equations 5, 6).
The ionic conductivity of the electrolyte becomes weakened due to the generation of and and the low solubility of K2CO3 and KHCO3. When they deposit on the air electrode, the oxygen transfer will be blocked to some extent, resulting in the performance decline of the Zn–air battery. Optimizing the structure of the Zn–air battery and the composition of the gas adsorption layer to allow oxygen to pass through unimpeded but to inhibit the passage of carbon dioxide and water vapor is an ideal solution. To solve the above problems, investigators also put forward several solutions. Pedicini et al. (1996) set up an air manager system for recirculating reactant air in the metal–air battery. Goldstein et al. (1997) put forward a scrubber system for removing carbon dioxide from a metal–air or fuel cell battery. Pedicni (2002) proposed to limit carbon dioxide and water vapor when the battery is not in use by loading a responsive air door for an electrochemical cell. There are many solutions to solve these problems, but the limitations are high-cost thresholds and reduced space utilization, which limit the development of Zn–air batteries in practical applications.
The flow electrolyte system is a very effective method for Zn–air batteries. The electrolyte is pumped and circulated through a power system of external pipes and pumps. In addition to removing the precipitated carbonate and other by-products through external filters, the flowing electrolyte improves OH− transfer and reduces concentration gradients (Iacovangelo and Will, 1985; Cheng et al., 2007). Compared with the static electrolyte, Zn–air battery is much improved including the cycle life and operating voltage with a circulating electrolyte system. However, the power of electrolyte circulation needs to be supported by an external pumping system and electric energy. Therefore, if the electrolyte circulation system is put into practical application, it is necessary to solve the problem that it is difficult to apply to the large-scale grid energy storage system with strict space and weight requirements.
Room Temperature Ionic Liquid
The room temperature ionic liquid is a molten salt that exists as a liquid at or below room temperature. It has a wide electrochemical window and is not easily ignited (Balaish et al., 2014). Therefore, more and more attention was paid to RTILs as substitutes for alkaline electrolytes. The inherent safety and stability of RTILs over a wide range of electrochemical potentials have led to its application in lithium-based batteries (Chou et al., 2008; Xiang et al., 2010). The use of RTILs in Zn-air batteries can effectively solve the problems of zinc electrode damage (Simons et al., 2012), CO2 damage, and electrolyte evaporation (Harting et al., 2012) in the alkaline electrolyte of the water system mentioned above, and make it possible for the battery to work at high temperatures. Moreover, for aprotic RTILs, the absence of protons can effectively avoid the corrosion of the zinc electrode caused by hydrogen evolution. Therefore, RTILs, as an electrolyte for Zn–air batteries, have put on the list in recent years.
RTILs used as an electrolyte for Zn–air cell, zinc oxidize to Zn2+ during discharge, and the reversible electrochemical reaction of zinc in RTILs has proven to be feasible (Xu et al., 2015). What we need to note here is that inappropriate RTILs may form insoluble substances with Zn2+ and make them unable to be reduced effectively. The mechanism of air electrode in RTIL electrolyte was proposed (Kar et al., 2014).
When oxygen reduction occurs in the RTILs electrolyte, oxygen gains electrons and forms a superoxide () (Equation 7). This reaction is considered quasi-reversible (AlNashef et al., 2002). For aprotic RTILs, there is no further electron transfer due to the presence of superoxide. In contrast, for protic RTILs, superoxide is a strong nucleophile that can further react with protons in RTILs to form per-hydroxy radical () (Equation 8). Then, per-hydroxy radical can also react with superoxide to form peroxide () (Equations 9, 10) and finally complete the reduction process (Equation 11).
As for whether hydrogen peroxide can further decompose into H2O, Zeller (2011) points out that it is determined by the electrode used. According to Kar et al. (2014)'s summary of oxygen reduction and oxygen precipitation reactions in RTILs, in the reaction, as mentioned above, paths have proven to be reversible and relatively stable peroxide products. However, there are still some related disproportionation reactions. Hydrogen peroxide requires less activation energy to produce oxygen, which makes it an effective support for oxygen reduction, and oxygen evolution reactions in RTILs.
The development of RTILs in the Zn–air battery still faces enormous challenges. On the one hand, the high cost of RTILs makes it challenging to use on a large scale. On the other hand, the RTILs' dual-electron reaction mechanism reduces the energy density of the battery, coupled with its high viscosity and low conductivity, which means that the Zn–air battery can only operate at a low current. With Li0.87Na0.63K0.50CO3 and NaOH as the electrolyte, Liu et al. (2017) investigated the Zn–air battery able to charge and discharge at 550°C for 100 cycles with Coulombic efficiency of 96.9%. When Ingale et al. (2017) applied diethylmethylammonium trifluoro-methanesulfonate (DEATfO) ionic liquid to the Zn–air battery, they found that although there was no zinc dendrite generation, the weak surface tension of DEATfO resulted in unsatisfactory energy density (Pozo-Gonzalo et al., 2014). Furthermore, Ghazvini et al. (2018) pointed out the positive effect of water addition on ionic interaction when RTIL electrolyte was used in Zn–air batteries. The above work provides a good strategy for improving the performance of the Zn–air battery with RTILs as an electrolyte.
Further, the application of more types of RTILs in Zn–air batteries should be investigated, including the beneficial effects of additives in RTILs. It is also necessary to develop specific bifunctional catalysts to reduce the energy barrier of oxygen reduction reaction and oxygen evolution reaction. Although the RTIL electrolyte needs further study in terms of interface properties, the electrochemical reaction mechanism of oxygen and the migration path of active substances, various pieces of evidence indicate that RTILs are the promising electrolytes for Zn–air batteries.
Quasi-Solid Flexible Electrolyte
With the increasing demand for flexible wearable electronic devices, the research on flexible batteries, especially quasi-solid electrolytes, has put forward higher requirements. Compared with other metal–air batteries, Zn–air batteries with high volume energy density have the characteristics of low cost and high safety. In contrast, zinc as an electrode has more energetic mechanical properties and productivity in flexible batteries. For example, Zn–MnO2 batteries using polymer electrolytes were commercially produced using printing technology (MacKenzie and Ho, 2015). Therefore, it is necessary to carry out scientific research on the structure and performance of a flexible Zn–air battery, and the production of this type of battery and the matching quasi-solid electrolyte needs to be continuously optimized.
The quasi-solid flexible electrolyte is usually prepared from alkaline aqueous solution and polymers such as polyvinyl alcohol (PVA) (Fan et al., 2019), polyacrylic acid (PAA) (Wu et al., 2006; Zhu et al., 2018), gelatin (Park et al., 2015), and related graft copolymer (Yu et al., 2017), which are required to meet the stable configuration, cathode and anode separation, and qualified ionic conductivity. During the preparation process, most of the quasi-solid flexible electrolytes can form a cross-linked network with a large number of hydrophilic functional groups (such as hydroxyl groups), which enables higher water retention and ionic conductivity in quasi-solid flexible electrolytes. In primary Zn–air cell, alkaline gel electrolyte can effectively reduce the leakage and volatilization of electrolyte and has been applied (Hilder et al., 2009). However, for rechargeable flexible Zn–air batteries, on account of the zinc electrode in the quasi-solid flexible electrolyte, they can only carry a small amount of . The process of ZnO reduction to is blocked (Xu et al., 2015). Therefore, it is a big challenge to realize rechargeable Zn–air batteries to work in a large current.
Flexible power density and cycle performance of the Zn–air battery have been highly favored. However, there are several important aspects in the bifunctional catalyst for electrochemical oxygen reactions, the ionic conductivity of the quasi-solid flexible electrolyte, and the performance of the electrolyte–electrode interface. The ionic conductivity of the electrolyte depends mainly on the type of polymer and electrolyte additives. Fan et al. (2019) prepared a porous PVA + SiO2 electrolyte with high ionic conductivity of 57.3 mS cm−1 and excellent cycling performance and power density. Li et al. (2019) fabricated the polymer dielectric TEAOH-PVA, which still had the ionic conductivity of 30 mS cm−1 after 2 weeks, showing excellent service and working life. It is not difficult to find that a single polymer can hardly become a flexible electrolyte of a quasi-solid state with excellent performance. However, a small number of additives can significantly improve the performance of electrolytes, which is also a process of polymer functionalization. This is mainly because the additive optimizes the structure of the crosslinked network of the polymer electrolyte, increases the number of hydrophilic functional groups (such as hydroxyl groups), and further improves water retention ability of the electrolyte, which has a great influence on the ionic conductivity. Moreover, in addition to the ionic conductivity and water retention performance of the quasi-solid flexible electrolyte, the transfer rate of OH− and should also be put to more attention, which has been paid insufficient emphasis at present. Their transfer process also has a profound impact on the energy density and other performance of flexible Zn–air batteries.
There is a challenge to improve the performance of the electrolyte–electrode interface (especially the electrolyte–air electrode interface) in the flexible Zn–air battery. The wettability of the quasi-solid flexible electrolyte was reduced, which makes it much more difficult for the catalyst to perform its function than in the alkaline electrolyte of the water system. When assembling the battery, Xu et al. (2019) pressed the battery for 3 min under 3 MPa through a tablet press to make the laminated structure more complete, and the flexible Zn–air battery could stabilize the circulation for 35 h. More exploration is still needed to improve the electrolyte–electrode interface, the preparation of electrolyte, and the battery packaging method.
The flexible Zn–air battery also puts forward to the higher requirements on the bending, stretching, and compression performance of the zinc electrode, air electrode, and electrolyte in the battery. The flexible Zn–air battery is generally divided into the 1D structure (line type) and 2D structure (sandwich shaped). Ma et al. (2019) prepared a dual network hydrogel electrolyte (polyacrylate hydrogel cross-linked by cellulose chains and N,N-methylene-bisacrylamide anchors) and optimized the structure of the zinc and air electrodes to assemble the Zn–air battery with excellent tensile properties. Pan et al. (2019) constructed a sponge-like squeezable Zn–air battery that performed well after 60% compression strain or 500 cycles of repeated compression tests. Li et al. (2018) prepared a 1D knittable Zn–air battery with a diameter of only 1.03 mm through the path, which had an excellent performance of flexibility and charge and discharge.
Table 2 lists more compared performances to provide more meaningful routes for the development of quasi-solid flexible electrolytes for Zn–air batteries. However, it is difficult to get a competent evaluation due to the different battery structure, catalyst, and electrolyte used in recorded works. Therefore, it is necessary to establish a unified evaluation standard for flexible Zn–air battery to evaluate the performance of the corresponding electrolyte better. Furthermore, the composition of the electrolyte in the flexible Zn–air battery is mostly in the “polymer + KOH solution” mode, which leads to the advantages and disadvantages of the aqueous electrolyte mentioned above acting on the quasi-solid electrolyte. At the same time, the combination of RTILs with the polymer may inject new vitality into the safety and stability of Zn–air batteries, but its practical feasibility needs to be verified in the coming future.
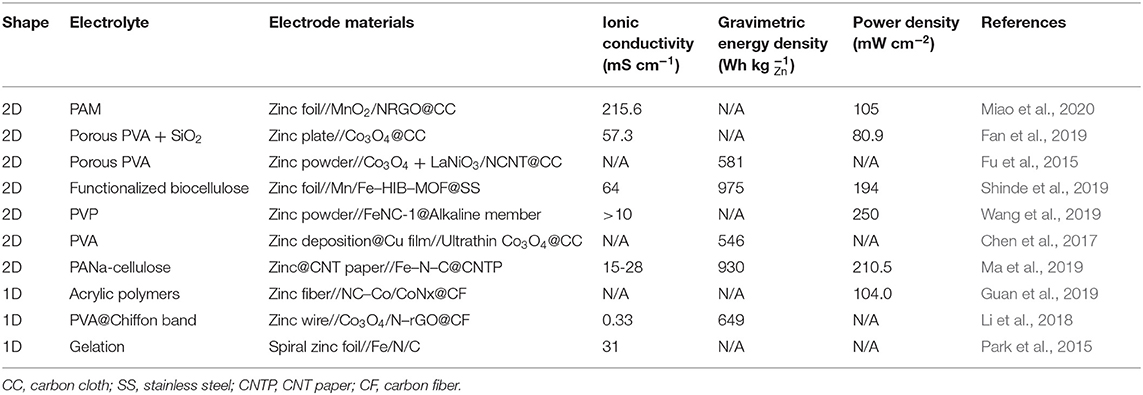
Table 2. Summary of recently reported quasi-solid flexible electrolyte additive for Zn–air batteries.
Summary
With the demand for high-power, long life, and flexibility of rechargeable Zn–air batteries, the development of electrolytes meets the opportunities and challenges. Electrolyte, as a critical part of Zn–air battery, has a profound influence on circulation efficiency, power density, and capacity performance. Up to now, alkaline electrolytes are the mainstream because of their excellent ionic conductivity and interfacial properties. However, alkaline electrolytes are susceptible to the effects of carbon dioxide content and relative humidity in the external environment. On the one hand, the suitable type and proportion of additives should be explored to improve the properties of the alkaline electrolyte. On the other hand, RTILs, as an electrolyte for Zn–air batteries, have a high threshold of aging, and its protection and safety for zinc electrodes are apparent. Moreover, the research on quasi-solid flexible electrolyte is more conducive to making portable and flexible Zn–air batteries, which provide that the shortcomings in interface performance and ionic conductivity need to be addressed. Finding the right RTILs and polymers makes sense to improve the performance of the electrolyte.
Furthermore, we think that the three electrolytes mentioned above may be integrated with different features. Suitable electrolyte additives can also promote the application of RTILs and quasi-solid electrolytes in Zn–air batteries, and the combination of RTILs and polymers can also improve the performance of electrolytes. The research on electrolytes should be paid more attention to make Zn–air batteries meet the demand for a new generation of energy storage.
Author Contributions
All authors listed have made a substantial, direct and intellectual contribution to the work, and approved it for publication.
Funding
This work was financially supported by the Natural Science Foundation of China (U1832136, 21303038), National Students' Innovation and Entrepreneurship Training Program (201910359010), and Natural Science Foundation of Anhui province (1808085QE140).
Conflict of Interest
The authors declare that the research was conducted in the absence of any commercial or financial relationships that could be construed as a potential conflict of interest.
References
AlNashef, I. M., Leonard, M. L., Matthews, M. A., and Weidner, J. W. (2002). Superoxide electrochemistry in an ionic liquid. Ind. Eng. Chem. Res. 41, 4475–4478. doi: 10.1021/ie010787h
Balaish, M., Kraytsberg, A., and Ein-Eli, Y. (2014). A critical review on lithium-air battery electrolytes. Phys. Chem. Chem. Phys. 16, 2801–2822. doi: 10.1039/C3CP54165G
Banik, S. J., and Akolkar, R. (2013). Suppressing dendrite growth during zinc electrodeposition by PEG-200 additive. J. Electrochem. Soc. 160, D519–D523. doi: 10.1149/2.040311jes
Chakkaravarthy, C., Waheed, A. A., and Udupa, H. (1981). Zinc-air alkaline batteries-a review. J. Power Sources 6, 203–228. doi: 10.1016/0378-7753(81)80027-4
Chamoun, M., Hertzberg, B. J., Gupta, T., Davies, D., Bhadra, S., Van Tassell, B., et al. (2015). Hyper-dendritic nanoporous zinc foam anodes. NPG Asia Mater. 7:e178. doi: 10.1038/am.2015.32
Chen, X., Liu, B., Zhong, C., Liu, Z., Liu, J., Ma, L., et al. (2017). Ultrathin Co3O4 layers with large contact area on carbon fibers as high-performance electrode for flexible zinc-air battery integrated with flexible display. Adv. Energy Mater. 7:1700779. doi: 10.1002/aenm.201700779
Cheng, J., Zhang, L., Yang, Y.-S., Wen, Y.-H., Cao, G.-P., and Wang, X.-D. (2007). Preliminary study of single flow zinc-nickel battery. Electrochem. Commun. 9, 2639–2642. doi: 10.1016/j.elecom.2007.08.016
Chou, S.-L., Wang, J.-Z., Sun, J.-Z., Wexler, D., Forsyth, M., Liu, H.-K., et al. (2008). High capacity, safety, and enhanced cyclability of lithium metal battery using a V2O5 nanomaterial cathode and room temperature ionic liquid electrolyte. Chem. Mater. 20, 7044–7051. doi: 10.1021/cm801468q
Dyer, C. K., Moseley, P. T., Ogumi, Z., Rand, D. A., and Scrosati, B. (2009). Encyclopedia Of Electrochemical Power Sources. (Newnes: Elsevier Science & Technology).
Fan, X., Liu, J., Song, Z., Han, X., Deng, Y., Zhong, C., et al. (2019). Porous nanocomposite gel polymer electrolyte with high ionic conductivity and superior electrolyte retention capability for long-cycle-life flexible zinc-air batteries. Nano Energy 56, 454–462. doi: 10.1016/j.nanoen.2018.11.057
Fan, X., Yang, Z., Xie, X., Long, W., Wang, R., and Hou, Z. (2013). The electrochemical behaviors of Zn–Al–La-hydrotalcite in Zn-Ni secondary cells. J. Power Sources 241, 404–409. doi: 10.1016/j.jpowsour.2013.04.136
Fu, J., Cano, Z. P., Park, M. G., Yu, A., Fowler, M., and Chen, Z. (2017). Electrically rechargeable zinc-air batteries: progress, challenges, and perspectives. Adv. Mater. 29:1604685. doi: 10.1002/adma.201604685
Fu, J., Lee, D. U., Hassan, F. M., Yang, L., Bai, Z., Park, M. G., et al. (2015). Flexible high-energy polymer-electrolyte-based rechargeable zinc-air batteries. Adv. Mater. 27, 5617–5622. doi: 10.1002/adma.201502853
Ghazvini, M. S., Pulletikurthi, G., Cui, T., Kuhl, C., and Endres, F. (2018). Electrodeposition of zinc from 1-ethyl-3-methylimidazolium acetate-water mixtures: investigations on the applicability of the electrolyte for Zn-air batteries. J. Electrochem. Soc. 165:D354. doi: 10.1149/2.0181809jes
Goldstein, J. R., Harats, Y., Sharon, Y., and Naimer, N. (1997). Scrubber system for removing carbon dioxide from a metal-air or fuel cell battery. U.S. Patent No. 5,595,949. (Washington, DC: U.S. Patent and Trademark Office patent application).
Guan, C., Sumboja, A., Zang, W., Qian, Y., Zhang, H., Liu, X., et al. (2019). Decorating Co/CoNx nanoparticles in nitrogen-doped carbon nanoarrays for flexible and rechargeable zinc-air batteries. Energy Storage Mater. 16, 243–250. doi: 10.1016/j.ensm.2018.06.001
Han, J., Meng, X., Lu, L., Bian, J., Li, Z., and Sun, C. (2019). Single-atom Fe-Nx-C as an efficient electrocatalyst for Zinc–Air batteries. Adv. Funct. Mater. 29:1808872. doi: 10.1002/adfm.201808872
Harting, K., Kunz, U., and Turek, T. (2012). Zinc-air batteries: prospects and challenges for future improvement. Z. Phys. Chem. 226, 151–166. doi: 10.1524/zpch.2012.0152
Hilder, M., Winther-Jensen, B., and Clark, N. (2009). Paper-based, printed zinc-air battery. J. Power Sources 194, 1135–1141. doi: 10.1016/j.jpowsour.2009.06.054
Hosseini, S., Abbasi, A., Uginet, L.-O., Haustraete, N., Praserthdam, S., Yonezawa, T., et al. (2019). The influence of dimethyl sulfoxide as electrolyte additive on anodic dissolution of alkaline zinc-air flow battery. Sci. Rep. 9:14958. doi: 10.1038/s41598-019-51412-5
Hosseini, S., Han, S. J., Arponwichanop, A., Yonezawa, T., and Kheawhom, S. (2018). Ethanol as an electrolyte additive for alkaline zinc-air flow batteries. Sci. Rep. 8:11273. doi: 10.1038/s41598-018-29630-0
Huang, J., Yang, Z., Wang, R., Zhang, Z., Feng, Z., and Xie, X. (2015). Zn-Al layered double oxides as high-performance anode materials for zinc-based secondary battery. J. Mater. Chem. A 3, 7429–7436. doi: 10.1039/C5TA00279F
Iacovangelo, C. D., and Will, F. G. (1985). Parametric study of zinc deposition on porous carbon in a flowing electrolyte cell. J. Electrochem. Soc. 132:851.
Ingale, P., Sakthivel, M., and Drillet, J. F. (2017). Test of diethylmethylammonium trifluoromethanesulfonate ionic liquid as electrolyte in electrically rechargeable Zn/air battery. J. Electrochem. Soc. 164, H5224–H5229. doi: 10.1149/2.0351708jes
Kar, M., Simons, T. J., Forsyth, M., and MacFarlane, D. R. (2014). Ionic liquid electrolytes as a platform for rechargeable metal-air batteries: a perspective. Phys. Chem. Chem. Phys. 16, 18658–18674. doi: 10.1039/C4CP02533D
Kumar, K. K., Brindha, R., Nandhini, M., Selvam, M., Saminathan, K., and Sakthipandi, K. (2019). Water-suspended graphene as electrolyte additive in zinc-air alkaline battery system. Ionics 25, 1699–1706. doi: 10.1007/s11581-019-02924-7
Lan, C., Lee, C., and Chin, T. (2007). Tetra-alkyl ammonium hydroxides as inhibitors of Zn dendrite in Zn-based secondary batteries. Electrochim. Acta 52, 5407–5416. doi: 10.1016/j.electacta.2007.02.063
Lee, C. W., Sathiyanarayanan, K., Eom, S. W., Kim, H. S., and Yun, M. S. (2006). Novel electrochemical behavior of zinc anodes in zinc/air batteries in the presence of additives. J. Power Sources 159, 1474–1477. doi: 10.1016/j.jpowsour.2005.11.074
Li, M., Liu, B., Fan, X., Liu, X., Liu, J., Ding, J., et al. (2019). Long-shelf-life polymer electrolyte based on tetraethylammonium hydroxide for flexible zinc-air batteries. ACS Appl. Mater. Interfaces 11, 28909–28917. doi: 10.1021/acsami.9b09086
Li, M., Luo, F., Zhang, Q., Yang, Z., and Xu, Z. (2020). Atomic layer Co3O4-x nanosheets as efficient and stable electrocatalyst for rechargeable zinc-air batteries. J. Catal. 381, 395–401. doi: 10.1016/j.jcat.2019.11.020
Li, Y., Zhong, C., Liu, J., Zeng, X., Qu, S., Han, X., et al. (2018). Atomically thin mesoporous Co3O4 layers strongly coupled with N-rGO nanosheets as high-performance bifunctional catalysts for 1D knittable zinc-air batteries. Adv. Mater. 30, 1703657. doi: 10.1002/adma.201703657
Liu, S., Han, W., Cui, B., Liu, X., Zhao, F., Stuart, J., et al. (2017). A novel rechargeable zinc-air battery with molten salt electrolyte. J. Power Sources 342, 435–441. doi: 10.1016/j.jpowsour.2016.12.080
Ma, L., Chen, S., Wang, D., Yang, Q., Mo, F., Liang, G., et al. (2019). Super-stretchable zinc-air batteries based on an alkaline-tolerant dual-network hydrogel electrolyte. Adv. Energy Mater. 9:1803046. doi: 10.1002/aenm.201803046
MacKenzie, J. D., and Ho, C. (2015). Perspectives on energy storage for flexible electronic systems. P. IEEE 103, 535–553. doi: 10.1109/JPROC.2015.2406340
Mainar, A. R., Iruin, E., Colmenares, L. C., Kvasha, A., de Meatza, I., Bengoechea, M., et al. (2018). An overview of progress in electrolytes for secondary zinc-air batteries and other storage systems based on zinc. J. Energy Storage 15, 304–328. doi: 10.1016/j.est.2017.12.004
Mainar, R. A, Leonet, O., Bengoechea, M., Boyano, I., de Meatza, I., et al. (2016). Alkaline aqueous electrolytes for secondary zinc-air batteries: an overview. Int. J. Energy Res. 40, 1032–1049. doi: 10.1002/er.3499
Masri, M. N., and Mohamad, A. A. (2013). Effect of adding carbon black to a porous zinc anode in a zinc-air battery. J. Electrochem. Soc. 160, A715–A721. doi: 10.1149/2.007306jes
Miao, H., Chen, B., Li, S., Wu, X., Wang, Q., Zhang, C., et al. (2020). All-solid-state flexible zinc-air battery with polyacrylamide alkaline gel electrolyte. J. Power Sources 450:227653. doi: 10.1016/j.jpowsour.2019.227653
Pan, Z., Yang, J., Zang, W., Kou, Z., Wang, C., Ding, X., et al. (2019). All-solid-state sponge-like squeezable zinc-air battery. Energy Storage Mater. 23, 375–382. doi: 10.1016/j.ensm.2019.04.036
Park, J., Park, M., Nam, G., Lee, J. S., and Cho, J. (2015). All-solid-state cable-type flexible zinc-air battery. Adv. Mater. 27, 1396–1401. doi: 10.1002/adma.201404639
Parker, J. F., Chervin, C. N., Nelson, E. S., Rolison, D. R., and Long, J. W. (2014). Wiring zinc in three dimensions re-writes battery performance—dendrite-free cycling. Energy Environ. Sci. 7, 1117–1124. doi: 10.1039/C3EE43754J
Pedicini, C., Sieminski, D. P., Skeggs, L. T., Young, J. E., and Cherry, E. C. (1996). Air manager system for recirculating reactant air in a metal-air battery. U.S. Patent No. 5,560,999. Washington, DC: U.S. Patent and Trademark Offic patent application.
Pedicni, C. S. (2002). Load responsive air door for an electrochemical cell. U.S. Patent No. 6,350,537. Washington, DC: U.S. Patent and Trademark Office patent application.
Pei, P., Wang, K., and Ma, Z. (2014). Technologies for extending zinc–air battery's cyclelife: a review. Appli. Energy 128, 315–324. doi: 10.1016/j.apenergy.2014.04.095
Pozo-Gonzalo, C., Virgilio, C., Yan, Y., Howlett, P. C., Byrne, N., MacFarlane, D. R., et al. (2014). Enhanced performance of phosphonium based ionic liquids towards 4 electrons oxygen reduction reaction upon addition of a weak proton source. Electrochem. Commun. 38, 24–27. doi: 10.1016/j.elecom.2013.10.004
Schröder, D., Borker, N. N. S., König, M., and Krewer, U. (2015). Performance of zinc air batteries with added K2CO3 in the alkaline electrolyte. J. Appl. Electrochem. 45, 427–437. doi: 10.1007/s10800-015-0817-0
See, D. M., and White, R. E. (1997). Temperature and concentration dependence of the specific conductivity of concentrated solutions of potassium hydroxide. J. Chem. Eng. Data 42, 1266–1268. doi: 10.1021/je970140x
Shinde, S. S., Lee, C. H., Jung, J.-Y., Wagh, N. K., Kim, S.-H., Kim, D.-H., et al. (2019). Unveiling dual-linkage 3D hexaiminobenzene metal–organic frameworks towards long-lasting advanced reversible Zn-air batteries. Energy Environ. Sci. 12, 727–738. doi: 10.1039/c8ee02679c
Simons, T., Torriero, A., Howlett, P., Macfarlane, D. R., and Forsyth, M. (2012). High current density, efficient cycling of Zn2+ in 1-ethyl-3-methylimidazolium dicyanamide ionic liquid: The effect of Zn2+ salt and water concentration. Electrochem. Commun. 18, 119–122. doi: 10.1016/j.elecom.2012.02.034
Tan, P., Chen, B., Xu, H., Zhang, H., Cai, W., Ni, M., et al. (2017). Flexible Zn-and Li-air batteries: Recent advances, challenges, and future perspectives. Energy Environ. Sci. 10, 2056–2080. doi: 10.1039/c7ee01913k
Wang, K., Pei, P., Ma, Z., Xu, H., Li, P., and Wang, X. (2014). Morphology control of zinc regeneration for zinc-air fuel cell and battery. J. Power Sources 271, 65–75. doi: 10.1016/j.jpowsour.2014.07.182
Wang, W., Tang, M., Zheng, Z., and Chen, S. (2019). Alkaline polymer membrane-based ultrathin, flexible, and high-performance solid-state Zn-air battery. Adv. Energy Mater. 9, 1803628. doi: 10.1002/aenm.201803628
Wang, X., Sunarso, J., Lu, Q., Zhou, Z., Dai, J., Guan, D., et al. (2020). High-performance platinum-perovskite composite bifunctional oxygen electrocatalyst for rechargeable Zn-air battery. Adv. Energy Mater. 10:1903271. doi: 10.1002/aenm.201903271
Wu, G., Lin, S., and Yang, C. (2006). Alkaline Zn-air and Al-air cells based on novel solid PVA/PAA polymer electrolyte membranes. J. Membr. Sci. 280, 802–808. doi: 10.1016/j.memsci.2006.02.037
Xiang, H., Yin, B., Wang, H., Lin, H., Ge, X., Xie, S., et al. (2010). Improving electrochemical properties of room temperature ionic liquid (RTIL) based electrolyte for Li-ion batteries. Electrochim. Acta 55, 5204–5209. doi: 10.1016/j.electacta.2010.04.041
Xu, L., Liu, J., Chen, P., Wang, Z., Tang, D., Liu, X., et al. (2020). High-power aqueous Zn-H2O2 batteries for wide applications. Cell Rep. Phys. Sci. 1:100027. doi: 10.1016/j.xcrp.2020.100027
Xu, M., Ivey, D., Xie, Z., and Qu, W. (2015). Rechargeable Zn-air batteries: Progress in electrolyte development and cell configuration advancement. J. Power Sources 283, 358–371. doi: 10.1016/j.jpowsour.2015.02.114
Xu, N., Zhang, Y., Wang, M., Fan, X., Zhang, T., Peng, L., et al. (2019). High-performing rechargeable/flexible zinc-air batteries by coordinated hierarchical Bi-metallic electrocatalyst and heterostructure anion exchange membrane. Nano Energy 65:104021. doi: 10.1016/j.nanoen.2019.104021
Yan, Z., Wang, E., Jiang, L., and Sun, G. (2015). Superior cycling stability and high rate capability of three-dimensional Zn/Cu foam electrodes for zinc-based alkaline batteries. RSC Adv. 5, 83781–83787. doi: 10.1039/C5RA16264E
Yang, H., Cao, Y., Ai, X., and Xiao, L. (2004). Improved discharge capacity and suppressed surface passivation of zinc anode in dilute alkaline solution using surfactant additives. J. Power Sources 128, 97–101. doi: 10.1016/j.jpowsour.2003.09.050
Yu, M., Wang, Z., Hou, C., Wang, Z., Liang, C., Zhao, C., et al. (2017). Nitrogen-doped Co3O4 mesoporous nanowire arrays as an additive-free air-cathode for flexible solid-state Zinc-air batteries. Adv. Mater. 29:1602868. doi: 10.1002/adma.201602868
Zeller, R. A. (2011). Effects Of Extrinsic And Intrinsic Proton Activity On The Mechanism Of Oxygen Reduction In Ionic Liquids. (Temp, AZ: Arizona State University, ProQuest Dissertations Publishing).
Zhong, X., Yi, W., Qu, Y., Zhang, L., Bai, H., Zhu, Y., et al. (2020). Co single-atom anchored on Co3O4 and nitrogen-doped active carbon toward bifunctional catalyst for zinc-air batteries. Appl. Catal., B 260, 118188. doi: 10.1016/j.apcatb.2019.118188
Keywords: Zn–air battery, electrolyte, alkaline electrolytes, room temperature ionic liquid, quasi-solid flexible electrolyte
Citation: Chen P, Zhang K, Tang D, Liu W, Meng F, Huang Q and Liu J (2020) Recent Progress in Electrolytes for Zn–Air Batteries. Front. Chem. 8:372. doi: 10.3389/fchem.2020.00372
Received: 10 March 2020; Accepted: 08 April 2020;
Published: 26 May 2020.
Edited by:
Changzhou Yuan, University of Jinan, ChinaReviewed by:
Xihong Lu, Sun Yat-sen University, ChinaXien Liu, Qingdao University of Science and Technology, China
Copyright © 2020 Chen, Zhang, Tang, Liu, Meng, Huang and Liu. This is an open-access article distributed under the terms of the Creative Commons Attribution License (CC BY). The use, distribution or reproduction in other forums is permitted, provided the original author(s) and the copyright owner(s) are credited and that the original publication in this journal is cited, in accordance with accepted academic practice. No use, distribution or reproduction is permitted which does not comply with these terms.
*Correspondence: Jiehua Liu, bGl1amhAaGZ1dC5lZHUuY24=