- 1College of Chemistry and Chemical Engineering, Chongqing University, Chongqing, China
- 2College of Physics, Chongqing University, Chongqing, China
Layered NbS2, a member of group-V transition metal dichalcogenides, was synthesized via a colloidal synthesis method and employed as a negative material for a supercapacitor. The morphologies of NbS2 can be tuned from ultrathin nanosheets to hierarchical structures through dynamics controls based on growth mechanisms. Electrochemical energy storage measurements present that the ultrathin NbS2 electrode exhibits the highest rate capability due to having the largest electrochemical surface area and its efficient ion diffusion. Meanwhile, the hierarchical NbS2 shows the highest specific capacitance at low current densities for small charge transfer resistance, displays 221.4 F g−1 at 1 A g−1 and 117.1 F g−1 at 10 A g−1, and cycling stability with 78.9% of the initial specific capacitance after 10,000 cycles. The aggregate or stacking of nanosheets can be suppressed effectively by constructing hierarchical structure NbS2 nanosheets.
Introduction
Layered transition-metal dichalcogenides (TMDs), which have a X-M-X sandwich structure (generalized formula: MX2, M = transition metal element; X = S, Se, or Te) in each layer, have attracted extensive attention due to their great potential for applications in energy storage (Chhowalla et al., 2013; Muller et al., 2015; Lin et al., 2019), catalysis (Yan et al., 2014; Yang et al., 2019), electronics (Wang et al., 2012; Lin et al., 2018), photonics (Mak and Shan, 2016; Linhart et al., 2019) etc. Recently, significant progress has been made on TMDs materials for energy storage applications (Han et al., 2018; Yun et al., 2020). However, a crucial issue regarding practical use is the aggregation or restacking of the individual layers with high surface energy by interlayer van der Waals forces (Chhowalla et al., 2013), which can cause low coulombic efficiency and capacity degradation irreversibly (Mei et al., 2018).
To solve the aggregation or restacking problem, some strategies to optimize the structure of TMDs materials have been proposed in previous studies. One strategy is to construct TMDs/conductor composites, e.g., grapheme (Li et al., 2011; Yang et al., 2017), carbon nanotubes (Wang et al., 2019), carbon nanofibers (Cha et al., 2017), organic polymers (Cho et al., 2019), etc., which can suppress the aggregation of layered TMDs effectively. Another important strategy is to adjust the structure parameters in the synthesis process. For instance, to tune the size and thickness of nanosheets (Yin and Alivisatos, 2005; Yoo et al., 2014; Mansouri and Semagina, 2018), increase the layer-to-layer spacing (Jang et al., 2011), or construct hierarchical architectures (Sun et al., 2014; Cong et al., 2017; Zhang J. et al., 2017).
Among layered TMDs, group-V 2H-NbS2 has an intrinsic metallic character owing to the half-filled 4orbital (Kuc et al., 2011). The good electric conductivity and preferable flexibility of 2H-NbS2 are practical for applications in energy storage (Han et al., 2018). However, the control of the morphologies, growth mechanism, and the structure-property relationship still need to be further explored. Therefore, this paper regulated the reaction dynamics of the colloidal synthesis process of 2H-NbS2 by adjusting growth temperature and the amount of carbon disulfide, obtaining different morphologies of NbS2, such as ultrathin nanosheets, self-assembled hierarchical structures, and layer stacked nanosheets. Furthermore, the growth mechanism was illustrated, and the effect of morphologies on supercapacitor performance was investigated.
Experimental Section
Chemicals
Niobium (V) chloride (NbCl5, 99.9%), carbon disulfide (CS2, 99.9%), and oleylamine (80–90%) were purchased from Aladdin Ltd. Potassium chloride (KCl), Hexane, n-butanol, and absolute ethanol were of analytical grade and all obtained from Chuan Dong Ltd.
Synthesis of NbS2 Nanosheets
A slight modified colloidal synthesis method is used to synthesize NbS2 nanosheets (Jeong et al., 2012). First, NbCl5 (1 mmol, 0.27 g) and oleylamine (24.3 mmol, 8.0 mL) was added to a 100 mL three-neck flask under Ar atmosphere. Next, the mixture was treated under ultrasound until completely dissolved of NbCl5 to form yellow transparent liquid. Then the mixture was heated to 120°C for 30 min under an Ar flow to remove water and oxygen. After the solution was heated to 300°C, CS2 (30 mmol, 1.8 mL) was injected dropwise into the hot solution at a speed of ~1 mL/min. The reaction proceeded at 300°C for 2 h before being stopped by removal from the heating mantle. The NbS2 nanosheets were precipitated by addition of excess butanol, and washed by mixed solution of hexane and absolute ethanol (1:1), and DI water for several times. Finally, the brownish black NbS2 powder was obtained by a freeze-drying method. To regulate the morphologies of NbS2, the reaction temperature was set at 280, 300, and 320°C, and the CS2 amount was adjusted as 10, 30, and 60 mmol, respectively, and other experiment parameters remained unchanged.
Material Characterization
Scanning electron microscopy (SEM) images were taken on a field-emission scanning electron microscope (FESEM, FEI Nova 400 Nano-SEM). A high-resolution transmission electron microscope (HRTEM, ThermoFisher Scientific, Talos F200s) was performed to analyze the structures and elemental mapping. X-ray diffraction (XRD) patterns of the as-synthesized NbS2 were recorded on a diffractometer with Cu Kα radiation (Spectris Pte. Ltd, PANalytical X' Pert Powder). Chemical states of the containing elements were analyzed by X-ray photoelectron spectroscopy (XPS, Thermo Scientific ESCALAB 250Xi, K-alpha). Fourier transform infrared (FTIR) spectrometer (ThermoFisher Scientific, Nicolet iS50) was used to analyze the composition of the samples.
Electrochemical Measurement
The electrochemical performance of NbS2 nanosheets for the supercapacitor was measured by using a three-electrode cell system at room temperature. Specifically, the active material (70 wt%), conductive carbon black (20 wt%), and polyvinylidene fluoride binder (10 wt%) were mixed and turned into slurry uniformly, using absolute ethanol. Then the slurry was coated onto the pre-cleaned nickel foam with mass loading of active material ~1.5 mg cm−2. After being dried in a vacuum oven at 60 °C overnight, the nickel foam coated with active material and another pre-cleaned nickel foam were overlapped and pressed at 10 MPa and used as a working electrode. A platinum foil and an Ag/AgCl (saturated KCl) electrode were used as the counter and reference electrode, respectively. The electrochemical performance was tested in 1 M KCl electrolyte solutions. Cyclic voltammetry (CV), galvanostatic charge-discharge (GCD) curves and electrochemical impedance spectroscopy (EIS) were tested using an electrochemical workstation (CHI660E, CH Instruments). Cycling stability tests were carried out on an electrochemical instrument (LAND).
Results and Discussion
Physical Characterization of NbS2 Nanosheets
The morphologies of NbS2 nanosheets can be tuned by adjusting the reaction conditions. The lateral size, thickness, and assembly mode of NbS2 nanosheets varied with the reaction temperature and the amount of carbon disulfide (CS2), as observed by SEM images [Figure 1 (high magnification), Figure S1 (low magnification)]. The materials exhibited large lateral size and ultrathin nanosheets when using 10 mmol CS2 as the sulfur source at a reaction temperature of 280, 300, and 320°C (Figures 1a–c, Figures S1a–c). The lateral size of the nanosheets was ~2 μm at 280°C, ~3 μm at 300°C, and ~4 μm at 320°C, and showed an increasing trend with reaction temperature. Scrolled structures could be observed at the edges of the ultrathin films. The nanosheets formed unavoidable wrinkles as a self-folding phenomenon to balance adhesion energy and bending strain energy, which can cause the intrinsic lattice structures to distort (Chen et al., 2019).
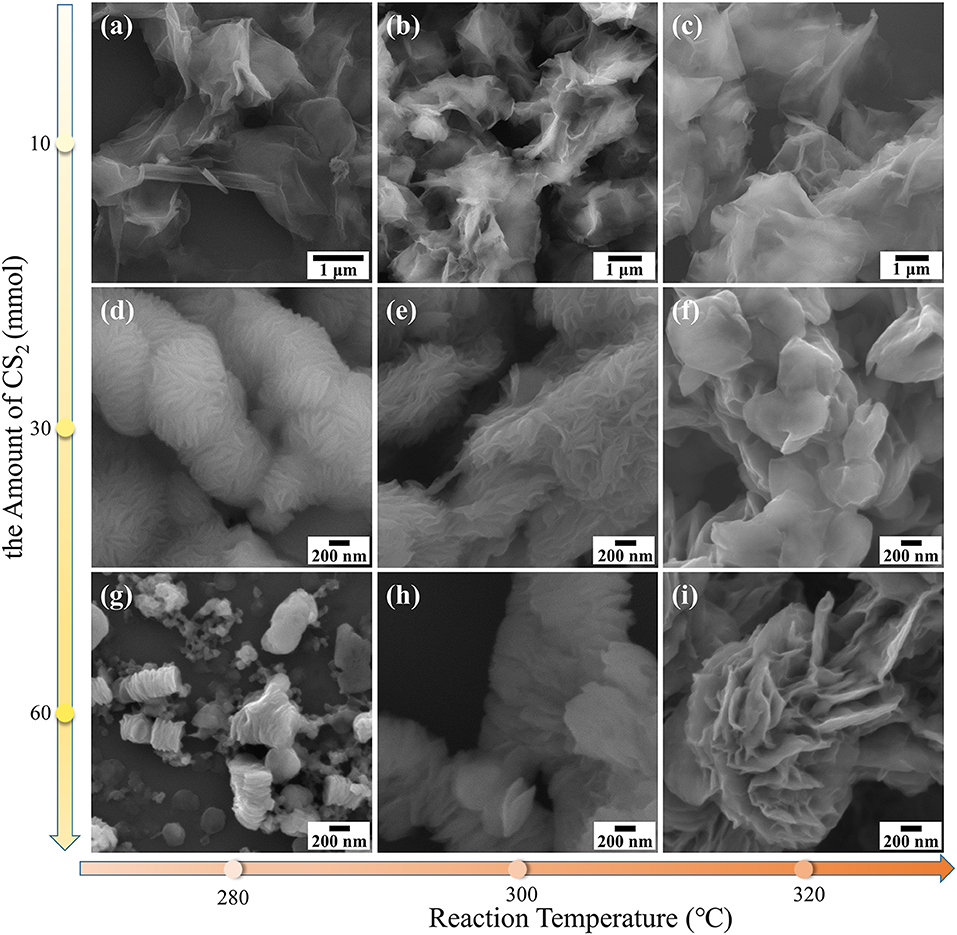
Figure 1. SEM images of NbS2 nanosheets synthesized at different reaction temperatures and carbon disulfide (CS2) amounts at high magnification. (a) 280°C, 10 mmol CS2. (b) 300°C, 10 mmol CS2. (c) 320°C, 10 mmol CS2. (d) 280°C, 30 mmol CS2. (e) 300°C, 30 mmol CS2. (f) 320°C, 30 mmol CS2. (g) 280°C, 60 mmol CS2. (h) 300°C, 60 mmol CS2. (i) 320°C, 60 mmol CS2.
With the increase of the amount of CS2, the NbS2 nanosheets tended to assemble to form hierarchical or stacked structures, including both vertically assembled (face-to- face) and laterally gathered (edge-to-edge) ways (Zhang X. et al., 2017), and the lateral size showed a decreasing trend while the thickness increased. Under the reaction condition of 300°C and 30 mmol CS2, the materials grew to hierarchical structures, composed of nanosheets with lateral sizes of ~700 nm (hierarchical NbS2, Figure 1e, Figure S1e). When the amount of CS2 was increased to 60 mmol at 300 °C, the NbS2 structures was assembled by layer-stacked nanodisks, which lateral size reduced to ~500 nm (stacked NbS2, Figure 1h, Figure S1h). At 280 °C and 60 mmol CS2 conditions, the nanodisks with diameters of ~300 nm were mainly vertically assembled, like granum that stack thylakoids (Figure 1g, Figure S1g). At 320°C and 60 mmol CS2 conditions, the nanosheets with diameters of ~1 μm formed flower-like structures (Figure 1i, Figure S1i). Overall, the lateral size and thickness of NbS2 nanosheets increased with the temperature. With the increasing amount of CS2, the lateral size decreased and the thickness increased.
To simplify the study, the ultrathin, hierarchical, and stacked NbS2 nanosheets in the following test results of this essay refer to the samples synthesized using 10, 30, and 60 mmol CS2 at 300°C, corresponding to Figures 1b,e,h respectively.
The TEM images of the NbS2 nanosheets are shown in Figure 2. The lateral size of the ultrathin NbS2 nanosheets was about 3 μm (Figure 2a). The lattice fringes of the ultrathin nanosheet presented in HRTEM images indicate the as-prepared NbS2 was in 2H phase (Figure 2b). The interplanar spacings of 2.8 and 1.7 Å corresponded to the (100) and (110) plane of 2H-NbS2 (Jeong et al., 2012). The diffraction rings in the selected area electron diffraction (SAED) pattern illustrated a polycrystalline nature, which can be assigned to (100), (110), and (200) planes, respectively (Figure 2c). The element mapping of the scanning transmission electron microscope-energy dispersive spectroscope (STEM-EDS) presented the uniform distributions of Nb and S in the nanosheets (Figures 2d–f). Figures 2g–i show the edges of ultrathin, hierarchical, and stacked nanosheets, corresponding to Figures 1b,e,h, respectively. The enlarged layer-to-layer spacings of ultrathin and hierarchical nanosheets were ~1.5 nm, indicating the existence of OLA molecules among layers (Jang et al., 2011). The 0.6 nm spacing of stacked nanosheets was consistent with the (002) lattice plane, while the 1.0 nm spacing could also be expanded by OLA molecules. The inset pattern in Figure 2i shows the TEM images of stacked NbS2 nanosheets. The thickness of the NbS2 nanosheets increased with the amount of CS2, from 10, 20, to 60 nm.
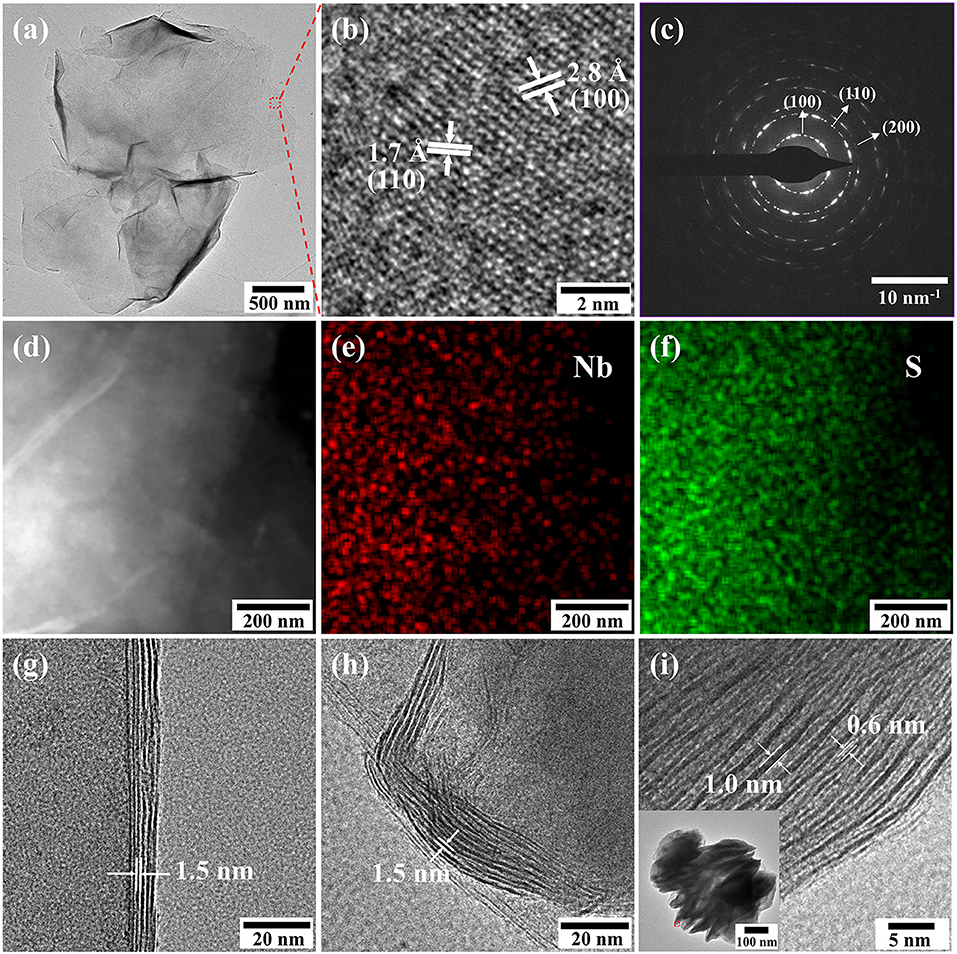
Figure 2. (a) TEM images, (b) HRTEM images and (c) SAED pattern of ultrathin NbS2 nanosheets. (d–f) Element mapping of ultrathin NbS2 nanosheets. (g–i) Edges of ultrathin, hierarchical and stacked NbS2 nanosheets. Inset: TEM images of stacked NbS2 nanosheets.
The crystalline characteristics of the various NbS2 samples with different morphologies were confirmed by XRD, as shown in Figure 3A. The diffraction peaks of all the as-prepared NbS2 matched with the standard pattern (JCPDS no.41-0980), the peaks at 14.8°, 31.0°, 55.3° and 64.7° could be assigned to (002), (100), (110), and (200) planes of the hexagonal crystal structure NbS2 with space group P63/mmc (Zhang J. et al., 2017), which was consistent with the lattice fringes of the HRTEM results.
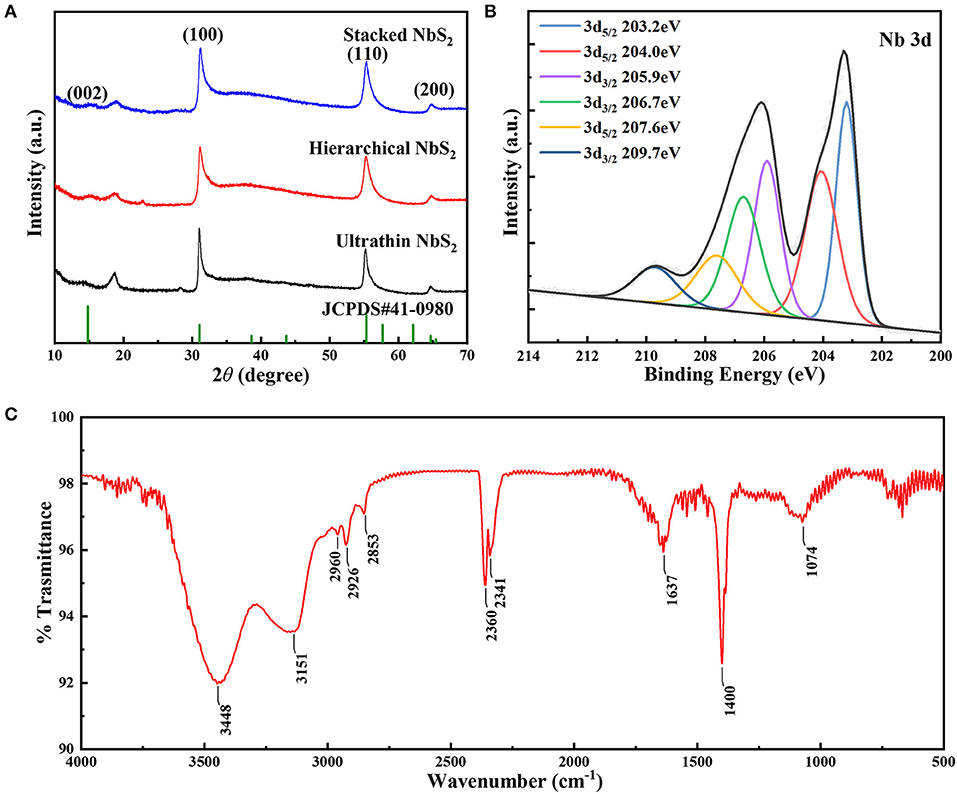
Figure 3. (A) XRD patterns of ultrathin, hierarchical and stacked NbS2 nanosheets (NbS2, JCPDS no.41-0980). (B) XPS results of Nb 3d and (C) FT-IR spectra of the hierarchical NbS2 nanosheets.
To analyze the surface electronic states of the NbS2 nanosheets, an XPS survey was carried out. Figure 3B shows the Nb 3d spectrum of the hierarchical NbS2 nanosheets. The binding energies of 3d3/2 located at 209.7 eV and 3d5/2 at 207.6 eV revealed the existence of Nb5+ (Zhang J. et al., 2017). The peaks of 3d3/2 at 206.7 eV and 3d5/2 at 204.0 eV could be assigned to Nb4+ (Zhang J. et al., 2017). The binding energies of 3d3/2 state occurring at 205.9 eV and 3d5/2 at 203.2 eV corresponded to the oxidation state of Nb(4−δ)+ (Izawa et al., 2008). The Nb 3d and S 2p XPS spectra of ultrathin, hierarchical and stacked NbS2 nanosheets were summarized in Figure S2. In the S 2p spectrum (Figure S2b), peaks of 2p2/3 and 2p1/2 at 160.6 and 161.7 eV respectively, was corresponding to S2− (Dash et al., 2015).
To examine the surfactant on the surface of the samples, an FT-IR test of hierarchical NbS2 nanosheets ranging from 500 ~ 4,000 cm−1 was conducted (Figure 3C). The FT-IR spectra proved the existence of oleylamine (OLA). The two broad absorption peaks at 3448 and 3151 cm−1 could be assigned to N–H stretching mode, and peak at 1637 cm−1 corresponded to NH2 scissoring mode (Altavilla et al., 2011; Cooper et al., 2011). The peaks at 2,960 and 2,926 cm−1 were due to asymmetrical stretching vibration of CH3, and the peak at 2,853 cm−1 was due to asymmetrical stretching vibration of CH2 (Altavilla et al., 2011). The broad peak located at 1074 cm−1 came from C–N stretching vibration mode (Gunasekaran et al., 2008). The peaks at 2,360 and 2,341 cm−1 were attributed to the adsorption doublet band of CO2. The peak located at 1,400 cm−1 could be due to CH3 symmetric bending vibration (Gunasekaran et al., 2008).
Growth Mechanism of NbS2 Nanosheets
The shape of nanocrystals is dominated by the surface energy of the facets. For some transition metal dichalcogenides, including NbS2, 2D shapes are thermodynamically favored (Jeong et al., 2012; Moon, 2018). In the synthesis process of colloidal NbS2 nanosheets, oleylamine (OLA) serves as an organic surfactant, solvent, and reducing agent, as reported in other colloidal synthesis works (Yin and Alivisatos, 2005). The reaction can be illustrated as the following steps: (1) NbCl5 and OLA formed complexes by the dissolution of NbCl5 in OLA (Nasilowski et al., 2016); (2) generation of H2S gas via the reaction of OLA with CS2 after the injection of CS2 (Yoo et al., 2014); (3) reaction of the niobium (V) with H2S to form Niobium (IV) disulfide monomers; (4) burst of nucleation of NbS2 crystals; (5) growth of NbS2 crystals to form nanosheets (Figure 4).
Temperature is an important factor for crystal growth as it can change dynamics of ions and atoms in solution, and also alter the probability of effective collision between atoms. The adhesion of OLA molecules as surfactant to the NbS2 nanocrystal surfaces can form NbS2 – OLA complexes, and the stability and motion rate can be adjusted by the growth temperature. The OLA molecules should be capable of exchanging on and off the surface of growing crystals for instantaneous growth (Yin and Alivisatos, 2005). As the temperature rises, the stability of the complexes reduces, and OLA molecules have a higher probability of leaving the NbS2 nanocrystals, then monomers are more likely to access and add to the nanocrystal surface—thus the thickness and lateral size of the nanosheets increase, which is consistent with the experiment results.
The morphology changes, including lateral size and thickness, caused by the dose of CS2 can be explained by the following two reasons. On one hand, the amount of CS2 injected into the reaction system may change the monomer concentration. According to “size-distribution focusing” theory (Yin and Alivisatos, 2005), the critical size of nanocrystals diminishes instantly after injection of precursor for a second time due to the burst of monomers. In the synthesis process of NbS2 nanocrystals in our experiment, CS2 was injected slowly (about 0.1 mL/min), so the higher amount of CS2, the longer the injection time is. Based on the condition that an excess of OLA acts as a reducing agent, and the quick enough reduction of CS2 by OLA, as well as the rapid reaction between NbCl5 and H2S, it is hypothesized that the generation of monomers occur instantly and continuously during the CS2 injection period, and the critical size of NbS2 nanocrystals become smaller steadily. On the other hand, owing to crystal surface energy differences and their distinct reactive activity toward sulfur, an increase in the amount of CS2 promotes vertical growth of anisotropic crystal (Mansouri and Semagina, 2018), and the thickness of nanosheets increases.
The assembly or aggregation of layered nanosheets can be influenced by the surface passivation and polarity of solvent, owing to the combined effect of solvation and cohesive energy (Zhang X. et al., 2017). The NbS2 nanosheets tend to assemble or aggregate with the CS2 amount increase, the possible reason is that more CS2 or H2S molecules adsorb to the surface of NbS2 nanosheets in the reaction solution, and occupy the sites of OLA molecules, thus the surface energy of NbS2 increases, and an assemble or aggregate phenomenon can more easily to occur.
Electrochemical Performance of NbS2 Nanosheets
To compare the electrochemical performance differences of NbS2 nanosheets with diverse morphologies, the electrochemical tests of ultrathin, hierarchical, and stacked NbS2 nanosheets were investigated as negative electrode materials for a supercapacitor using a three-electrode electrolytic cell in neutral 1 M KCl aqueous solution (Figure 5). The CV curves of three samples all show quasi-rectangular shapes at scan rates of 10 and 100 mV s−1 (Figures 5A,B). There existed weak redox peaks at −0.82 V and −0.78 V (vs. Ag/AgCl) for the ultrathin sample at scan rate of 10 mV s−1, which may be due to faster ion diffusion than the other two samples. The operating voltage windows of galvanostatic charge-discharge (GCD) curves was −0.3 ~ −1V (vs. Ag/AgCl) for ultrathin and hierarchical NbS2 electrodes, and −0.35 ~ −1V (vs. Ag/AgCl) for stacked NbS2 electrodes because its discharge process was sluggish as the potential came near to −0.3 V (vs. Ag/AgCl) at the current density of 1 A g−1 (Figure 5C). The GCD curves of the ultrathin and hierarchical NbS2 electrodes performed a good symmetric triangular shape at 1 A g−1, suggesting its rapid and reversible charge-discharge ability (Liu et al., 2018a; Li et al., 2019), while the stacked NbS2 electrode showed slow charge process when the potential approached to −1.0 V (vs. Ag/AgCl) (Figure 5C). The specific capacitance can be calculated using Equation (S1) or Equation (S2) from Supplementary Material. The ultrathin NbS2 electrode had the highest rate capability, and displayed 120.0 F g−1 at 10 A g−1. However, the hierarchical NbS2 electrode showed the highest specific capacitance at low current densities (1 to 7 A g−1), displaying 221.4 F g−1 at 1 A g−1 (Figure 5D). The electrochemical energy storage performance of the stacked NbS2 electrode was poorer than the former two samples at high current densities.
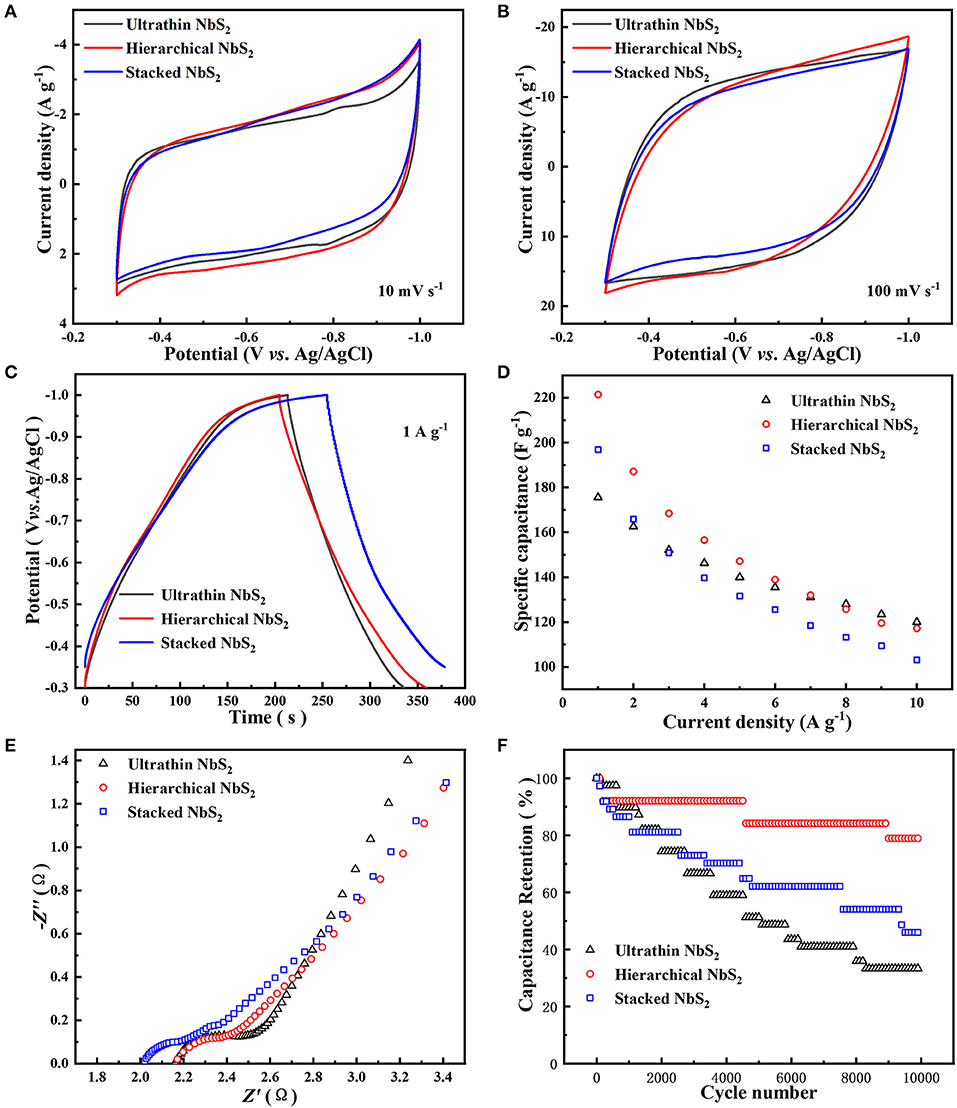
Figure 5. Electrochemical performance of ultrathin, hierarchical and stacked NbS2 nanosheets toward supercapacitor using a three-electrode electrolytic cell in neutral 1 M KCl aqueous solution. (A) CV curves at 10 mV s−1. (B) CV curves at 100 mV s−1. (C) GCD curves at current density of 1 A g−1. (D) Specific capacitance at different current densities. (E) EIS spectrum. (F) Cycling stability at 10 A g−1 for 10,000 cycles.
To estimate the ion accessible surface area of NbS2 nanosheets electrodes, the electrochemical surface area (ECSA) was evaluated using cyclic voltammetry measurement (Figure S3). The electrochemical double layer capacitance (CEDL) of the ultrathin, hierarchical, and stacked NbS2 electrodes were 206.6, 195.8, and 165.5 mF respectively. The CEDL of the ultrathin NbS2 electrode was the highest among three samples, while its specific capacitance was the lowest in low current densities (1 and 2 A g−1), demonstrating the ECSA was not the only affected factor for the performance of specific capacitance. The ECSA may play a more important role in the rapid charge and discharge process as the specific capacitance of ultrathin NbS2 electrodes was the highest in high current densities (8, 9, and 10 A g−1).
The EIS test was carried out to understand the internal resistance of the various NbS2 electrodes and interactions of the electrode/electrolyte interface. As shown in Figure 5E, the Nyquist plots displayed semicircles in the high frequency region, relating to charge transfer resistance (Rct) (Liu et al., 2018b). The Rct value of the hierarchical NbS2 electrode was smaller than the ultrathin one, reflecting the easier process of charge transfer, which was coherent with the results that the specific capacitance of the former electrode was higher than the latter one in low current densities (Figure 5D). The slope of the ultrathin NbS2 electrode was the steepest among the three samples in the low frequency region, indicating more efficient ion diffusion, which was consistent with the higher rate capability shown in Figure 5D.
To test the potential application of NbS2 in practical uses, the cycling stability test was carried out at current density of 10 A g−1. The hierarchical NbS2 electrode exhibited capacitance retention with 78.9% of the initial specific capacitance after 10 000 cycles—much better than the ultrathin and stacked NbS2 electrodes (Figure 5F). The structure of the hierarchical NbS2 sample maintained well after the stability test, indicating that decay of capacitance may mainly be due to the exfoliation of partial active materials from the electrode (Figure S4). However, both the ultrathin and stacked NbS2 nanosheets aggregated to some extent, consistent with poor stability.
As the hierarchical NbS2 electrode showed better overall electrochemical performance than the ultrathin or stacked NbS2 electrode, and the capacitive characteristics of the three electrodes are similar, we only discuss the capacitive performance of the hierarchical NbS2 electrode in more detail here. The capacitive performance of ultrathin and stacked NbS2 electrodes can be obtained from supporting information (Figure S6). Figure 6A shows the CV curves of the hierarchical NbS2 electrode tested at scan rates from 10 to 100 mV s−1 in the potential range of −0.3 ~ −1V (vs. Ag/AgCl), which displayed quasi-rectangular shapes, indicating the electrochemical double layer capacitance (EDLC) mechanism primarily (Peng et al., 2019). The hierarchical NbS2 electrode material occasioned oxidation reaction when the operating voltage window was broadened to −0.2 ~ −1 V and −0.1 ~ −1V (vs. Ag/AgCl), as the CV curves at 50 mV s−1 shows (Figure S5).
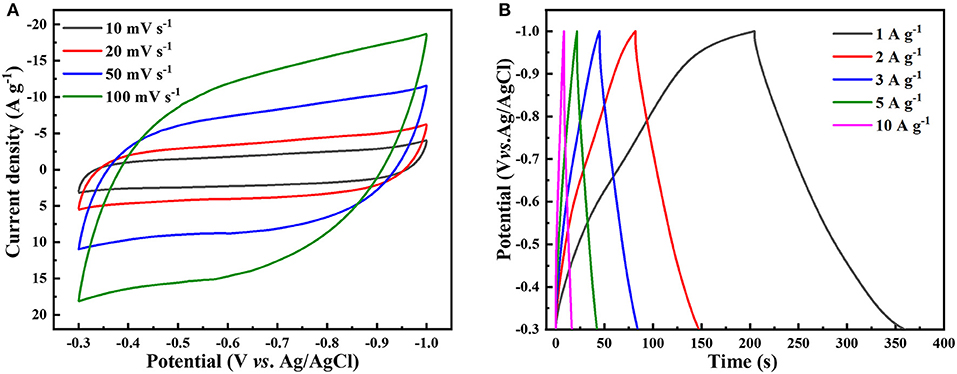
Figure 6. Capacitive performance of hierarchical NbS2 nanosheets toward supercapacitor using a three-electrode electrolytic cell in neutral 1 M KCl aqueous solution. (A) CV curves at different scan rates. (B) GCD curves at different current density.
The GCD curves of NbS2 electrodes indicated the capacitive behavior at different current densities. The near-triangular GCD curves of the hierarchical NbS2 recorded from 1 to 10 A g−1 are shown in Figure 6B, exhibiting good specific capacitance of 221.4 F g−1 at 1 A g−1 and 117.1 F g−1 at 10 A g−1, respectively. The CV curves and the GCD curves of the ultrathin and stacked NbS2 are presented in Figure S6. The specific capacitance of ultrathin NbS2 was 175.6 F g−1 at 1 A g−1 and 120.0 F g−1 at 10 A g−1, respectively. And the specific capacitance of stacked NbS2 was 196.9 F g−1 at 1 A g−1 and 103.1 F g−1 at 10 A g−1, respectively.
From the electrochemical analysis, the hierarchical NbS2 electrode showed great overall electrochemical performance for energy storage. Fast and efficient electron transfer of the electrode can be achieved owing to the small charge transfer resistance. Furthermore, the restacking or aggregation of nanosheets can be suppressed by constructing a hierarchical structure, and the electrochemical active materials can be fully utilized during long-term charging and discharging processes.
Conclusions
In summary, we introduce a colloidal synthesis method to regulate the morphologies of colloidal NbS2 nanocrystals via dynamics control. The size, thickness, and structure of nanocrystals can be adjusted by controlling the growth temperature and CS2 amount. The ultrathin NbS2 nanosheets show more efficient ion diffusion in the application of supercapacitor, while the self-assembled hierarchical structure NbS2 can suppress the restacking or aggregation of nanosheets effectively. This work offers insight into morphology regulation of layered TMDs in the synthesis process. The self-assembling mechanism has not yet been fully understood and needs to be investigated in future research.
Data Availability Statement
All datasets generated for this study are included in the article/Supplementary Material.
Author Contributions
WL (Investigation: Lead, Methodology: Lead, Writing – original draft: Lead, Writing – review and editing: Lead). XW (Investigation: Supporting, Writing – review and editing: Supporting). HD and YO (Writing – review and editing: Supporting). SX and YY (Software: Supporting). PX and YZ (Supervision: Lead, Writing – review and editing: Lead).
Funding
This work was supported by the Project of State Key Laboratory of Environment Friendly Energy Materials, the Southwest University of Science and Technology (No. 17FKSY0115).
Conflict of Interest
The authors declare that the research was conducted in the absence of any commercial or financial relationships that could be construed as a potential conflict of interest.
Supplementary Material
The Supplementary Material for this article can be found online at: https://www.frontiersin.org/articles/10.3389/fchem.2020.00189/full#supplementary-material
References
Altavilla, C., Sarno, M., and Ciambelli, P. (2011). A novel wet chemistry approach for the synthesis of hybrid 2D free-floating single or multilayer nanosheets of MS2@oleylamine (M=Mo, W). Chem. Mater. 23, 3879–3885. doi: 10.1021/cm200837g
Cha, J.- H., Choi, S.- J., Yu, S., and Kim, I.- D. (2017). 2D WS2-edge functionalized multi-channel carbon nanofibers: effect of WS2 edge-abundant structure on room temperature NO2 sensing. J. Mater. Chem. A 5, 8725–8732. doi: 10.1039/C6TA11019C
Chen, W., Gui, X., Yang, L., Zhu, H., and Tang, Z. (2019). Wrinkling of two-dimensional materials: methods, properties and applications. Nanoscale Horiz. 4, 291–320. doi: 10.1039/C8NH00112J
Chhowalla, M., Shin, H. S., Eda, G., Li, L. J., Loh, K. P., and Zhang, H. (2013). The chemistry of two-dimensional layered transition metal dichalcogenide nanosheets. Nat. Chem. 5, 263–275. doi: 10.1038/nchem.1589
Cho, K., Pak, J., Chung, S., and Lee, T. (2019). Recent advances in interface engineering of transition-metal dichalcogenides with organic molecules and polymers. ACS Nano 13, 9713–9734. doi: 10.1021/acsnano.9b02540
Cong, L., Xie, H., and Li, J. (2017). Hierarchical structures based on two-dimensional nanomaterials for rechargeable lithium batteries. Adv. Energy Mater. 7:1601906. doi: 10.1002/aenm.201601906
Cooper, J. K., Franco, A. M., Gul, S., Corrado, C., and Zhang, J. Z. (2011). Characterization of primary amine capped CdSe, ZnSe, and ZnS Quantum dots by FT-IR: determination of surface bonding interaction and identification of selective desorption. Langmuir 27, 8486–8493. doi: 10.1021/la201273x
Dash, J. K., Chen, L., Dinolfo, P. H., Lu, T.-M., and Wang, G.-C. (2015). A method toward fabricating semiconducting 3R-NbS2 ultrathin films. J. Phys. Chem. C 119, 19763–19771. doi: 10.1021/acs.jpcc.5b04057
Gunasekaran, S., Natarajan, R. K., Renganayaki, V., and Rathikha, R. (2008). FTIR and UV visible spectrophotometric approach to discriminate leukemic sera. Asian J. Chem. 20, 2521–2530.
Han, J. H., Kim, H. K., Baek, B., Han, J., Ahn, H. S., Baik, M., et al. (2018). Activation of the basal plane in two dimensional transition metal chalcogenide nanostructures. J. Am. Chem. Soc. 140, 13663–13671. doi: 10.1021/jacs.8b05477
Izawa, K., Ida, S., Unal, U., Yamaguchi, T. J., Kang, H. J., Choy, H., et al. (2008). A new approach for the synthesis of layered niobium sulfide and restacking route of NbS2 nanosheet. J. Solid State Chem. 181, 319–324. doi: 10.1016/j.jssc.2007.12.002
Jang, J. T., Jeong, S., Seo, J. W., Kim, M. C., Sim, E., Oh, Y., et al. (2011). Ultrathin zirconium disulfide nanodiscs. J Am Chem Soc. 133, 7636–7639. doi: 10.1021/ja200400n
Jeong, S., Yoo, D., Jang, J. T., Kim, M., and Cheon, J. (2012). Well-defined colloidal 2-D layered transition-metal chalcogenide nanocrystals via generalized synthetic protocols. J. Am. Chem. Soc. 134, 18233–18236. doi: 10.1021/ja3089845
Kuc, A. B., Zibouche, N., and Heine, T. (2011). Influence of quantum confinement on the electronic structure of the transition metal sulfide TS2. Phys. Rev. B 83:245213. doi: 10.1103/PhysRevB.83.245213
Li, Y., Tang, X., Zhou, X., Li, L., and Jiang, S. (2019). Improvement of lithium ion storage in titanium dioxide nanowires by introducing interfacial capacity. Appl. Surf. Sci. doi: 10.1016/j.apsusc.2019.144649. [Epub ahead of print].
Li, Y., Wang, H., Xie, L., Liang, Y., Hong, G., and Dai, H. (2011). MoS2 nanoparticles grown on graphene: an advanced catalyst for the hydrogen evolution reaction. J. Am. Chem. Soc. 133, 7296–7299. doi: 10.1021/ja201269b
Lin, L., Lei, W., Zhang, S., Liu, Y., Wallace, G. G., and Chen, J. (2019). Two-dimensional transition metal dichalcogenides in supercapacitors and secondary batteries. Energy Storage Mater. 19, 408–423. doi: 10.1016/j.ensm.2019.02.023
Lin, Y. C., Jariwala, B., Bersch, B. M., Xu, K., Nie, Y., Wang, B., et al. (2018). Realizing large-scale, electronic-grade two-dimensional semiconductors. ACS Nano 12, 965–975. doi: 10.1021/acsnano.7b07059
Linhart, L., Paur, M., Smejkal, V., Burgdörfer, J., Mueller, T., and Libisch, F. (2019). Localized intervalley defect excitons as single-photon emitters in WSe2. Phys. Rev. Lett. 123:146401. doi: 10.1103/PhysRevLett.123.146401
Liu, X., Sheng, G., Zhong, M., and Zhou, X. (2018a). Dispersed and size-selected WO3 nanoparticles in carbon aerogel for supercapacitor applications. Mater. Des. 141, 220–229. doi: 10.1016/j.matdes.2017.12.038
Liu, X., Sheng, G., Zhong, M., and Zhou, X. (2018b). Hybrid nanowires and nanoparticles of WO3 in a carbon aerogel for supercapacitor applications. Nanoscale 10, 4209–4217. doi: 10.1039/C7NR07191D
Mak, K. F., and Shan, J. (2016). Photonics and optoelectronics of 2D semiconductor transition metal dichalcogenides. Nat. Photonics 10, 216–226. doi: 10.1038/nphoton.2015.282
Mansouri, A., and Semagina, N. (2018). Colloidal synthesis protocol of shape- and dimensionally-controlled transition-metal chalcogenides and their hydrodesulfurization activities. ACS Appl. Nano Mater. 1, 4408–4412. doi: 10.1021/acsanm.8b01353
Mei, J., Zhang, Y., Liao, T., Sun, Z., and Dou, S. X. (2018). Strategies for improving the lithium-storage performance of 2D nanomaterials. Natl. Sci. Rev. 5, 389–416. doi: 10.1093/nsr/nwx077
Moon, G. D. (2018). Anisotropic Metal Chalcogenide Nanomaterials: Synthesis, Assembly, and Applications. Busan: Springer. doi: 10.1007/978-3-030-03943-1
Muller, G. A., Cook, J. B., Kim, H. S., Tolbert, S. H., and Dunn, B. (2015). High performance pseudocapacitor based on 2D layered metal chalcogenide nanocrystals. Nano Lett. 15, 1911–1917. doi: 10.1021/nl504764m
Nasilowski, M., Mahler, B., Lhuillier, E., Ithurria, S., and Dubertret, B. (2016). Two-dimensional colloidal nanocrystals. Chem. Rev. 116, 10934–10982. doi: 10.1021/acs.chemrev.6b00164
Peng, H., Yao, B., Wei, X., Liu, T., Kou, T., Xiao, P., et al. (2019). Pore and heteroatom engineered carbon foams for supercapacitors. Adv. Energy Mater. 9:1803665. doi: 10.1002/aenm.201803665
Sun, P., Zhang, W., Hu, X., Yuan, L., and Huang, Y. (2014). Synthesis of hierarchical MoS2 and its electrochemical performance as an anode material for lithium-ion batteries. J. Mater. Chem. A 2, 3498–3504. doi: 10.1039/C3TA13994H
Wang, C., Wu, X., Xu, H., Zhu, Y., Liang, F., Luo, C., et al. (2019). VSe2/carbon-nanotube compound for all solid-state flexible inplane supercapacitor. Appl. Phys. Lett. 114. doi: 10.1063/1.5078555
Wang, Q. H., Kalantar-Zadeh, K., Kis, A., Coleman, J. N., and Strano, M. S. (2012). Electronics and optoelectronics of two-dimensional transition metal dichalcogenides. Nat. Nanotechnol. 7, 699–712. doi: 10.1038/nnano.2012.193
Yan, Y., Xia, B., Xu, Z., and Wang, X. (2014). Recent development of molybdenum sulfides as advanced electrocatalysts for hydrogen evolution reaction. ACS Catal. 4, 1693–1705. doi: 10.1021/cs500070x
Yang, J., Mohmad, A. R., Wang, Y., Fullon, R., Song, X., Zhao, F., et al. (2019). Ultrahigh-current-density Niobium disulfide catalysts for hydrogen evolution. Nat. Mater. 18, 1309–1314. doi: 10.1038/s41563-019-0463-8
Yang, P., Zhang, Z., Shi, J., Jiang, S., and Zhang, Y. (2017). Progress in controllable construction and energy-related applications of MX2/Graphene and MX2/MX2 heterostructures. ChemNanoMat 3, 340–351. doi: 10.1002/cnma.201700031
Yin, Y., and Alivisatos, A. P. (2005). Colloidal nanocrystal synthesis and the organic-inorganic interface. Nature 437, 664–670. doi: 10.1038/nature04165
Yoo, D., Kim, M., Jeong, S., Han, J., and Cheon, J. (2014). Chemical synthetic strategy for single-layer transition-metal chalcogenides. J. Am. Chem. Soc. 136, 14670–14673. doi: 10.1021/ja5079943
Yun, Q., Li, L., Hu, Z., Lu, Q., Chen, B., and Zhang, H. (2020). Layered transition metal dichalcogenide-based nanomaterials for electrochemical energy storage. Adv. Mater. 32:e1903826. doi: 10.1002/adma.201903826
Zhang, J., Du, C., Dai, Z., Chen, W., Zheng, Y., Li, B., et al. (2017). NbS2 nanosheets with M/Se (M = Fe, Co, Ni) Codopants for Li+ and Na+ Storage. ACS Nano 11, 10599–10607. doi: 10.1021/acsnano.7b06133
Keywords: colloidal synthesis, morphology regulation, niobium disulfide nanosheets, supercapacitor, transition metal dichalcogenides
Citation: Li W, Wei X, Dong H, Ou Y, Xiao S, Yang Y, Xiao P and Zhang Y (2020) Colloidal Synthesis of NbS2 Nanosheets: From Large-Area Ultrathin Nanosheets to Hierarchical Structures. Front. Chem. 8:189. doi: 10.3389/fchem.2020.00189
Received: 08 February 2020; Accepted: 28 February 2020;
Published: 07 April 2020.
Edited by:
Junlei Qi, Harbin Institute of Technology, ChinaReviewed by:
Kaibing Xu, Donghua University, ChinaXudong Liu, China Academy of Engineering Physics, China
Copyright © 2020 Li, Wei, Dong, Ou, Xiao, Yang, Xiao and Zhang. This is an open-access article distributed under the terms of the Creative Commons Attribution License (CC BY). The use, distribution or reproduction in other forums is permitted, provided the original author(s) and the copyright owner(s) are credited and that the original publication in this journal is cited, in accordance with accepted academic practice. No use, distribution or reproduction is permitted which does not comply with these terms.
*Correspondence: Peng Xiao, eGlhb3BlbmcmI3gwMDA0MDtjcXUuZWR1LmNu; Yunhuai Zhang, eHAyMDMxJiN4MDAwNDA7MTYzLmNvbQ==