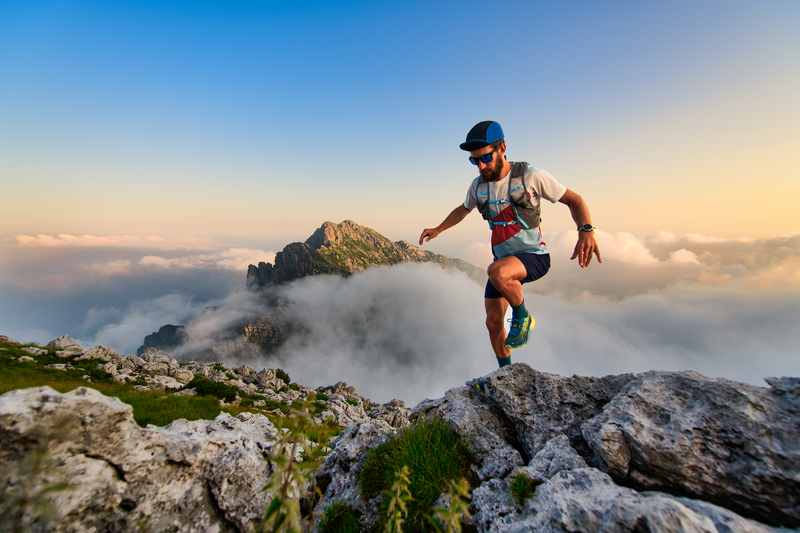
95% of researchers rate our articles as excellent or good
Learn more about the work of our research integrity team to safeguard the quality of each article we publish.
Find out more
MINI REVIEW article
Front. Chem. , 25 February 2020
Sec. Electrochemistry
Volume 8 - 2020 | https://doi.org/10.3389/fchem.2020.00125
This article is part of the Research Topic Deep Eutectic Solvents/Complex Salts-Based Electrolyte for Next Generation Rechargeable Batteries View all 10 articles
Although the successful deployment of lithium-ion batteries (LIBs) in various fields such as consumer electronics, electric vehicles and electric grid, the efforts are still ongoing to pursue the next-generation battery systems with higher energy densities. Interest has been increasing in the batteries relying on the multivalent-ions such as Mg2+, Zn2+, and Al3+, because of the higher volumetric energy densities than those of monovalent-ion batteries including LIBs and Na-ion batteries. Among them, magnesium batteries have attracted much attention due to the promising characteristics of Mg anode: a low redox potential (−2.356 V vs. SHE), a high volumetric energy density (3,833 mAh cm−3), atmospheric stability and the earth-abundance. However, the development of Mg batteries has progressed little since the first Mg-ion rechargeable battery was reported in 2000. A severe technological bottleneck concerns the organic electrolytes, which have limited compatibility with Mg anode and form an Mg-ion insulating passivation layer on the anode surface. Consequently, beneficial to the good chemical and mechanical stability, Mg-ion solid electrolyte should be a promising alternative to the liquid electrolyte. Herein, a mini review is presented to focus on the recent development of Mg-ion solid conductor. The performances and the limitations were also discussed in the review. We hope that the mini review could provide a quick grasp of the challenges in the area and inspire researchers to develop applicable solid electrolyte candidates for Mg batteries.
Since the commercialization proposed by Sony in 1990, Li-ion batteries (LIBs) have dominated in various fields, such as electronics, electric vehicles, and smart-grids, as the energy storage system (Goodenough and Kim, 2010; Etacheri et al., 2011; Devi et al., 2020). As the most successful battery technology nowadays, LIBs possess several advantages including high energy density, good capacity retention, no memory effect and low self-discharge, surpassing last generation of batteries i.e., lead-acid batteries, nickel metal hydride batteries (Li et al., 2020; Zeng et al., 2020). However, the growing demand for battery with even higher energy density is difficult to be satisfied by LIBs because their energy storage relies on the intercalation mechanism (Cabana et al., 2010; Choi and Aurbach, 2016; Hong et al., 2020). To further increase the energy density, great interest has been again aroused to develop lithium batteries directly using Li metal as the anode, which has remained quiescence from 1980s. The technical challenge of Li anode comes from the lithium dendrite formed on surface during cycling, which could penetrate the separator and result in the short-circuit of the battery and thus fire-catching or even explosion (Janek and Zeier, 2016; Chen et al., 2020). Despite the endeavors to suppress the dendrite formation, the progress is limited and none of the solutions meets the commercial standards (Cengiz et al., 2019; Michel et al., 2019).
Therefore, next generation advanced rechargeable batteries, such as multivalent metal (Mg, Ca, Al etc.) batteries, have aroused much interest due to high energy density in theory (Aurbach et al., 2000, 2007; Yoo et al., 2013; Wang et al., 2019). Among them, rechargeable Mg batteries are considered as the most promising battery technology because of the highest theoretical volumetric energy density of Mg anode (3,866 mAh cm−3), surpassing that of Li anode (2,066 mAh cm−3) (Aurbach et al., 2000; Bucur et al., 2015). While Li metal reacts violently with water, the reaction between Mg and water is much more stable because of the passive Mg hydroxide/oxide film formed on the surface. In addition, there is no dendrite formed for Mg anode during the reversible plating/stripping process. These advantages endow Mg anode high safety compared to Li anode (Aurbach et al., 2001). In addition, Mg is more abundant relative to Li in earth crust.
Several key challenges, however, should be overcome before the Mg battery technology comes true (Yoo et al., 2013; Saha et al., 2014; Bucur et al., 2015; Wang et al., 2020). For instance, due to the electrochemical reduction, a passivation layer is formed on Mg anode surface once Mg is on contact with conventional carbonate-based electrolyte solvents used in LIBs (Muldoon et al., 2012). While conducting for Li ion in LIBs, solid electrolyte interface (SEI) is insulating for Mg ion and thus prevents the conventional electrolyte solvents to be used in Mg batteries (Pan et al., 2020). Novel electrolytes are therefore developed for Mg batteries and most of them are based on Grignard reagents dissolved in ethereal solvents or glymes such as tetrahydrofuran (THF) (Deivanayagam et al., 2019). Nevertheless, concerns on safety and stability still remain for the high vapor pressure and the high flammability of ether-based organic solvents. Furthermore, the presence of Cl− anions in Grignard reagents results in the high corrosion and the electrolytes also have narrow electrochemical operation window (<2 V vs. Mg/Mg2+), indicating limited practical application (Tutusaus et al., 2015). Therefore, solid-state electrolyte employed by all-solid-state Mg batteries is a safe alternative in terms of heat and mechanical shock resistance (Ikeda et al., 1987; Imanaka et al., 1999; Janek and Zeier, 2016; Famprikis et al., 2019). Nevertheless, the development of solid-state Mg ion conductor with sufficient conductivity is a key challenge at ambient temperature because of the sluggish mobility resulted by the high charge density of Mg ion (Janek and Zeier, 2016; Famprikis et al., 2019). Many efforts are thus devoted to improve the mobility of Mg ion within the solid conductor and the target is 10−3-10−4 S cm−1 at ambient temperature, which is comparable with those of solid electrolytes used in lithium or sodium batteries (Janek and Zeier, 2016).
In this mini review, we will present a comprehensive development of solid Mg ion conductors, including phosphates, borohydrides, metal-organic frameworks (MOFs) and chalcogenides. It highlights the performance and the limitation of each material and also discusses the conduction mechanism on the basis of the crystal structure and the strategy to improve the ionic conductivity.
The study on solid-state ionic conductors have aroused much interest due to the merits of high stability and good safety. It is well-accepted that the migration of ion species is quite difficult in a solid, highly affected by the valence states of the ion species. Therefore, while there are many kinds of monovalent ionic conductors with high conductivity, few choices are available for multivalent ions such as Mg2+ (Janek and Zeier, 2016; Famprikis et al., 2019). Na+ superionic conductor (NASICON) is well-known for permitting the smooth migration of Na ion species due to the well-ordered three-dimensional network structure, and therefore it is highly interested to develop NASICON-type MZr4(PO4)6 (M = Ca2+, Sr2+, Mg2+, Ba2+) solids as multivalent ionic conductors (Lee et al., 2019; Shao et al., 2019).
The earliest report on NASICON-type Mg2+ conductor came from Ikeda in 1987, who studied the system of Mg-Zr-PO4 in various molar ratios as Mg ion conductor using mechanochemical synthesis (Ikeda et al., 1987). Among them, MgZr4(PO4)6 (MZP) showed the highest conductivity of 2.9 × 10−5 and 6.1 × 10−3 S cm−1 at 400 and 800°C, respectively. The Tubandt's method and the electron probe microanalysis were used to confirm the charge carrier to be Mg ions. According to X-ray diffraction (XRD) measurements, the author simply claimed that the crystal structure of MZP similar to NaZr2(PO4)3, resulted in the best conductivity relative to analogs with other ratios, while no further evidence was provided in the report.
Imanaka and Adachi continued the exploration on NASICON-type Mg2+ conductor. In 1999, they intentionally varied the starting materials of MZP to be non-stoichiometric ratios so as to produce the secondary phase of Zr2O(PO4)2 (Imanaka et al., 1999, 2000a). With the increase of the Zr2O(PO4)2 content in the composite, the Mg ion conductivity increased to a maximum value of 6.92 × 10−3 S cm−1 at 800°C for Mg1+xZr4P6O24+x+xZr2O(PO4)2 with x = 0.4. Too high content of Zr2O(PO4)2 however deteriorated the conductivity of the composite because of the insulating property of Zr2O(PO4)2. The presence of secondary phase was believed to enhance the relative density and thus the ionic conductivity by microscopically dispersing the Zr2O(PO4)2 secondary phase in the composite.
In 2001, Imanaka used the substitution strategy to improve the ionic conductivity of MZP by partially replacing Zr4+ with Nb5+ (Imanaka et al., 2000b). The substitution was used to statistically distribute mobile Mg ions to make a smooth Mg ion diffusion but it also reduced the number of migrating Mg2+ ion species in the solid solution electrolyte. Thus, the optimum conductivity was observed at x = 0.15 in Mg1−2x(Zr1−xNbx)4P6O24 with 7.7 × 10−4 S cm−1 at 600°C and 3.7 × 10−3 S cm−1 at 750°C, demonstrating little enhancement compared to pristine MZP.
Later in 2016, Imanaka and Tamura claimed that most of the attempts to obtain the NASICON-type structure of Mg ion conductor were unsuccessful, because of the small ionic radius of Mg2+ resulting in the formation of β-Fe2(SO4)3-type structure at high temperature (Tamura et al., 2016). They selected HfNb(PO4)3 as the mother solid and partially substituted Hf4+ with Mg2+ to realize Mg2+ conduction. Although the ion conductivity of (Mg0.1Hf0.9)4/3.8Nb(PO4)3 at high temperature was lower than that of Mg0.7(Zr0.85Nb0.15)4(PO4)6, the Mg2+ ion conductivity of the former at a moderate temperature of 300°C (2.1 × 10−6 S cm−1) was 20 times higher than that of latter (1.1 × 10−7 S cm−1). The authors proposed that the good ionic conductivity was resulted from the three-dimensionally well-ordered NASICON structure and also the presence of cations with a higher valence than that of the conducting cation Mg2+, enabling the smooth ion migration of the latter.
Adamu and Kale proposed a sol-gel method to synthesize MZP (Adamu and Kale, 2016). The MZP synthesized in this work showed an ionic conductivity of 7.23 × 10−3 S cm−1 at 725°C. They attributed improvement in the conductivity to the sol-gel preparation route, which ensured synthesis at the molecular level and avoid the impurity produced by the solid-state route due to the inhomogeneous mixing.
Liang and Laine used spray pyrolysis method to synthesize Mg0.5Ce0.2Zr1.8(PO4)3 nanopowders, where Ce4+ partially substituted Zr4+ in Mg0.5Zr2(PO4)3 (Liang et al., 2018). Mg0.5Ce0.2Zr1.8(PO4)3 offered highest conductivity up to 3 × 10−6 S cm−1 at 280°C, which was comparable with the previous report by Imanaka.
Inspired by the fabrication of amorphous solid conductor for LIBs, such as Li3PO4, Su and Tsuruoka proposed a plasma-assisted atomic layer deposition method to fabricate amorphous oxygen-deficient Mg2.4P2O5.4 thin film in 2019 (Cengiz et al., 2019; Su et al., 2019). It exhibited an ionic conductivity of 1.6 × 10−7 S cm−1 at 500°C. The hopping conduction of Mg ions in the disordered amorphous phosphate matrix was believed to result in the conductivity.
Despite the effort devoted to the development of phosphate-based Mg ion conductors, the progress is very limited and the ionic conductivity of Mg solid electrolyte based on MZP is never above 10−6 S cm−1 at moderate temperature of 300°C. The aim to function well at room temperature is still far for phosphate-based ionic conductors. It seems that phosphate-based materials are not ideal candidates of solid Mg ion conductors and it is necessary to turn to novel Mg ion conductors.
Since Mohtadi demonstrated the first fully inorganic and halide-free Mg electrolytes, enabling reversible Mg plating and stripping, in 2012, liquid electrolytes based on magnesium borohydride, Mg(BH4)2, have received significant attention (Mohtadi et al., 2012). Because of the reductive stability of the anion, Mg(BH4)2 also arouses the interest to develop as solid Mg ion conductor. However, the conductivity of Mg(BH4)2 is very low at room temperature (10−12 S cm−1 at 30°C), resulted by the firm tetrahedral sites of hindering the smooth migration of Mg ions. Proper modification of Mg(BH4)2 are thus necessary to improve the conductivity.
In 2014, Higashi first reported Mg(BH4)(NH2) as a new class of solid-state Mg ion conductor (Higashi et al., 2014). The diffusion of Mg ions in Mg(BH4)(NH2) was attributed to the Mg zigzag chain and tunneling structures in the a and b planes. It showed the ionic conductivity of 10−6 S cm−1 at 150°C and the electrochemical window of ~3 V in estimation.
Le Ruyet and Janot followed the work on Mg(BH4)(NH2) in 2019, and studied the influences of the synthesis parameters on the ionic conductivity (Le Ruyet et al., 2019). They carefully investigated the synthesis parameters and the ionic conductivity could reach as high as 3 × 10−6 S cm−1 at 100°C, which was three orders of magnitude higher than that reported by Higashi. The improvement in conductivity was attributed to the creation of a glass-ceramic-like composite due to the presence of an amorphous additional phase.
Roedern and Remhof synthesized a Mg(BH4)2 derivative by coordinating Mg2+ with a neutral bidentate ethylenediamine ligand to replace two BH4 ligand (Roedern et al., 2017). It showed a high ionic conductivity of 5 × 10−8 S cm−1 at 30°C and 6 × 10−5 S cm−1 at 70°C. The good conductivity was attributed to the partially chelated, mixed coordination of Mg2+ leading to its high mobility. However, the electrochemical stability of this new phase is limited to 1.2 V vs. Mg/Mg2+, due to the stability limitation by the ethylenediamine ligand.
Compared with phosphate-based Mg ion conductors, Mg(BH4)2 and its derives show a promising future as Mg ion conductors with high ionic conductivity at low temperature. Proper modification of Mg(BH4)2 are still necessary to improve the conductivity, stability and operating potential window.
Chalcogenide-based materials have been developed as solid conductors for Li ion and Na ion and have shown high ionic conductivity (Ramos et al., 2018; Xuan et al., 2018; Jia et al., 2019; Wang Y. et al., 2019). It is therefore highly interesting to develop chalcogenide-based ionic conductors for Mg ion.
In 2014, Yamanaka and Tatsumisago prepared the MgS–P2S5-MgI2 glasses and glass-ceramics by a mechanochemical method (Yamanaka et al., 2014). The addition of MgI2 content in 60MgS·40P2S5 helped the formation of glass-ceramic and the conductivity monotonically increased with the increase of MgI2 content, showing the highest ionic conductivity to be 2.1 × 10−7 S cm−1 at 200°C. The authors proposed that the Mg2P2S6 crystal phase contributed to the increased conductivities. But no solid evidence was presented to support the claim in the study.
In 2017, Canepa, Bo and Ceder demonstrated the discovery of spinel chalcogenides MgX2Z4 [X = (In, Y, Sc) and Z = (S, Se)] as a class of fast Mg ion solid conductors with the combination of theoretical and experimental studies (Canepa et al., 2017). The ambient-temperature ionic conductivity could reach as high as ~10−4 S cm−1 for MgSc2Se4 at 25°C. The fast diffusion of Mg ions was achieved by the occupation of stable Mg2+ site in its unfavorable tetrahedral coordination environment within spinel structure (Figures 1A,B). The measured Mg migration barrier was consistent with the computed data (Figure 1C). Such rationale could be used as a general design rule for multivalent-ion solid conductors. However, the electronic conductivity of MgSc2Se4 is ~0.04% of the ionic conductivity, which is substantially larger than other state-of-the-art alkali solid-state electrolytes (σe/σi~10−4-10−6%) and hinders MgSc2Se4 as an applicable solid-state conductor.
Figure 1. Tet-oct-tet migration path in the AX2Z4 framework, with energy of the tet, oct, and transition sites indicated by Etet, Eoct, Ea, respectively (Ea corresponds to the migration energy) (A); effect of the anion size on the shared (triangular) face between tet and oct sites (B) and 25Mg static variable temperature spin lattice relaxation data collected at 7.02 T as function of temperature and Arrhenius fit (C). Reproduced from Canepa et al. (2017), licensed under CC BY 4.0. structures of the metal–organic frameworks Mg2(dobdc) (1) and Mg2(dobpdc) (2), as viewed along the c-axis (D); a close-up of the open coordination sites at the vertices of the pore that interact with nucleophilic guest species: PhO− = phenolate, MePhO− = 4-methylphenolate, CF3PhO− = 4-trifluoromethylphenolate, and TFSI−= bis(trifluoromethanesulfonyl)imide (E). Reproduced with permission from Aubrey et al. (2014), Copyright 2014, Royal Society of Chemistry.
In order to make MgSc2Se4 as an applicable solid-state conductor, Wang and Fichtner tried two strategies to reduce the electronic conductivity of MgSc2Se4, including synthesizing Se-rich phase and doping with Ce4+ or ti4+ to partially substitute Sc3+ (Wang et al., 2019). However, neither methods could successfully reduce the electronic conductivity. The authors suggested to employ MgSc2Se4 as a surface coating to protect Mg anode or cathode materials.
A significant improvement in ionic conductivity is achieved by chalcogenide-based material, which is 10−4 S cm−1 for MgSc2Se4 at ambient temperature. Despite the promising conductivity, its high electronic conductivity hinders its application as a suitable solid conductor. Further modifications are necessary to decrease the electronic conductivity and increase the ionic conductivity at the same time.
Metal–organic frameworks (MOFs) are crystalline solids composed of metal ions coordinated by multifunctional organic molecules with a three-dimensional porous structure. The composition and structure of MOFs could be easily adjusted via the rational selection of the metal ion and organic molecule (Rouhani et al., 2019). Furthermore, they feature poor electrical conductivity and well-defined porous structure, allowing for fast ion diffusion (Zhu et al., 2019). Therefore, MOFs could be promising candidates as ideal ionic conductors for selective transport. Many MOFs have been reported as solid Li+ conductors, with the conductivities as high as 3 × 10−4 S cm−1 at room temperature. In contrast, the work on solid Mg conductors is still few in the field.
In 2014, Aubrey and Long first presented porous MOFs of Mg2(dobdc) (dobdc4− = 2,5-dioxidobenzene-1,4-dicarboxylate) and its analog Mg2(dobpdc) (dobpdc4−=4,4′-dioxidobiphenyl-3,3′-dicarboxylate) as solid Mg2+ conductors (Aubrey et al., 2014). These MOFs exhibited ionic conductivities of up to 2.5 × 10−4 S cm−1 at room temperature after soaked in solution containing Mg salt, comparable with polymer gels. As can be seen in Figure 1D, Mg2(dobdc) shows the pore size of 13 Å and Mg2(dobpdc) has the pore size up to 21 Å, suggesting their good ability to accommodate the ion species like Mg2+ in high charge density. With the insertion of Mg salts, a high density of open metal sites in MOFs could capture nucleophilic anions and thus allow for the favorably free mobility of Mg ions within pores (Figure 1E). Therefore, high ionic conductivity was achieved for Mg2(dobpdc) impregnated with magnesium phenolates.
In 2017, Park and Dinca proposed a Cu(II)–azolate MOF (MIT-20) as a tunable solid electrolyte for Li+, Na+ and Mg2+ after soaking in the corresponding halide or pseudohalide salts (Park et al., 2017). Its Mg2+-substituted analog, MIT-20-MgBr2, exhibited the Mg ionic conductivity of 8.8 × 10−7 S cm−1 at the room temperature. MIT-20 was attractive for its feature of immobilizing anions by the Cu(II) metal center, allowing the favorably free migration of cations within the one-dimensional pores. Later in 2019, Miner from Dinca's group continued their work on the tunable solid electrolyte of MOF (Miner et al., 2019). The MOF was Cu4(ttpm)2·0.6CuCl2, possessing high surface area with plenty of Cu(II) cations to bound halide anions. Its analog, Cu4(ttpm)2·0.6CuCl2-MgBr2, exhibited an ionic conductivity of 1.3 × 10−4 S cm−1 for Mg ions, demonstrating the promising future of MOF-based solid electrolyte to optimize the ionic conductivity via the control of identity, geometry and distribution of the cation hopping sites.
Instead of pressed pellets, Luo, Tsung and Wang synthesized a Mg-MOF-74 thin film as the Mg ion conductor to eliminate interparticle gaps that were inevitable for pressed pellets and enabled studies on the inherent ionic conductivity of MOFs (Luo et al., 2019). It showed the ionic conductivity of 3.17 × 10−6 S cm−1 at the room temperature, which was in consistent with the previous report by Aubrey.
Despite the promising properties, additional salt contents in excess are required for MOFs solid conductors based on the number of available anion binding sites. Furthermore, activation energies are sometimes higher that what are expected, due to the strong pairing between cations and anions of the salts. Electrochemical stability upon cycling is another challenge for some reported MOFs-based conductors.
Mg battery is considered as a promising next-generation advanced battery technology possessing high theoretical volumetric energy density, good safety and high abundance. Nevertheless, before the battery technology becomes viable, several critical technological challenges should be conquered, including the development of proper electrolyte and cathodes. The SEI formed on the Mg surface is ionic-blocking for Mg ion when Mg batteries employs the conventional carbonate-based solvent commonly used in LIBs. The electrolyte development for Mg batteries therefore cannot simply mimic LIB electrolytes but requires novel design strategies. The proposal of ether-based solvent demonstrated the ability to avoid the formation of insulating passivation layer, but the practical application is still highly limited by the corrosive issues from Grignard salts and the safety concern, as well as the small electrochemical potential window. Featuring high stability and safety, ionic solid conductor could be an ideal solution to the above problems. But Mg ions have sluggish mobility within solid conductor due to the high charge density. Several types of solid conductors, including phosphates, borohydrides, chalcogenides and MOFs, have been developed to improve the performance of Mg ionic solid conductor over the recent years (Figure 2A).
Figure 2. A timeline of key development of Mg ion solid conductors (A) and a summary of the ionic conductivities of various solid conductors as a function of temperature in the report (B).
As summarized in Figure 2B, the impressive progress has been achieved with the conductivity of initially 10−3 S cm−1 at 800°C improved to be 10−4 S cm−1 at ambient temperature. Despite the efforts, phosphates are still far from to meet the requirement of high ionic conductivity at low temperature and show poor progress over decades, demonstrating little promise as Mg solid conductors. In contrast, significant improvements in conductivity have been shown by the recent development of borohydrides, chalcogenides and MOFs. These novel solid conductors should be the development emphasis in next stage.
However, more work should be done before the ionic conductors are sufficiently conductive for practical consideration in Mg batteries. The electrochemical potential window should be increased to a sufficiently high level. The feasibility of the solid conductor requires the practical evaluation in combination with Mg anode and cathodes as an all-solid-state Mg battery. Furthermore, theoretical calculation could be a powerful tool to improve the performance of solid conductor.
YZ was in charge of organization and writing of the manuscript. WZ contributed the Phosphate section. BL contributed the Borohydride section. HL contributed the Chalcogenide section. WL contributed the MOFs section.
This work was conducted by Hundred Talents Project that was supported by Sun Yat-Sen University, China.
The authors declare that the research was conducted in the absence of any commercial or financial relationships that could be construed as a potential conflict of interest.
Adamu, M., and Kale, G. M. (2016). Novel sol-gel synthesis of MgZr4P6O24 composite solid electrolyte and newer insight into the Mg2+-ion conducting properties using impedance spectroscopy. J. Phys. Chem. C 120, 17909–17915. doi: 10.1021/acs.jpcc.6b05036
Aubrey, M. L., Ameloot, R., Wiers, B. M., and Long, J. R. (2014). Metal-organic frameworks as solid magnesium electrolytes. Energy Environ. Sci. 7, 667–671. doi: 10.1039/c3ee43143f
Aurbach, D., Gofer, Y., Lu, Z., Schechter, A., Chusid, O., Gizbar, H., et al. (2001). A short review on the comparison between Li battery systems and rechargeable magnesium battery technology. J. Power Sources 97–8, 28–32. doi: 10.1016/S0378-7753(01)00585-7
Aurbach, D., Lu, Z., Schechter, A., Gofer, Y., Gizbar, H., Turgeman, R., et al. (2000). Prototype systems for rechargeable magnesium batteries. Nature 407, 724–727. doi: 10.1038/35037553
Aurbach, D., Suresh, G. S., Levi, E., Mitelman, A., Mizrahi, O., Chusid, O., et al. (2007). Progress in rechargeable magnesium battery technology. Adv. Mater. 19, 4260–4267. doi: 10.1002/adma.200701495
Bucur, C. B., Gregory, T., Oliver, A. G., and Muldoon, J. (2015). Confession of a Magnesium Battery. J. Phys. Chem. Lett. 6, 3578–3591. doi: 10.1021/acs.jpclett.5b01219
Cabana, J., Monconduit, L., Larcher, D., and Rosa Palacin, M. (2010). Beyond intercalation-based Li-ion batteries: the state of the Art and challenges of electrode materials reacting through conversion reactions. Adv. Mater. 22, E170–E192. doi: 10.1002/adma.201000717
Canepa, P., S.-,Bo, H., Gautam, G. S., Key, B., Richards, W. D., Shi, T., et al. (2017). High magnesium mobility in ternary spinel chalcogenides. Nat. Commun. 8:1759. doi: 10.1038/s41467-017-01772-1
Cengiz, M., Oh, H., and Lee, S. H. (2019). Lithium dendrite growth suppression and ionic conductivity of Li2S-P2S5-P2O5 glass solid electrolytes prepared by mechanical milling. J. Electrochem. Soc. 166, A3997–A4004. doi: 10.1149/2.0311916jes
Chen, L., Huang, Z., Pang, W., Jin, Z., Li, Y., and Wang, C.-A. (2020) Dual interface layers for solid-state Li metal battery with low interfacial resistance small polarization based on garnet electrolyte. Electrochim. Acta 330:135352. doi: 10.1016/j.electacta.2019.135352
Choi, J. W., and Aurbach, D. (2016). Promise and reality of post-lithium-ion batteries with high energy densities. Nat. Rev. Mater. 1:16013. doi: 10.1038/natrevmats.2016.13
Deivanayagam, R., Ingram, B. J., and Shahbazian-Yassar, R. (2019). Progress in development of electrolytes for magnesium batteries. Energy Storage Mater. 21, 136–153. doi: 10.1016/j.ensm.2019.05.028
Devi, M. M., Ankush Guchhait, S. K., Sunaina Suresh Babu, G. N, Sreekanth, M, et al. (2020) Energy efficient electrodes for lithium-ion batteries: recovered processed from spent primary batteries. J. Hazard. Mater. 384:121112. doi: 10.1016/j.jhazmat.2019.121112.
Etacheri, V., Marom, R., Elazari, R., Salitra, G., and Aurbach, D. (2011). Challenges in the development of advanced Li-ion batteries: a review. Energy Environ. Sci. 4, 3243–3262. doi: 10.1039/c1ee01598b
Famprikis, T., Canepa, P., Dawson, J. A., Islam, M. S., and Masquelier, C. (2019). Fundamentals of inorganic solid-state electrolytes for batteries. Nat. Mater. 18, 1278–1291. doi: 10.1038/s41563-019-0431-3
Goodenough, J. B., and Kim, Y. (2010). Challenges for rechargeable Li batteries. Chem. Mater. 22, 587–603. doi: 10.1021/cm901452z
Higashi, S., Miwa, K., Aoki, M., and Takechi, K. (2014). A novel inorganic solid state ion conductor for rechargeable Mg batteries. Chem. Commun. 50, 1320–1322. doi: 10.1039/c3cc47097k
Hong, X., Wang, R., Liu, Y., Fu, J., Liang, J., and Dou, S. (2020) Recent advances in chemical adsorption catalytic conversion materials for Li-S batteries. J. Energy Chem. 42, 144–168. doi: 10.1016/j.jechem.2019.07.001
Ikeda, S., Takahashi, M., Ishikawa, J., and Ito, K. (1987). Solid electrolytes with multivalent cation conduction. I. Conducting species in Mg-Zr-PO4 system. Solid State Ion 23, 125–129. doi: 10.1016/0167-2738(87)90091-9
Imanaka, N., Okazaki, Y., and Adachi, G. (2000a). Divalent magnesium ion conducting characteristics in phosphate based solid electrolyte composites. J. Mater. Chem. 10, 1431–1435. doi: 10.1039/a909599c
Imanaka, N., Okazaki, Y., and Adachi, G. (2000b). Divalent magnesium ionic conduction in Mg1-2x(Zr1-xNbx)(4)P6O24 (x=0-0.4) solid solutions. Electrochem. Solid State Lett. 3, 327–329. doi: 10.1149/1.1391138
Imanaka, N., Okazaki, Y., and Adachi, G. Y. (1999). Bivalent magnesium ionic conduction in the magnesium phosphate based composites. Chem. Lett. 28, 939–940. doi: 10.1246/cl.1999.939
Janek, J., and Zeier, W. G. (2016). A solid future for battery development. Nat. Energy 1:16141. doi: 10.1038/nenergy.2016.141
Jia, H., Sun, Y., Zhang, Z., Peng, L., An, T., and Xie, J. (2019). Group 14 element based sodium chalcogenide Na4Sn0.67Si0.33S4 as structure template for exploring sodium superionic conductors. Energy Stor. Mater. 23, 508–513. doi: 10.1016/j.ensm.2019.04.011
Le Ruyet, R., Berthelot, R., Salager, E., Florian, P., Fleutot, B., and Janot, R. (2019). Investigation of Mg(BH4)(NH2)-based composite materials with enhanced Mg2+ ionic conductivity. J. Phys. Chem. C 123, 10756–10763. doi: 10.1021/acs.jpcc.9b00616
Lee, W., Tamura, S., and Imanaka, N. (2019). Synthesis and characterization of divalent ion conductors with NASICON-type structures. J. Asian Ceramic Soc. 7, 221–227. doi: 10.1080/21870764.2019.1606141
Li, Q., Chen, D., Tan, H., Zhang, X., Rui, X., and Yu, Y. (2020) 3D porous V2O5 architectures for high-rate lithium storage. J. Energy Chem. 40, 15–21. doi: 10.1016/j.jechem.2019.02.010
Liang, B., Keshishian, V., Liu, S., Yi, E., Jia, D., Zhou, Y., et al. (2018). Processing liquid-feed flame spray pyrolysis synthesized Mg0.5Ce0.2Zr1.8(PO4)(3) nanopowders to free standing thin films and pellets as potential electrolytes in all-solid-state Mg batteries. Electrochimica Acta 272, 144–153. doi: 10.1016/j.electacta.2018.04.015
Luo, J. R., Li, Y., Zhang, H. C., Wang, A. L., Lo, W. S., Dong, Q., et al. (2019). A metal-organic framework thin film for selective Mg2+ transport. Angew. Chem. Int. Ed. 58, 15313–15317. doi: 10.1002/anie.201908706
Michel, F., Becker, M., Janek, J., and Polity, A. (2019). Investigations of the solid electrolyte interphase using X-ray photoelectron spectroscopy in situ experiment on the lithium-based solid electrolyte LiPSON. Phys. Status Solidi B-Basic Solid State Phys. doi: 10.1002/pssb.201900336. [Epub ahead of print].
Miner, E. M., Park, S. S., and Dinca, M. (2019). High Li+ and Mg2+ conductivity in a Cu-azolate metal-organic framework. J. Am. Chem. Soc. 141, 4422–4427. doi: 10.1021/jacs.8b13418
Mohtadi, R., Matsui, M., Arthur, T. S., and Hwang, S.-J. (2012). Magnesium borohydride: from hydrogen storage to magnesium battery. Angew. Chem. Int. Ed. 51, 9780–9783. doi: 10.1002/anie.201204913
Muldoon, J., Bucur, C. B., Oliver, A. G., Sugimoto, T., Matsui, M., Kim, H. S., et al. (2012). Electrolyte roadblocks to a magnesium rechargeable battery. Energy Environ. Sci. 5, 5941–5950. doi: 10.1039/c2ee03029b
Pan, W., Guan, W., and Jiang, Y. (2020). Research advances in polyanion-type cathodes for sodium-ion batteries. Acta Phys. Chim. Sin. 36:1600275. doi: 10.1002/advs.201600275
Park, S. S., Tulchinsky, Y., and Dinca, M. (2017). Single-Ion Li+, Na+, and Mg2+ solid electrolytes supported by a mesoporous anionic cu-azolate metal-organic framework. J. Am. Chem. Soc. 139, 13260–13263. doi: 10.1021/jacs.7b06197
Ramos, E. P., Zhang, Z., Assoud, A., Kaup, K., Lalere, F., and Nazar, L. F. (2018). Correlating ion mobility and single crystal structure in sodium-ion chalcogenide-based solid state fast ion conductors: Na(11)Sn(2)PnS(12) (Pn = Sb, P). Chem. Mater. 30, 7413–7417. doi: 10.1021/acs.chemmater.8b02077
Roedern, E., R.-,Kuehnel, S., Remhof, A., and Battaglia, C. (2017). Magnesium ethylenediamine borohydride as solid-state electrolyte for magnesium batteries. Sci. Rep. 7:46189. doi: 10.1038/srep46189
Rouhani, F., Rafizadeh-Masuleh, F., and Morsali, A. (2019). Highly electroconductive metal-organic framework: tunable by metal ion sorption quantity. J. Am. Chem. Soc. 141, 11173–11182. doi: 10.1021/jacs.9b04173
Saha, P., Datta, M. K., Velikokhatnyi, O. I., Manivannan, A., Alman, D., and Kumta, P. N. (2014). Rechargeable magnesium battery: current status and key challenges for the future. Prog. Mater. Sci. 66, 1–86. doi: 10.1016/j.pmatsci.2014.04.001
Shao, Y. J., Zhong, G. M., Lu, Y. X., Liu, L. L., Zhao, C. L., Zhang, Q. Q., et al. (2019). A novel NASICON-based glass-ceramic composite electrolyte with enhanced Na-ion conductivity. Energy Stor. Mater. 23, 514–521. doi: 10.1016/j.ensm.2019.04.009
Su, J., Tsuruoka, T., Tsujita, T., Nishitani, Y., Nakura, K., and Terabe, K. (2019). Atomic layer deposition of a magnesium phosphate solid electrolyte. Chem. Mater. 31, 5566–5575. doi: 10.1021/acs.chemmater.9b01299
Tamura, S., Yamane, M., Hoshino, Y., and Imanaka, N. (2016). Highly conducting divalent Mg2+ cation solid electrolytes with well-ordered three-dimensional network structure. J. Solid State Chem. 235, 7–11. doi: 10.1016/j.jssc.2015.12.008
Tutusaus, O., Mohtadi, R., Arthur, T. S., Mizuno, F., Nelson, E. G., and Sevryugina, Y. V. (2015). An efficient halogen-free electrolyte for use in rechargeable magnesium batteries. Angew. Chem. Int. Ed. 54, 7900–7904. doi: 10.1002/anie.201412202
Wang, C., Zeng, Y., Xiao, X., Wu, S., Zhong, G., Xu, K, et al. (2020) gamma-MnO2 nanorods/graphene composite as efficient cathode for advanced rechargeable aqueous zinc-ion battery. J. Energy Chem. 43, 182–187. doi: 10.1016/j.jechem.2019.08.011.
Wang, L.-P., Zhao-Karger, Z., Klein, F., Chable, J., Braun, T., Schuer, A. R., et al. (2019). MgSc(2)Se(4)A magnesium solid ionic conductor for all-solid-state Mg batteries? ChemSusChem 12, 2286–2293. doi: 10.1002/cssc.201900225
Wang, Y., Lu, X., Zheng, C., Liu, X., Chen, Z., Yang, W., et al. (2019). Chemistry design towards a stable sulfide-based superionic conductor Li4Cu8Ge3S12. Angew. Chem. Int. Ed. 58, 7673–7677. doi: 10.1002/anie.201901739
Xuan, M., Xiao, W., Xu, H., Shen, Y., Li, Z., Zhang, S., et al. (2018). Ultrafast solid-state lithium ion conductor through alloying induced lattice softening of Li6PS5Cl. Journal of Materials Chemistry A 6, 19231–19240. doi: 10.1039/C8TA07240J
Yamanaka, T., Hayashi, A., Yamauchi, A., and Tatsuinisago, M. (2014). Preparation of magnesium ion conducting MgS-P2S5-MgI2 glasses by a mechanochemical technique. Solid State Ionics 262, 601–603. doi: 10.1016/j.ssi.2013.10.037
Yoo, H. D., Shterenberg, I., Gofer, Y., Gershinsky, G., Pour, N., and Aurbach, D. (2013). Mg rechargeable batteries: an on-going challenge. Energy Environ. Sci. 6, 2265–2279. doi: 10.1039/c3ee40871j
Zeng, J., Peng, C., Wang, R., Cao, C., Wang, X., and Liu, J. (2020) Micro-sized FeS2@FeSO4 core-shell composite for advanced lithium storage. J. Alloys Compounds 814:151922. doi: 10.1016/j.jallcom.2019.151922
Keywords: Mg batteries, solid electrolyte, phosphate, borohydride, chalcogenides, metal-organic frame (MOFs)
Citation: Zhan Y, Zhang W, Lei B, Liu H and Li W (2020) Recent Development of Mg Ion Solid Electrolyte. Front. Chem. 8:125. doi: 10.3389/fchem.2020.00125
Received: 06 January 2020; Accepted: 11 February 2020;
Published: 25 February 2020.
Edited by:
Du Yuan, Nanyang Technological University, SingaporeReviewed by:
Jin Zhao, Nanjing University of Posts and Telecommunications, ChinaCopyright © 2020 Zhan, Zhang, Lei, Liu and Li. This is an open-access article distributed under the terms of the Creative Commons Attribution License (CC BY). The use, distribution or reproduction in other forums is permitted, provided the original author(s) and the copyright owner(s) are credited and that the original publication in this journal is cited, in accordance with accepted academic practice. No use, distribution or reproduction is permitted which does not comply with these terms.
*Correspondence: Yi Zhan, emhhbnk5QG1haWwuc3lzdS5lZHUuY24=
Disclaimer: All claims expressed in this article are solely those of the authors and do not necessarily represent those of their affiliated organizations, or those of the publisher, the editors and the reviewers. Any product that may be evaluated in this article or claim that may be made by its manufacturer is not guaranteed or endorsed by the publisher.
Research integrity at Frontiers
Learn more about the work of our research integrity team to safeguard the quality of each article we publish.