- 1Department of Chemistry, University of Bath, Bath, United Kingdom
- 2School of Physical and Mathematical Sciences, Nanyang Technological University, Singapore, Singapore
- 3Materials and Chemical Characterization (MC2), University of Bath, Bath, United Kingdom
- 4Department of Chemical Engineering and Water Innovation & Research Centre, University of Bath, Bath, United Kingdom
- 5Centre for Sustainable Chemical Technologies, University of Bath, Bath, United Kingdom
Colorimetry is an advantageous method for detecting fluoride in drinking water in a resource-limited context, e. g., in parts of the developing world where excess fluoride intake leads to harmful health effects. Here we report a selective colorimetric chemosensor for fluoride that employs an azulene as the reporter motif and a pinacolborane as the receptor motif. The chemosensor, NAz-6-Bpin, is prepared using the Nozoe azulene synthesis, which allows for its rapid and low-cost synthesis. The chemosensor gives a visually observable response to fluoride both in pure organic solvent and also in water/alcohol binary solvent mixtures.
Introduction
Fluoride is present in naturally abundant minerals in the Earth's crust, and is therefore present in groundwater throughout the world (Ozsvath, 2009). There is only a narrow range between levels of fluoride intake that are beneficial and detrimental to human health. Whilst use of fluoridated toothpaste can help the prevention of tooth decay (Selwitz et al., 2007), exposure to greater quantities of fluoride can lead to both dental and skeletal fluorosis (DenBesten and Li, 2011; Ghosh et al., 2013). The World Health Organization (WHO) guidelines suggest that drinking water should not exceed fluoride concentrations of 1.5 mg L−1 (World Health Organization, 2011). For most of the world, fresh-water fluoride content is below 0.5 mg L−1 (World Health Organization, 1994). However, it is estimated that for over 200 million people their main source of drinking water exceeds the WHO acceptable limit of fluoride (Amini et al., 2008). Dental fluorosis is prevalent amongst the populations within areas where fluoride content of water is greater than the WHO recommended limit (Petersen et al., 2005). High ground-water fluoride content and dental fluorosis is particularly prevalent in Brazil, China, India, and throughout Africa (Fawell et al., 2006).
Whilst a range of approaches have been developed to remove fluoride from drinking water (Jagtap et al., 2012), it is necessary to have methods for detecting fluoride concentrations above the WHO safe limit, in order to know when to deploy fluoride remediation techniques. Accordingly, the development of molecular sensors for fluoride has seen significant research activity (Cametti and Rissanen, 2009; Zhou et al., 2014). Methods for chemical detection of fluoride anion may be subdivided into use of chemosensors (in which fluoride is reversibly bound to a receptor motif) and chemodosimeters (in which fluoride mediates an irreversible chemical reaction of the probe molecule). Strategies for chemodosimeter design include formation of an Si-F bond, usually inducing cleavage of an Si-O or Si-C bond (For a review, see: Chen et al., 2019. See also: Yamaguchi et al., 2000; Descalzo et al., 2002; Kim and Swager, 2003; Zhu et al., 2005; Bozdemir et al., 2010; Hu et al., 2010; Lu et al., 2011; Baker and Phillips, 2012; Li et al., 2014; Turan and Akkaya, 2014; Zou et al., 2014; Chavali et al., 2015; Mahapatra et al., 2015; Gabrielli and Mancin, 2016; Chansaenpak et al., 2018). Strategies for chemosensor design include coordination of fluoride to trivalent boron (For a review, see: Wade et al., 2010. See also: Yamaguchi et al., 2001; Sole and Gabbaï, 2004; Kim and Gabbaï, 2009; Nishimura et al., 2013; Mellerup et al., 2016; Tao et al., 2019), coordination to lanthanide complexes (Liu et al., 2014; Butler, 2015; Singhal and Jha, 2019), and fluoride-induced proton transfer and/or conformational change (Black et al., 1999; Gunnlaugsson et al., 2001, 2004, 2005; Sessler et al., 2002; Peng et al., 2005; Salman et al., 2005; Mahapatra et al., 2015).
Molecular probes for fluoride may have reporter motifs that give rise to a fluorescence, chemiluminescence, electrochemical or colorimetric response, for example. Of these, colorimetric probes for fluoride have significant advantages in the context of fluoride detection in drinking water in developing nations. They have the potential to be easy to transport and mass produce, and tests can be performed by non-expert users, without any requirement for a laboratory environment, expensive equipment or a power supply. Indeed, observing a color change does not require the user to be literate. However, such colorimetric probes are not without disadvantages. Fluoride has proven difficult to detect in water, primarily due to the extensive solvent cluster of water around fluoride (Cabarcos et al., 1999; Zhan and Dixon, 2004), with which a sensor would have to compete. In such instances, surfactants such as cetyltrimethylammonium bromide (CTAB) have sometimes been employed to dissolve the sensor in a micellular environment (Hu et al., 2010; Calderon-Ortiz et al., 2012; Elsayed et al., 2013; Roy et al., 2015; Wang et al., 2015; Qiu et al., 2016; Wallabregue et al., 2016). Phase-transfer catalysts such as tetra-n-butylammonium hydrogensulfate (TBAS) can be used in tandem to aid transport of the analyte into the micelle (Lopez-Alled et al., 2017).
Azulene, an isomer of naphthalene, consists of fused 7- and 5-membered ring systems. It is both unusually polar and colorful (blue) for an aromatic hydrocarbon (Michl and Thulstrup, 1976). The color of azulene can be tuned in a predictable fashion by altering the substituents at different positions on the azulene core (Liu and Asato, 2003). This fact has been exploited for its use in a range of colorimetric sensors. Examples include sensors for silver (Wakabayashi et al., 2013), nitrite (Murfin et al., 2020), mercury (Wakabayashi et al., 2007, 2008, 2012; Razus et al., 2011; Birzan et al., 2017; Buica et al., 2018, 2019), phosphate (Lichosyt et al., 2016, 2018), and reactive oxygen species (Murfin et al., 2019).
We have previously described the colorimetric azulene-based fluoride sensor Az-1-Bpin which exhibited excellent selectivity toward fluoride in THF (Lopez-Alled et al., 2017), with a notable color change from purple to yellow (Figure 1). The sensor did not respond to fluoride in any water/organic mixed solvent system. However, a response of Az-1-Bpin to fluoride in water was achieved in the presence of surfactants CTAB and TBAS, for which a color change from purple to purple-blue was observed. Subsequent to our work, further reports on boron-containing azulenes that respond colorimetrically to fluoride anion have been published, describing a variant of Az-1-Bpin for which a dimeric structure was claimed (Fang et al., 2018), and describing annulated borazaazulenes (Xin et al., 2020).
Results and Discussion
The synthesis of Az-1-Bpin employs an iridium-catalyzed C-H borylation reaction (Kurotobi et al., 2003), wherein the catalyst comprises an expensive and depleting platinum group metal. Although an alternative synthesis was later reported (Bagutski et al., 2013), both syntheses require azulene itself as a starting material, which is also costly. In order to produce an azulene-based fluoride chemodosimeter that did not require azulene as a starting material, we instead considered the classical Nozoe azulene synthesis. Azulenes bearing ester substituents at the 1- and 3-positions can be readily accessed using this methodology, which employs tropolone as the starting material (Nozoe et al., 1962). We opted to employ the proven pinacolboron group as the receptor motif, but appended to the seven-membered ring of azulene (as opposed to the five-membered ring as in Az-1-Bpin). We synthesized and assessed several variants based on this design strategy, and it was found that diethyl 2-chloro-6-(4,4,5,5-tetramethyl-1,3,2-dioxaborolan-2-yl)azulene-1,3-dicarboxylate (which we have termed “NAz-6-Bpin”) gave the most substantial color change in response to fluoride.
Similarly to the case of Az-1-Bpin, our expectation was that upon fluoride binding to NAz-6-Bpin, the conjugation between the azulene ring and vacant p-orbital on the sp2-hybridized boron atom would be abolished since the boron would necessarily adopt sp3 hybridization. This in turn would significantly perturb the π-system and resulting in the hoped-for colorimetric response (Figure 2).
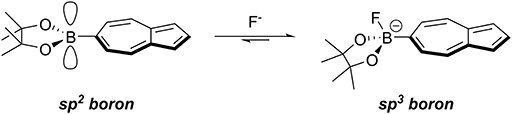
Figure 2. Binding of fluoride to the vacant p-orbital on the boronic ester results in rehybridization from sp2 to sp3.
NAz-6-Bpin has previously been synthesized (for a purpose other than chemical sensing) through use of isoamyl nitrite and HCl gas (Xin et al., 2016). We found we could avoid the use of HCl gas by adopting a more recent procedure from the same group, using Me3SiCl in its place (Xin et al., 2018). Using this method, NAz-6-Bpin was synthesized from precursor 1 in a yield of 96 % (Figure 3).
To assess the suitability of NAz-6-Bpin to detect fluoride, initial studies were performed in THF. We compared the selectivity of NAz-6-Bpin toward fluoride over other halide anions. As the experiments were performed in organic solvent, halides were added as their tetra-n-butyl ammonium salts (TBAX, where X = F, Cl, Br, I). An instant color change from pink to yellow was observed with TBAF only (Figure 4), and the UV-vis absorbance spectra were collected in each case (Figure S1). The absorption maximum underwent a hypsochromic shift from λmax = 523 nm (NAz-6-Bpin), to λmax = 464 nm (NAz-6-Bpin + F−). The 11B NMR spectra of NAz-6-Bpin and NAz-6-Bpin + TBAF in THF show two resonances, at δ = 28 and 4 ppm, respectively (Figure S2). The latter upfield shift is characteristic of a tetracoordinate boronate complex (Wrackmeyer, 2008), supporting the hypothesis presented in Figure 2.
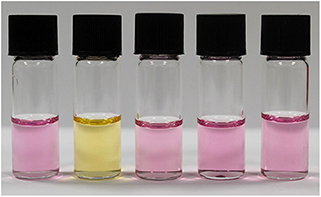
Figure 4. Visual selectivity test of NAz-6-Bpin in THF (0.5 mM) and halide analyte, 1:1. From left to right: no analyte, TBAF, TBACl, TBABr, TBAI. Photo taken immediately after addition of TBAX salt.
NAz-6-Bpin was then titrated against TBAF in THF (Figure 5), for which an isosbestic point at 507 nm was observed. The absorbance at 464 nm did not increase beyond the addition of 1 equivalent of TBAF, and the variation of observed absorbance at 464 nm with equivalents of TBAF shows a good linear correlation in the region from 0 to 1.0 equivalents (r2 = 0.992, Figure S3). Furthermore, a Job Plot of the interaction of NAz-6-Bpin and TBAF indicates a 1:1 stoichiometry of fluoride binding (Figure S4). With TBAF in THF, the limit of detection is 1.68 mg L−1 (Figure S5).
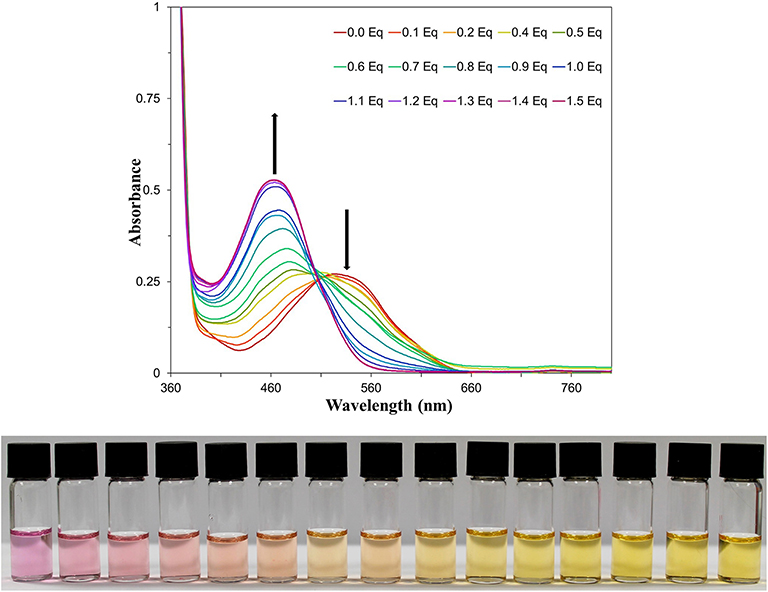
Figure 5. (Top) UV-vis titration of NAz-6-Bpin in THF (0.5 mM) with TBAF. (Bottom) Titration image of NAz-6-Bpin in THF (0.5 mM) with TBAF as a solution in THF. Equivalents of TBAF increase from left to right by 0.1 equivalents, starting at 0 equivalents and reaching 1.4 equivalents.
The ability of NAz-6-Bpin to detect fluoride in water/organic solvent mixtures was then evaluated. Exploratory experiments used a 1:1 mixture of water/organic solvent and an excess of NaF (10 equivalents), with THF, MeCN, EtOH, and MeOH being assessed (Figure 6). A mixed solvent system of DMSO/water caused NAz-6-Bpin to precipitate upon mixing. The most noticeable color change was observed in the MeOH/water system, with no further changes after 5 min. Complete UV-vis data were collected (Figure S6).
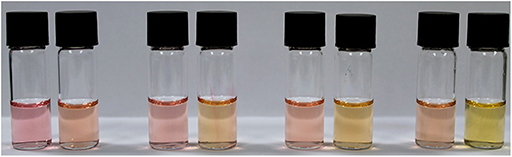
Figure 6. Visible assessment of color change from 1:1, v/v, aqueous/organic mixtures of NAz-6-Bpin (0.5 mM) and NaF (10 eq.). From left to right: THF, MeCN, EtOH, MeOH. Incubation time 5 min.
Whilst the methanol/water solvent system gave the most pronounced color change, further experiments were conducted on the EtOH/water system. It was reasoned that for the testing of fluoride by non-experts in the field, use of an ethanol-based system would prove more advantageous. Ethanol is appreciably less toxic than methanol, widely available, and low cost. The solvent ratio was explored and the optimum ratio was found to be 3:7 EtOH/H2O. However, the assay is robust with regard to the precise solvent ratio, with obvious color changes observed with ethanol proportions between 10 and 90% (Figure S7).
The optimized system was then titrated with increasing concentrations of fluoride (Figure 7). An obvious color change was observed from red-pink to yellow-orange after 10–20 equivalents of NaF were added. Increased equivalents of NaF were used to determine the maximum UV-vis response (Figure S8). The requirement for an excess of fluoride to induce the maximal spectroscopic response is likely due to the extensive solvation shell of the fluoride anion in water and competition with hydroxide for binding to boron. Fitting of the data in Figure S8 to a Langmuir isotherm allowed the association constant to be determined as KA = 214 M−1 at [NAz-6-Bpin] = 0.5 mM (Figure S9). The absorption maximum underwent a hypsochromic shift from λmax = 486 nm (NAz-6-Bpin), to λmax = 462 nm (NAz-6-Bpin + F−) and an isosbestic point at 502 nm was observed. NAz-6-Bpin was able to detect fluoride at neutral and basic pH levels (Figure S10). Acidic pH levels were avoided to prevent the generation of HF. Without the presence of fluoride, the sensor was stable and did not trigger a false response between pH 3–9. At pH 10, without the presence of NaF a minor color change was observed (Figure S11). NAz-6-Bpin was also assessed in a mixed ethanol: phosphate-buffered saline (PBS) solution, 3:7, v/v. It was found that the sensor changed color without the presence of fluoride, likely due to the coordination of the phosphate anion to the vacant p-orbital on the boronic ester (Figure S12). The pH stability of NAz-6-Bpin consequently means that a buffer is unnecessary. This is advantageous in the context of NAz-6-Bpin being used by non-expert users without access to such chemicals as buffer salts.
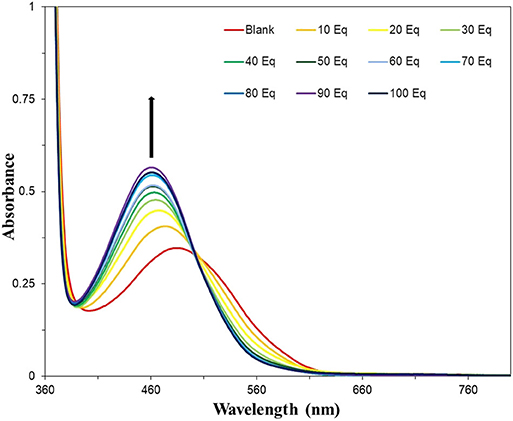
Figure 7. UV-vis titration of NAz-6-Bpin in EtOH/H2O, 3:7, v/v (0.5 mM) with NaF. Each sample was incubated for 30 min prior to acquisition of spectra.
A range of anions commonly found in drinking water were also assessed in the 3:7 EtOH/water solvent system (Figure 8), for which the distinct red-pink to yellow-orange color change occurred only for NaF. A small (~6 nm) shift of the absorption maximum for Na2SO4 was observed in the UV-vis absorbance spectra (Figure S13) but this is difficult to see with the naked eye. Finally, the UV-vis limit of detection of NAz-6-Bpin and NaF in the 3:7, EtOH/water, v/v system was found to be 5.75 mg L−1 (Figure S14). Visually, the limit of detection was found to be 10 mg L−1 (Figure S15).
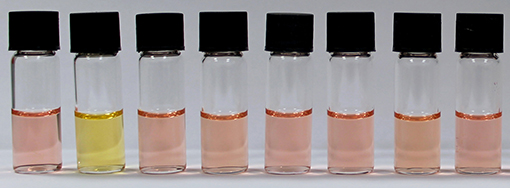
Figure 8. Visual selectivity test of NAz-6-Bpin in EtOH/H2O, 3:7, v/v (0.5 mM), with 60 equivalents of analyte used. From left to right: no analyte, NaF, NaCl, NaBr, NaI, NaNO3, NH4Cl, and Na2SO4. Each sample was incubated for 30 min prior to acquisition of spectra.
Conclusion
We have designed, synthesized and evaluated an azulene-based colorimetric fluoride sensor, NAz-6-Bpin, that can successfully detect fluoride in water over other halides and common anions. The sensor has an advantage over our previously published probe Az-1-Bpin, as it is able to function in a mixed water-ethanol solvent system, without the need for any surfactant. The binary solvent system of ethanol and water used in this system renders it potentially applicable for the detection of fluoride in drinking water in the field.
Methods
Synthesis of NAz-6-Bpin
Under atmospheric conditions, isoamyl nitrite (0.81 mL, 6.05 mmol, 5.0 eqv) and chlorotrimethylsilane (0.78 mL, 6.05 mmol, 5.0 eqv) were dissolved in CH2Cl2 (20 mL) and left to stir for 15 min, affording a pale-yellow solution. Diethyl 2-aminoazulene-1,3-dicarboxylate 1 (0.50 g, 1.21 mmol, 1.0 eqv) was added to the solution as a single portion, causing the mixture to immediately turn dark brown and bubble rapidly, before acquiring a dark purple color over a period of 1 h. The reaction was left to stir at room temperature for 13 h, after which the volatiles were removed in vacuo. The crude product was purified by flushing through a silica plug, from a which a single purple band was eluted with EtOAc/Petrol (1:4) to give diethyl 2-chloro-6-(4,4,5,5-tetramethyl-1,3,2-dioxaborolan-2-yl)azulene-1,3-dicarboxylate (NAz-6-Bpin, 0.50 g, 96%) as a purple solid. δH (500 MHz, CDCl3) 9.54–9.42 (2H, m, H4, H8), 8.28–8.18 (2H, m, H5, H7), 4.49 (4H, q, J 7.1 Hz, CH2), 1.47 (6H, t, J 7.1 Hz, CH2CH3), 1.40 (12H, s, C(CH3)2). δC (125 MHz, CDCl3) 164.4 (C=O), 145.0 (C2), 142.9 (C3a, C8a), 137.04 (C4, C8), 136.99 (C5, C7), 115.3 (C1, C3), 85.3 (C(CH3)2), 60.8 (CH2), 25.1 (C(CH3)2), 14.6 (CH2CH3). The resonance for C6 was not observed. HRMS (ESI+) m/z calcd for (C22H26BO6Cl+Na)+, 455.1407; found 455.1437. Analytical data in agreement with those previously reported (Xin et al., 2016).
For the complete synthesis and analysis of NAz-6-Bpin, please see the corresponding Supplementary Material.
Data Availability Statement
All datasets generated for this study are included in the article/Supplementary Material.
Author Contributions
SL, TJ, JW, and LM conceived the project idea. LM synthesized NAz-6-Bpin. KC, LM, and GW evaluated NAz-6-Bpin as a probe for fluoride anion. LM, AJ, and KC analyzed the data. CL and LM conducted the NMR experiments. SL and LM wrote the manuscript. All authors approved the manuscript.
Funding
We thank the EPSRC for DTP Ph.D. funding to LM, and the EPSRC and Public Health England for funding to GW. The Royal Society is gratefully acknowledged for a Wolfson merit award to TJ.
Conflict of Interest
The authors declare that the research was conducted in the absence of any commercial or financial relationships that could be construed as a potential conflict of interest.
Acknowledgments
NMR and MS facilities were provided through the Material and Chemical Characterization Facility (MC2) at the University of Bath.
Supplementary Material
The Supplementary Material for this article can be found online at: https://www.frontiersin.org/articles/10.3389/fchem.2020.00010/full#supplementary-material
References
Amini, M., Mueller, K., Abbaspour, K. C., Rosenberg, T., Afyuni, M., Moller, K. N., et al. (2008). Statistical modeling of global geogenic fluoride contamination in groundwaters. Environ. Sci. Technol. 42, 3662–3668. doi: 10.1021/es071958y
Bagutski, V., del Grosso, A., Carrillo, J. A., Cade, I. A., Helm, M. D., Lawson, J. R., et al. (2013). Mechanistic studies into amine-mediated electrophilic arene borylation and its application in MIDA boronate synthesis. J. Am. Chem. Soc. 135, 474–487. doi: 10.1021/ja3100963
Baker, M. S., and Phillips, S. T. (2012). A small molecule sensor for fluoride based on an autoinductive, colorimetric signal amplification reaction. Org. Biomol. Chem. 10, 3595–3599. doi: 10.1039/c2ob25363a
Birzan, L., Cristea, M., Draghici, C. C., Tecuceanu, V., Hanganu, A., Ungureanu, E.-M., et al. (2017). 4-(Azulen-1-yl) six-membered heteroaromatics substituted in 2- and 6- positions with 2-(2-furyl)vinyl, 2-(2-thienyl)vinyl or 2-(3-thienyl)vinyl moieties. Tetrahedron 73, 2488–2500. doi: 10.1016/j.tet.2017.03.035
Black, C. B., Andrioletti, B., Try, A. C., Ruiperez, C., and Sessler, J. L. (1999). Dipyrrolylquinoxalines: efficient sensors for fluoride anion in organic solution. J. Am. Chem. Soc. 121, 10438–10439. doi: 10.1021/ja992579a
Bozdemir, O. A., Sozmen, F., Buyukcakir, O., Guliyev, R., Cakmak, Y., and Akkaya, E. U. (2010). Reaction-based sensing of fluoride ions using built-in triggers for intramolecular charge transfer and photoinduced electron transfer. Org. Lett. 12, 1400–1403. doi: 10.1021/ol100172w
Buica, G.-O., Ivanov, A. A., Lazar, I.-G., Tatu (Arnold), G.-L., Omocea, C., Birzan, L., et al. (2019). Colorimetric and voltammetric sensing of mercury ions using 2,2′-(ethane-1,2-diylbis((2-(azulen-2-ylamino)-2-oxoethyl)azanediyl))diacetic acid. J. Electroanal. Chem. 849, 113351. doi: 10.1016/j.jelechem.2019.113351
Buica, G. O., Lazar, I. G., Birzan, L., Lete, C., Prodana, M., Enachescu, M., et al. (2018). Azulene-ethylenediaminetetraacetic acid: a versatile molecule for colorimetric and electrochemical sensors for metal ions. Electrochim. Acta 263, 382–390. doi: 10.1016/j.electacta.2018.01.059
Butler, S. J. (2015). Quantitative determination of fluoride in pure water using luminescent europium complexes. Chem. Commun. 51, 10879–10882. doi: 10.1039/C5CC03428K
Cabarcos, O. M., Weinheimer, C. J., Lisy, J. M., and Xantheas, S. S. (1999). Microscopic hydration of the fluoride anion. J. Chem. Phys. 110, 5–8. doi: 10.1063/1.478075
Calderon-Ortiz, L. K., Tauscher, E., Bastos, E. L., Gorls, H., Weiss, D., and Beckert, R. (2012). Hydroxythiazole-based fluorescent probes for fluoride ion detection. Eur. J. Org. Chem. 2535–2541. doi: 10.1002/ejoc.201200140
Cametti, M., and Rissanen, K. (2009). Recognition and sensing of fluoride anion. Chem. Commun. 2809–2829. doi: 10.1039/b902069a
Chansaenpak, K., Kamkaew, A., Weeranantanapan, O., Suttisintong, K., and Tumcharern, G. (2018). Coumarin probe for selective detection of fluoride ions in aqueous solution and its bioimaging in live cells. Sensors 18 2042/1–2042/16. doi: 10.3390/s18072042
Chavali, R., Gunda, N. S. K., Naicker, S., and Mitra, S. K. (2015). Rapid detection of fluoride in potable water using a novel fluorogenic compound 7-O-tert-butyldiphenylsilyl-4-methylcoumarin. Anal. Chem. Res. 26, 26–31. doi: 10.1016/j.ancr.2015.10.003
Chen, P., Bai, W., and Bao, Y. (2019). Fluorescent chemodosimeters for fluoride ions via silicon-fluorine chemistry: 20 years of progress. J. Mater. Chem. C 7, 11731–11746. doi: 10.1039/C9TC04567H
DenBesten, P., and Li, W. (2011). Chronic fluoride toxicity: dental fluorosis. Monogr. Oral Sci. 22, 81–96. doi: 10.1159/000327028
Descalzo, A. B., Jiménez, D., El Haskouri, J., Beltrán, D., Amorós, P., Marcos, M. D., et al. (2002). A new method for fluoride determination by using fluorophores and dyes anchored onto MCM-41. Chem. Commun. 2002, 562–563. doi: 10.1039/b111128k
Elsayed, S., Agostini, A., Santos-Figueroa, L. E., Martinez-Manez, R., and Sancenon, F. (2013). An instantaneous and highly selective chromofluorogenic chemodosimeter for fluoride anion detection in pure water. ChemistryOpen 2, 58–62. doi: 10.1002/open.201300010
Fang, H., Gan, Y. T., Wang, S. R., and Tao, T. (2018). A selective and colorimetric chemosensor for fluoride based on dimeric azulene boronate ester. Inorg. Chem. Commun. 95, 17–21. doi: 10.1016/j.inoche.2018.06.025
Fawell, J., Bailey, K., Chilton, J., Dahi, E., Fewtrell, L., and Magara, Y. (2006). Fluoride in Drinking-Water. Geneva: World Health Organization.
Gabrielli, L., and Mancin, F. (2016). Minimal self-immolative probe for multimodal fluoride detection. J. Org. Chem. 81, 10715–10720. doi: 10.1021/acs.joc.6b01787
Ghosh, A., Mukherjee, K., Ghosh, S. K., and Saha, B. (2013). Sources and toxicity of fluoride in the environment. Res. Chem. Intermediat. 39, 2881–2915. doi: 10.1007/s11164-012-0841-1
Gunnlaugsson, T., Davis, A. P., and Glynn, M. (2001). Fluorescent photoinduced electron transfer (PET) sensing of anions using charge neutral chemosensors. Chem. Commun. 2556–2557. doi: 10.1039/b107608f
Gunnlaugsson, T., Davis, A. P., Hussey, G. M., Tierney, J., and Glynn, M. (2004). Design, synthesis and photophysical studies of simple fluorescent anion PET sensors using charge neutral thiourea receptors. Org. Biomol. Chem. 2, 1856–1863. doi: 10.1039/b404706k
Gunnlaugsson, T., Kruger, P. E., Jensen, P., Tierney, J., Ali, H. D. P., and Hussey, G. M. (2005). Colorimetric “naked eye” sensing of anions in aqueous solution. J. Org. Chem. 70, 10875–10878. doi: 10.1021/jo0520487
Hu, R., Feng, J. A., Hu, D. H., Wang, S. Q., Li, S. Y., Li, Y., et al. (2010). A Rapid aqueous fluoride ion sensor with dual output modes. Angew. Chem. Int. Ed. 49, 4915–4918. doi: 10.1002/anie.201000790
Jagtap, S., Yenkie, M. K., Labhsetwar, N., and Rayalus, S. (2012). Fluoride in drinking water and defluoridation of water. Chem. Rev. 112, 2454–2466. doi: 10.1021/cr2002855
Kim, T. H., and Swager, T. M. (2003). A Fluorescent self-amplifying wavelength-responsive sensory polymer for fluoride ions. Angew. Chem. Int. Ed. 42, 4803–4806. doi: 10.1002/anie.200352075
Kim, Y., and Gabbaï, F. P. (2009). Cationic boranes for the complexation of fluoride ions in water below the 4 ppm maximum contaminant level. J. Am. Chem. Soc. 131, 3363–3369. doi: 10.1021/ja8091467
Kurotobi, K., Miyauchi, M., Takakura, K., Murafuji, T., and Sugihara, Y. (2003). Direct introduction of a boryl substituent into the 2-position of azulene: application of the Miyaura and Smith methods to azulene. Eur. J. Org. Chem. 3663–3665. doi: 10.1002/ejoc.200300001
Li, X., Hu, B., Li, J., Lu, P., and Wang, Y. (2014). Fluoride anion detection based on the excited state intramolecular proton transfer (ESIPT) of 2-(o-hydroxyphenyl) imidazole induced by the Si-O cleavage of its silyl ether. Sens. Actuat. B 203, 635–640. doi: 10.1016/j.snb.2014.07.014
Lichosyt, D., Dydio, P., and Jurczak, J. (2016). Azulene-based macrocyclic receptors for recognition and sensing of phosphate anions. Chem. Eur. J. 22, 17673–17680. doi: 10.1002/chem.201603555
Lichosyt, D., Wasiłek, S., Dydio, P., and Jurczak, J. (2018). The Influence of binding site geometry on anion-binding selectivity: a case study of macrocyclic receptors built on the azulene skeleton. Chem. Eur. J. 24, 11683–11692. doi: 10.1002/chem.201801460
Liu, R. S. H., and Asato, A. E. (2003). Tuning the color and excited state properties of the azulenic chromophore: NIR absorbing pigments and materials. J. Photochem. Photobiol. C 4, 179–194. doi: 10.1016/j.jphotochemrev.2003.09.001
Liu, T., Nonat, A., Beyler, M., Regueiro-Figueroa, M., Nono, K. N., Jeannin, O., et al. (2014). Supramolecular luminescent lanthanide dimers for fluoride sequestering and sensing. Angew. Chem. Int. Ed. 53, 7259–7263. doi: 10.1002/anie.201404847
Lopez-Alled, C. M., Sanchez-Fernandez, A., Edler, K. J., Sedgwick, A. C., Bull, S. D., McMullin, C. L., et al. (2017). Azulene-boronate esters: colorimetric indicators for fluoride in drinking water. Chem. Commun. 53, 12580–12583. doi: 10.1039/C7CC07416F
Lu, H., Wang, Q., Li, Z., Lai, G., Jiang, J., and Shen, Z. (2011). A specific chemodosimeter for fluoride ion based on a pyrene derivative with trimethylsilylethynyl groups. Org. Biomol. Chem. 9, 4558–4562. doi: 10.1039/c1ob05164d
Mahapatra, A. K., Manna, S. K., Pramanik, B., Maiti, K., Mondal, S., Ali, S. S., et al. (2015). Si bond cleavage. RSC Adv. 5, 10716–10722. doi: 10.1039/C4RA12910E
Mellerup, S. K.;, Rao, Y.-L., Amarne, H., and Wang, S. (2016). Tuning the colors of the dark isomers of photochromic boron compounds with fluoride ions: four-state color switching. Org. Lett. 18, 4436–4439. doi: 10.1021/acs.orglett.6b02308
Michl, J., and Thulstrup, E. W. (1976). Why is azulene blue and anthracene white? A simple MO picture. Tetrahedron 32, 205–209. doi: 10.1016/0040-4020(76)87002-0
Murfin, L. C., Lopez-Alled, C. M., Sedgwick, A. C., Wenk, J., James, T. D., and Lewis, S. E. (2020). A simple, azulene-based colorimetric probe for the detection of nitrite in water. Front. Chem. Sci. Eng. 14, 90–96. doi: 10.1007/s11705-019-1790-7
Murfin, L. C., Weber, M., Park, S. J., Kim, W. T., Lopez-Alled, C. M., McMullin, C. L., et al. (2019). Azulene-derived fluorescent probe for bioimaging: detection of reactive oxygen and nitrogen species by two-photon microscopy. J. Am. Chem. Soc. 141, 19389–19396. doi: 10.1021/jacs.9b09813
Nishimura, T., Xu, S. Y., Jiang, Y. B., Fossey, J. S., Sakurai, K., Bull, S. D., et al. (2013). A simple visual sensor with the potential for determining the concentration of fluoride in water at environmentally significant levels. Chem. Commun. 49, 478–480. doi: 10.1039/C2CC36107H
Nozoe, T., Seto, S., Matsumura, S., and Murase, Y. (1962). The synthesis of azulene derivatives from troponoids. Bull. Chem. Soc. Jpn. 35, 1179–1188. doi: 10.1246/bcsj.35.1179
Ozsvath, D. L. (2009). Fluoride and environmental health: a review. Rev. Environ. Sci. Biol. 8, 59–74. doi: 10.1007/s11157-008-9136-9
Peng, X. J., Wu, Y. K., Fan, J. L., Tian, M. Z., and Han, K. L. (2005). Colorimetric and ratiometric fluorescence sensing of fluoride: tuning selectivity in proton transfer. J. Org. Chem.70, 10524–10531. doi: 10.1021/jo051766q
Petersen, P. E., Bourgeois, D., Ogawa, H., Estupinan-Day, S., and Ndiaye, C. (2005). The global burden of oral diseases and risks to oral health. B. World Health Organ. 83, 661–669.
Qiu, B., Zeng, Y., Cao, L. X., Hu, R., Zhang, X. H., Yu, T. J., et al. (2016). A colorimetric and ratiometric fluorescence sensor for sensitive detection of fluoride ions in water and toothpaste. RSC Adv. 6, 49158–49163. doi: 10.1039/C6RA04747E
Razus, A. C., Birzan, L., Cristea, M., Tecuceanu, V., Hanganu, A., and Enache, C. (2011). 4-(Azulen-1-yl) six-membered heteroaromatics substituted with thiophen-2-yl or furan-2-yl moieties in 2 and 6 positions. J. Het. Chem. 48, 1019–1027. doi: 10.1002/jhet.684
Roy, A., Saha, T., and Talukdar, P. (2015). Turn-on fluorescent probe designed for fluoride ion sensing in aqueous media. Tetrahedron Lett. 56, 4975–4979. doi: 10.1016/j.tetlet.2015.06.086
Salman, H., Abraham, Y., Tal, S., Meltzman, S., Kapon, M., Tessler, N., et al. (2005). 1,3-Di(2-pyrrolyl)azulene: an Efficient luminescent probe for fluoride. Eur. J. Org. Chem. 2207–2212. doi: 10.1002/ejoc.200500012
Selwitz, R. H., Ismail, A. I., and Pitts, N. B. (2007). Dental caries. Lancet 369, 51–59. doi: 10.1016/S0140-6736(07)60031-2
Sessler, J. L., Maeda, H., Mizuno, T., Lynch, V. M., and Furuta, H. (2002). Quinoxaline-oligopyrroles: improved pyrrole-based anion receptors. Chem. Commun. 2002, 862–863. doi: 10.1039/b111708d
Singhal, P., and Jha, S. K. (2019). A semi quantitative visual probe for fluoride ion sensing in aqueous medium. J. Luminescence 206, 113–119. doi: 10.1016/j.jlumin.2018.10.065
Sole, S., and Gabbaï, F. P. (2004). A bidentate borane as colorimetric fluoride ion sensor. Chem. Commun. 1284–1285. doi: 10.1039/B403596H
Tao, T., Zhao, J., Chen, H., Mao, S., Yua, J., and Huang, W. (2019). Precisely controlling fluorescence enhancement and high-contrast colorimetric assay in OFF-ON fluoride sensing based on a diketopyrrolopyrrole boronate ester. Dyes Pigments 170:107638. doi: 10.1016/j.dyepig.2019.107638
Turan, I. S., and Akkaya, E. U. (2014). Chemiluminescence sensing of fluoride ions using a self-immolative amplifier. Org. Lett. 16, 1680–1683. doi: 10.1021/ol5003412
Wade, C. R., Broomsgrove, A. E. J., Aldridge, S., and Gabbaï, F. P. (2010). Fluoride ion complexation and sensing using organoboron compounds. Chem. Rev. 110, 3958–3984. doi: 10.1021/cr900401a
Wakabayashi, S., Kato, Y., Mochizuki, K., Suzuki, R., Matsumoto, M., Sugihara, Y., et al. (2007). Pyridylazulenes: synthesis, color changes, and structure of the colored product. J. Org. Chem. 72, 744–749. doi: 10.1021/jo061684h
Wakabayashi, S., Uchida, M., Tanaka, R., Habata, Y., and Shimizu, M. (2013). Synthesis of azulene derivatives that have an azathiacrown ether moiety and their selective color reaction towards silver ions. Asian J. Org. Chem. 2, 786–791. doi: 10.1002/ajoc.201300131
Wakabayashi, S., Uriu, R., Asakura, T., Akamatsu, C., and Sugihara, Y. (2008). Synthesis of 1,3-di(2-thiazolyl)azulene and its selective chromogenic response to mercury(ii) ion. Heterocycles 75, 383–390. doi: 10.3987/COM-07-11229
Wakabayashi, S., Yamaoka, R., Matsumoto, E., Nishiguchi, M., Ishiura, M., Tsuji, M., et al. (2012). Sulfur-containing pyridylazulenes: synthesis and chromogenic behaviors for heavy metal ions. Heterocycles 85, 2251–2258. doi: 10.3987/COM-12-12536
Wallabregue, A., Moreau, D., Sherin, P., Lorente, P. M., Jarolimova, Z., Bakker, E., et al. (2016). Selective imaging of late endosomes with a pH-sensitive diazaoxatriangulene fluorescent probe. J. Am. Chem. Soc. 138, 1752–1755. doi: 10.1021/jacs.5b09972
Wang, H., Fan, P. H., Tong, B., Dong, Y. P., Ou, X. M., Li, F., et al. (2015). Hydrogen-terminated Si nanowires as label-free colorimetric sensors in the ultrasensitive and highly selective detection of fluoride anions in pure water phase. Adv. Funct. Mater. 25, 1506–1510. doi: 10.1002/adfm.201401632
World Health Organization (2011). Guidelines for Drinking-Water Quality, 4th Edn. Geneva: World Health Organization.
Wrackmeyer, B. (2008). “Organoboron chemistry” in Modern Magnetic Resonance, ed G. A. Webb (Dordrecht: Springer), 455–457. doi: 10.1007/1-4020-3910-7_56
Xin, H., Ge, C., Jiao, X., Yang, X., Rundel, K., McNeill, C. R., et al. (2018). Incorporation of 2,6-connected azulene units into the backbone of conjugated polymers: towards high-performance organic optoelectronic materials. Angew. Chem. Int. Ed. 57, 1322–1326. doi: 10.1002/anie.201711802
Xin, H., Li, J., Yang, X., and Gao, X. (2020). Azulene-based BN-heteroaromatics. J. Org. Chem. 85, 70–78. doi: 10.1021/acs.joc.9b01724
Xin, H. S., Ge, C. W., Yang, X. D., Gao, H. L., Yang, X. C., and Gao, X. K. (2016). Biazulene diimides: a new building block for organic electronic materials. Chem. Sci. 7, 6701–6705. doi: 10.1039/C6SC02504H
Yamaguchi, S., Akiyama, S., and Tamao, K. (2000). Photophysical properties changes caused by hypercoordination of organosilicon compounds: from trianthrylfluorosilane to trianthryldifluorosilicate. J. Am. Chem. Soc. 122, 6793–6794. doi: 10.1021/ja001042q
Yamaguchi, S., Akiyama, S., and Tamao, K. (2001). Colorimetric fluoride ion sensing by boron-containing π-electron systems. J. Am. Chem. Soc. 123, 11372–11375. doi: 10.1021/ja015957w
Zhan, C. G., and Dixon, D. A. (2004). Hydration of the fluoride anion: structures and absolute hydration free energy from first-?principles electronic structure calculations. J. Phys. Chem. A 108, 2020–2029. doi: 10.1021/jp0311512
Zhou, Y., Zhang, J. F., and Yoon, J. (2014). Fluorescence and colorimetric chemosensors for fluoride-ion detection. Chem. Rev. 114, 5511–5571. doi: 10.1021/cr400352m
Zhu, C.-Q., Chen, J.-L., Zheng, H., Wu, Y.-Q., and Xu, J.-G. (2005). A colorimetric method for fluoride determination in aqueous samples based on the hydroxyl deprotection reaction of a cyanine dye. Anal. Chim. Acta 539, 311–316. doi: 10.1016/j.aca.2005.03.002
Keywords: azulene, sensor, fluoride, colorimetric, boron, water sensing
Citation: Murfin LC, Chiang K, Williams GT, Lyall CL, Jenkins ATA, Wenk J, James TD and Lewis SE (2020) A Colorimetric Chemosensor Based on a Nozoe Azulene That Detects Fluoride in Aqueous/Alcoholic Media. Front. Chem. 8:10. doi: 10.3389/fchem.2020.00010
Received: 31 October 2019; Accepted: 07 January 2020;
Published: 29 January 2020.
Edited by:
Karl J. Wallace, University of Southern Mississippi, United StatesCopyright © 2020 Murfin, Chiang, Williams, Lyall, Jenkins, Wenk, James and Lewis. This is an open-access article distributed under the terms of the Creative Commons Attribution License (CC BY). The use, distribution or reproduction in other forums is permitted, provided the original author(s) and the copyright owner(s) are credited and that the original publication in this journal is cited, in accordance with accepted academic practice. No use, distribution or reproduction is permitted which does not comply with these terms.
*Correspondence: A. Toby A. Jenkins, YS50LmEuamVua2lucyYjeDAwMDQwO2JhdGguYWMudWs=; Jannis Wenk, ai5oLndlbmsmI3gwMDA0MDtiYXRoLmFjLnVr; Tony D. James, dC5kLmphbWVzJiN4MDAwNDA7YmF0aC5hYy51aw==; Simon E. Lewis, cy5lLmxld2lzJiN4MDAwNDA7YmF0aC5hYy51aw==