- 1School of Materials and Energy, University of Electronic Science and Technology of China, Chengdu, China
- 2Center of Excellence in Transportation Electrification and Energy Storage, Hydro-Québec, Varennes, QC, Canada
The intrinsic bottleneck of graphite intercalation compound mechanism in potassium-ion batteries necessitates the exploitation of novel potassium storage strategies. Hence, utmost efforts have been made to efficiently utilize the extrinsic pseudo-capacitance, which offers facile routes by employing low-cost carbonaceous anodes to improve the performance of electrochemical kinetics, notably facilitating the rate and power characteristics for batteries. This mini-review investigates the methods to maximize the pseudo-capacitance contribution based on the size control and surface activation in recent papers. These methods employ the use of cyclic voltammetry for kinetics analysis, which allows the quantitative determination on the proportion of diffusion-dominated vs. pseudo-capacitance by verifying a representative pseudo-capacitive material of single-walled carbon nanotubes. Synergistically, additional schemes such as establishing matched binder–electrolyte systems are in favor of the ultimate purpose of high-performance industrialized potassium-ion batteries.
Introduction
Suffering from the geopolitical maldistribution of lithium resources, sodium-ion batteries (SIBs) and potassium-ion batteries (PIBs) reach a hotspot in view of wider resource reserves compared with lithium-ion batteries (LIBs) (2.09 wt% of K vs. 2.36 wt% of Na vs. 0.0017 wt% of Li) (Carmichael, 1989; Larcher and Tarascon, 2015). Significantly, the PIB system has the lowest negative potential (0.15 V below the Li/Li+) (Komaba et al., 2015; Eftekhari et al., 2016; Wang et al., 2018a,b) and satisfactory electrochemical kinetics in ionic diffusion kinetics and conductivity theoretically (Okoshi et al., 2013, 2017; Komaba et al., 2015; Eftekhari et al., 2016; Su et al., 2016) in non-aqueous electrolytes, ascribed to low de-solvation due to its weak Lewis acid character (Okoshi et al., 2017; Lei et al., 2018). Similar to the behavior of LIBs in graphite (Wang, 2017), the intercalation mechanism of PIBs involves three potassiation stages, generating the KC36 in Stage III, KC24 in Stage II, and finally the KC8 in Stage I (Jian et al., 2017) with 270 mA h g−1, which is far more stable than SIBs (Wang et al., 2013; Zheng et al., 2017). Nevertheless, large Shannon ionic radius (K+ = 1.38 Å, Na+ = 1.02 Å, Li+ = 0.76 Å) and atomic mass (K = 39.10, Na = 22.99, Li = 6.94) (Shannon, 1976) have decreased the theoretical capacity and induced high volume expansion of 61% (Wen et al., 2015; Eftekhari et al., 2016; Zou et al., 2017). Although some strategies enter into consideration such as adopting expand graphite (An et al., 2018), implementing solvent co-intercalation (SCI) (Wang et al., 2013, 2019; David and Singh, 2014), and developing dual-carbon batteries (DCBs) (Carlin et al., 1996, 2010; Beltrop et al., 2017; Fan et al., 2017; Ji et al., 2017), essential kinetics deficiency is hard to surmount.
To address the irreversible expansion induced by equilibrium graphite, amorphous carbons come into the focused sight (Xing et al., 2017), which are admittedly classified as hard carbon (HC) and soft carbon (SC). HC is proven to have a prolonged cycling ability for its randomly oriented bend graphitic layers along the c axis without observed expansion after a thorough potassiation (Jian et al., 2016, 2017). On the contrary, SC is easily graphitized with turbostratic domains although far from the commercial graphite. SC presents obvious expansion to HC if undergoing complete potassiation (Luo et al., 2015a; Wang et al., 2017). Nonetheless, SC has a better rate performance than HC for more aligned domains (Jian et al., 2017), regarded as the reason for the better rate performance.
Pseudo-capacitance is the middle part of the battery and electrical double-layer capacitors (EDLCs) (Jiang and Liu, 2019) as shown in Figure 1a. In Figure 1a, Liu points out that the current generation of batteries depends on the Faradic electron transfer from the surface to the metal center based on the charge-compensating ions by intercalation or adsorption. In contrast, a pseudo-capacitor is different from EDLCs because it is not electrostatic-induced and the transfer process of surface electrons distinguishes the behavior from batteries. Pseudo-capacitance can be classified into two categories—intrinsic and extrinsic. The former (Chao et al., 2016) describes an inherent feature of specific materials such as RuO2 and MnO2, which is on the strength of Faradaic electron transfer. However, the latter emphasizes the technological means of low dimension, nanoscale size, and high surface area (Wang et al., 2007; Brezesinski et al., 2009; Muller et al., 2015; Cook et al., 2016) among a majority of materials, for instance, the single-walled carbon nanotube (SWCNT) with surface-enriched potassium ions in Figure 1b (Hersam, 2009; Kang et al., 2013), attributed to the regular hexagonal arrangement of carbon atoms on the surface. This non-Faradaic pathway provides a possibility to utilize inexpensive carbonaceous anodes (Gogotsi and Penner, 2018) if proper surface treatments such as activization, doping, and plasma processing have been undergone (Chao et al., 2018).
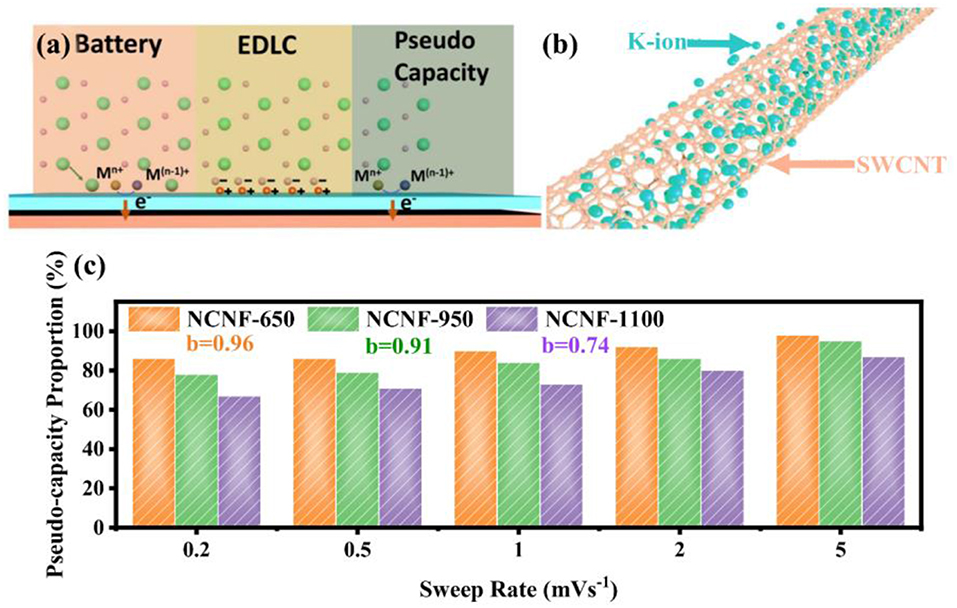
Figure 1. (a) Surface processes among battery, EDLC and pseudo-capacitance. (b) Diagram of the surface-dominated procedure for SWCNT; with permission from Wiley. (c) Pseudo-capacity proportion of NCNF-650, NCNF-950 and NCNF-1100 with b value; with permission from Springer.
These surface-dominated anodes uptake and absorb potassium ions with fast reaction kinetics during the electrochemistry process, avoiding the hindrance in the intercalation mechanism, which is regarded as the primary cause for high rate property and large capacity of PIBs undergoing charging and discharging processes (Shin et al., 2011; Shao et al., 2013; Chen et al., 2016; Long et al., 2016). As a consequence, this mini-review analyzes the methods for distinguishing the proportion of capacity contribution and summarizes the application of pseudo-capacitance to PIBs very recently, aiming to design a practical performance improvement approach.
Analyses And Applications Of Pseudo-Capacitance
Generally, it is widely admitted that pseudo-capacitance is not a pure Faradaic progress but a rapid reversible surface redox reaction involved in EDLCs. The charge storage mechanism of complex PIBs behaviors is composed of two typical contribution progresses: surface-induced pseudo-capacitor process and diffusion-dominated process (Brezesinski et al., 2009; Wen et al., 2015; Xu et al., 2019b). Nonetheless, Faradaic and non-Faradaic reactions are electro indistinguishable for jointly contributing to the current parameter (Gogotsi and Penner, 2018). Most researchers employ cyclic voltammetry (CV) to determine the relative proportion of contribution from pseudo-capacitor and diffusion-dominated processes. The peak current is proportional to the square root of sweep rate describing the reversible diffusion-limited state (i-), whereas it is proportional to the sweep rate (i-v) describing the capacitive state. A representative power law relationship between the current and scan rate reveals the charge storage mechanism in PIBs (Wang et al., 2007; Torsten et al., 2010; Veronica et al., 2013):
where a and b are constants. The b value can be figured out by profiling the log(i)–log(v) curve. If b = 0.5, the Faradic diffusion is predominant; while b = 1, the pseudo-capacitance assumes the primary contribution (Sathiya et al., 2011; Lijun et al., 2014; Zou et al., 2017). Furthermore, as for a fixed sweep rate, the specific pseudo-capacitance contribution can be given in detail by the following formula (Torsten et al., 2010; Wang Y. et al., 2016):
where the parameter k1v represents the capacitive process while the is in favor of the diffusion process as stated earlier.
Furthermore, Marveh maintains that compared with the CV method, the step potential electrochemical spectroscopy (SPECS) has wider adaptive range with prominent advantages. In high sweep rates, SPECS presents precise characterization to depict the process of electrical double layer on the surface of electrodes (Forghani and Donne, 2018).
Recent works validate the validity of the pseudo-capacitance algorithm based on the surface-dominated pseudo-capacitance mechanism, which has been extensively applied in carbonaceous anodes in PIBs by constructing high surface area or activating.
Doping and activating are highly feasible methods that introduce abundant defects, expand specific surface area, and promote the conductivity, meanwhile adding charge storage for PIBs (Lijun et al., 2014; Share et al., 2016; Chen et al., 2017; Lei et al., 2018; Xu et al., 2018).
Nitrogen-doped strategy has a practical significance eliciting satisfying performance enhancements. According to the X-ray photoelectron spectroscopy (XPS) results, pyrrole nitrogen (N-5), pyridine nitrogen (N-6), and quaternary N (N-Q) are three N-doping forms presented in Figure 2A, where N-5 and N-6 possess high electrochemical activity and generate additional defects in the surface of the graphene layer, hence promoting the adsorption quantity of potassium ions, accelerating the kinetic process (Li et al., 2013; Wang et al., 2014; Xu et al., 2018). This differentiation of N forms is ascribed to their constructions of respective bonding electrons, resulting in different chemical activities. However, N-Q, located in the internal surface of graphene layer, bonding with three sp2 carbon atoms, is beneficial to improve electrical conductivity (Yang et al., 2018). Notably, N-6 is regarded as the most effective doping precursor because it replaces the carbon atom with a nitrogen atom at the defect or the edge of the graphite plane and occupies abundant active centers to adsorb potassium ions (Ma et al., 2012; Ding et al., 2014; Xie et al., 2017). Consistently, recent researches demonstrated that N-6 defects decreases with temperature increasing; meanwhile, the degree of graphitization rises, accompanied by the generation of N-Q. Xu concludes that among three temperature-controlled materials NCNF-650, NCNF-950, and NCNF-1100 derived from poly-pyrrole nanofibers, the pseudo-capacitance contribution of NCNF-650 occupies 90% at 1 mV s−1 for abundant N-6 defects, while the others occupy 73 and 84% at 1 mV s−1 as displayed in Figure 1c with their b value (Xu et al., 2018). The b value increases with the temperature dropping, revealing the degree deepening of pseudo-capacity, in accordance with the quantity N-6 defects. Similar results are obtained in Xie's report; nonetheless, Xie indicates that enhancement of electrochemical performance is a comprehensive result associated with N-6 defects, electrical conductivity, and transfer resistance. Three sets of temperature-controlled experiments point out that PNCM-700 is equipped with the best comprehensive performance compared with PNCM-500 and PNCM-900. As for the function of defects, Clement states that the D-bond from the Raman spectrum is employed to describe the sp3 defect distribution, which intensifies a six-fold rate performance to the un-doped material (Clement et al., 2015; Share et al., 2016). The prominent significance of N-doping is to spread out the interlayer spacing and provide huge specific surface area to promote the pseudo-capacitive effect.
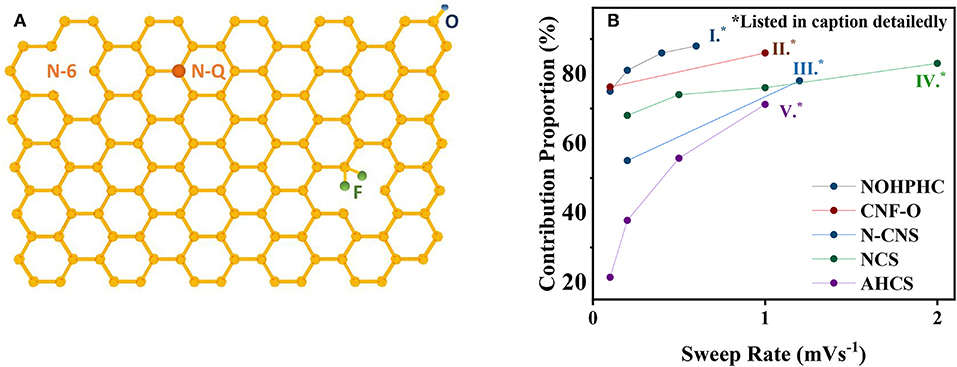
Figure 2. (A) Surface defects induced by N-doping, O-doping and F-doping. (B) Relationship between pseudo-capacitance contribution proportion and sweep rate based on recent researches (I) (Yang et al., 2018), (II) (Adams et al., 2017), (III) (Lei et al., 2018), (IV) (Chen et al., 2017), (V) (Wang et al., 2018).
Doping some other elements also achieves fair results. Oxidation functional groups on the carbon surface polishes up the wettability of carbon-based materials and advances the pseudo-capacitance behavior (Tarun et al., 2010; Shao et al., 2013; Wang X. et al., 2016; Wu et al., 2016; Xie et al., 2017; Wang et al., 2018). Adams reported that oxidation groups increase obviously on the surface capacitive storage while inducing the capacity reduction contributed by the intercalation mechanism. As a consequence, there is no significant enhancement to the total capacity (Adams et al., 2017). In addition, mixed-doping P and O doping (Ma et al., 2017) based on the triphenylphosphine precursor obtains a satisfactory capacity of 474 mA h g−1, benefiting from expanding the interlayer spacing; N and F doping immensely adds the conductivity distinctly (Ju et al., 2016; Share et al., 2016; Adams et al., 2017).
Activated hollow carbon nanospheres (HCS) underwent HF etching from C@SiO2 nanospheres in Wang's work. Wang emphasizes on the sharp increase on the surface area from 481.4 to 757.8 m2 g−1 after activating utilizing KOH as the activator. Capacitive contribution occupies 71.2% at a sweep rate of 1 mV s−1, leading to 192.7 mA h g−1 at 2 A g−1 after 5,000 cycles with a retention of 99.5% (Wang et al., 2018). Aforesaid data support the rule that the pseudo-capacity contribution has the tendency of positive correlation with sweep rate as summarized in Figure 2B. This work claimed that activated hollow carbon expands the layer spacing of the carbon anode and shortens the diffusion distance of K-ions.
Nevertheless, surface-modified strategies, whether doping or activating, may give rise to the decrease of initial Coulombic efficiency (ICE) unsatisfactorily. Compensatory methods work well in LIBs and SIBs (Suo et al., 2017) to establish appropriate binder–electrolyte systems (BESs), which directly impact the formation of solid electrolyte interphase (SEI), especially the morphology features such as thickness, pore, and wrinkle. Tailored BESs shape the SEI into a smooth and thin layer, hence improving the transfer efficiency of ions on the phase interface (Xu et al., 2019a). Similarly, employing KFSI and KTFSI electrolyte (Eftekhari et al., 2016; Jin et al., 2016), adding electrolyte additives (Wu et al., 2017), selecting hydrophilic binders such as CMC, PANa, and SA (Komaba et al., 2015; Luo et al., 2015b; Jin et al., 2016; Xu et al., 2019a), and utilizing pre-potassiation technique (Yang et al., 2018) serve the same purpose for superb PIBs.
Conclusions And Perspectives
PIBs with carbonaceous anodes provide the possibility for industrialization under controlled price. Facilely, surface modifications such as doping and activating obviously enhance the pseudo-capacitance contribution, speeding up the rate and power performance based on a rapid electrochemical kinetics.
This non-insertion charge storage (pseudo-capacitance absorption) integrates with both battery-type and capacitor-type characteristics, exhibiting distinct redox separation peaks including analogous linear capacitive voltage response. However, the relationship between capacitive and sweep rate is only authentic limited in a low and narrow sweep rate under the CV separation method. Precisely, the SPECS is suitable for a wider range of sweep rates, inducing detailed contribution information for each potential point (Forghani and Donne, 2018). In addition, matching binder–electrolyte with anodes accurately can synergistically promote specific capacity and rate properties, deriving high-performance PIBs.
Author Contributions
All authors listed have made a substantial, direct and intellectual contribution to the work, and approved it for publication.
Funding
This work was supported by the Sichuan Science and Technology Program (Grant Nos. 2018GZ0006 and 2018GZ0134).
Conflict of Interest
The authors declare that the research was conducted in the absence of any commercial or financial relationships that could be construed as a potential conflict of interest.
References
Adams, R. A., Syu, J. M., Zhao, Y., Lo, C. T., Varma, A., and Pol, V. G. (2017). Binder-free N- and O-rich carbon nanofiber anodes for long cycle life K-ion batteries. ACS Appl. Mater. Interfaces 9, 17872–17881. doi: 10.1021/acsami.7b02476
An, Y., Fei, H., Zeng, G., Ci, L., Xi, B., Xiong, S., et al. (2018). Commercial expanded graphite as a low-cost, long-cycling life anode for potassium–ion batteries with conventional carbonate electrolyte. J. Power Sources 378, 66–72. doi: 10.1016/j.jpowsour.2017.12.033
Beltrop, K., Beuker, S., Heckmann, A., Winter, M., Placke, T. J. E., and Science, E. (2017). Alternative electrochemical energy storage: Potassium-based dual-graphite batteries. Energy Environ. Sci. 10, 2090–2094. doi: 10.1039/C7EE01535F
Brezesinski, T., Wang, J., Polleux, J., Dunn, B., and Tolbert, S. H. (2009). Templated nanocrystal-based porous TiO(2) films for next-generation electrochemical capacitors. J. Am. Chem. Soc. 131, 1802–1809. doi: 10.1021/ja8057309
Carlin, R. T., Fuller, J., Kuhn, W. K., Lysaght, M. J., and Trulove, P. C. (1996). Electrochemistry of room-temperature chloroaluminate molten salts at graphitic and nongraphitic electrodes. J. Appl. Electrochem. 26, 1147–1160. doi: 10.1007/BF00243740
Carlin, R. T., Long, H. C. D., Fuller, J., and Trulove, P. C. (2010). ChemInform abstract: dual intercalating molten electrolyte batteries. Cheminform 25, L73–L76. doi: 10.1002/chin.199446010
Carmichael, R. S. (1989). CRC Practical Handbook of Physical Properties of Rocks and Minerals. Boca Raton, FL: CRC Press.
Chao, D., Ouyang, B., Liang, P., Huong, T. T. T., Jia, G., Huang, H., et al. (2018). C-plasma of hierarchical graphene survives SnS bundles for ultrastable and high volumetric Na-ion storage. Adv. Mater. 30:1804833. doi: 10.1002/adma.201804833
Chao, D., Zhu, C., Yang, P., Xia, X., Liu, J., Wang, J., et al. (2016). Array of nanosheets render ultrafast and high-capacity Na-ion storage by tunable pseudocapacitance. Nat. Commun. 7:12122. doi: 10.1038/ncomms12122
Chen, C., Wang, Z., Zhang, B., Miao, L., Cai, J., Peng, L., et al. (2017). Nitrogen-rich hard carbon as a highly durable anode for high-power potassium-ion batteries. Energy Storage Mater. 8, 161–168. doi: 10.1016/j.ensm.2017.05.010
Chen, C., Xu, H., Zhou, T., Guo, Z., Chen, L., Yan, M., et al. (2016). Integrated intercalation-based and interfacial sodium storage in graphene-wrapped porous Li4Ti5O12 nanofibers composite aerogel. Adv. Energy Mater. 6:1600322. doi: 10.1002/aenm.201600322
Clement, B., Todd Wesley, S., Michelle, D., and Xiulei, J. J. N. L. (2015). New mechanistic insights on Na-ion storage in nongraphitizable carbon. Nano Lett. 15, 5888–5892. doi: 10.1021/acs.nanolett.5b01969
Cook, J. B., Kim, H. S., Yan, Y., Ko, J. S., Robbennolt, S., Dunn, B., et al. (2016). Mesoporous MoS2 as a transition metal dichalcogenide exhibiting pseudocapacitive Li and Na-ion charge storage. Adv. Energy Mater. 6:1501937. doi: 10.1002/aenm.201501937
David, L., and Singh, G. (2014). Reduced graphene oxide paper electrode: opposing effect of thermal annealing on Li and Na cyclability. J. Phys. Chem. C 118, 28401–28408. doi: 10.1021/jp5080847
Ding, N., Huang, Z. H., Lv, R., Lu, Y., Wang, J., Shen, W., et al. (2014). Nitrogen-enriched electrospun porous carbon nanofiber networks as high-performance free-standing electrode materials. J. Mater. Chem. A 2, 19678–19684. doi: 10.1039/C4TA03868A
Eftekhari, A., Jian, Z., and Ji, X. (2016). Potassium secondary batteries. ACS Appl. Mater. Interfaces 9, 4404–4419. doi: 10.1021/acsami.6b07989
Fan, L., Liu, Q., Chen, S., Lin, K., Xu, Z., and Lu, B. J. S. (2017). Potassium-based dual ion battery with dual-graphite electrode. Small 13:1701011. doi: 10.1002/smll.201701011
Forghani, M., and Donne, S. W. (2018). Method comparison for deconvoluting capacitive and pseudo-capacitive contributions to electrochemical capacitor electrode behavior. J. Electrochem. Soc. 165, A664–A673. doi: 10.1149/2.0931803jes
Gogotsi, Y., and Penner, R. M. (2018). Energy storage in nanomaterials—Capacitive, pseudocapacitive, or battery-like? Acs Nano 12, 2081–2083. doi: 10.1021/acsnano.8b01914
Hersam, M. C. (2009). “Progress towards monodisperse single-walled carbon nanotubes,” in Nanoscience and Technology, ed P. Rodgers (London: Macmillan Publishers Ltd.), 3–10. doi: 10.1142/9789814287005_0001
Ji, B., Fan, Z., Wu, N., and Tang, Y. (2017). A dual-carbon battery based on potassium-ion electrolyte. Adv. Energy Mater. 7:1700920. doi: 10.1002/aenm.201700920
Jian, Z., Hwang, S., Li, Z., Hernandez, A. S., Wang, X., Xing, Z., et al. (2017). Hard–soft composite carbon as a long-cycling and high-rate anode for potassium-ion batteries. Adv. Funct. Mater. 27:1700324. doi: 10.1002/adfm.201700324
Jian, Z., Xing, Z., Bommier, C., Li, Z., and Ji, X. (2016). Hard carbon microspheres: potassium-ion anode versus sodium-ion anode. Adv. Energy Mater. 6:1501874. doi: 10.1002/aenm.201501874
Jiang, Y., and Liu, J. (2019). Definitions of pseudocapacitive materials: a brief review. Energy Environ. Sci. 2, 30–37. doi: 10.1002/eem2.12028
Jin, Z., Zou, X., Zhu, Y., Xu, Y., and Wang, C. (2016). Electrochemical intercalation of potassium into graphite. Adv. Funct. Mater. 26, 8103–8110. doi: 10.1002/adfm.201602248
Ju, Z., Zhang, S., Xing, Z., Zhuang, Q., Qiang, Y., and Qian, Y. (2016). Direct synthesis of few-layer F-doped graphene foam and its lithium/potassium storage properties. ACS Appl. Mater. Interfaces 8, 20682–20690. doi: 10.1021/acsami.6b04763
Kang, J., Wen, J., Jayaram, S. H., Wang, X., and Chen, S. K. (2013). Electrochemical characterization and equivalent circuit modeling of single-walled carbon nanotube (SWCNT) coated electrodes. J. Power Sources 234, 208–216. doi: 10.1016/j.jpowsour.2013.01.058
Komaba, S., Hasegawa, T., Dahbi, M., and Kubota, K. (2015). Potassium intercalation into graphite to realize high-voltage/high-power potassium-ion batteries and potassium-ion capacitors. Electrochem. Commun. 60, 172–175. doi: 10.1016/j.elecom.2015.09.002
Larcher, D., and Tarascon, J. M. (2015). Towards greener and more sustainable batteries for electrical energy storage. Nat. Chem. 7, 19–29. doi: 10.1038/nchem.2085
Lei, L., Yu, C., Xie, Y., Peng, T., Li, Q., and Yan, C. (2018). Understanding of the ultrastable K-ion storage of carbonaceous anode. Adv. Funct. Mater. 28:1801989. doi: 10.1002/adfm.201801989
Li, Z., Xu, Z., Tan, X., Wang, H., Holt, C. M. B., Stephenson, T., et al. (2013). Mesoporous nitrogen-rich carbons derived from protein for ultra-high capacity battery anodes and supercapacitors. Energy Environ. Sci. 6, 871–878. doi: 10.1039/c2ee23599d
Lijun, F., Kun, T., Kepeng, S., Van Aken, P. A., Yu, Y., and Maier, J. (2014). Nitrogen doped porous carbon fibres as anode materials for sodium ion batteries with excellent rate performance. Nanoscale 6, 1384–1389. doi: 10.1039/C3NR05374A
Long, Q., Chen, W., Xiong, X., Hu, C., Feng, Z., Hu, P., et al. (2016). Sulfur-doped carbon with enlarged interlayer distance as a high-performance anode material for sodium-ion batteries. Adv. Sci. 2:1500195. doi: 10.1002/advs.201500195
Luo, W., Jian, Z., Xing, Z., Wang, W., Bommier, C., Lerner, M. M., et al. (2015a). Electrochemically expandable soft carbon as anodes for Na-ion batteries. ACS Central Sci. 1, 516–522. doi: 10.1021/acscentsci.5b00329
Luo, W., Wan, J., Ozdemir, B., Bao, W., Chen, Y., Dai, J., et al. (2015b). Potassium ion batteries with graphitic materials. Nano Lett. 15, 7671–7677. doi: 10.1021/acs.nanolett.5b03667
Ma, C., Shao, X., and Cao, D. (2012). Nitrogen-doped graphene nanosheets as anode materials for lithium ion batteries: a first-principles study. J. Mater. Chem. A 22, 8911–8915. doi: 10.1039/c2jm00166g
Ma, G., Huang, K., Ma, J. S., Ju, Z., Xing, Z., and Zhuang, Q. C. (2017). Phosphorus and oxygen dual-doped graphene as superior anode material for room-temperature potassium-ion batteries. J. Mater. Chem. A 5, 7854–7861. doi: 10.1039/C7TA01108C
Muller, G. A., Cook, J., Kim, H. S., Tolbert, S. H., and Dunn, B. J. N. L. (2015). High performance pseudocapacitor based on 2D layered metal chalcogenide nanocrystals. Nano Lett. 15, 1911–1917. doi: 10.1021/nl504764m
Okoshi, M., Yamada, Y., Komaba, S., Yamada, A., and Nakai, H. (2017). Theoretical analysis of interactions between potassium ions and organic electrolyte solvents: a comparison with lithium, sodium, and magnesium ions. J. Electrochem. Soc. 164, A54–A60. doi: 10.1149/2.0211702jes
Okoshi, M., Yamada, Y., Yamada, A., and Nakai, H. (2013). Theoretical analysis on de-solvation of lithium, sodium, and magnesium cations to organic electrolyte solvents. J. Electrochem. Soc. 160, A2160–A2165. doi: 10.1149/2.074311jes
Sathiya, M., Prakash, A. S., Ramesha, K., Tarascon, J. M., and Shukla, A. K. (2011). V2O5-anchored carbon nanotubes for enhanced electrochemical energy storage. J. Am. Chem. Soc. 133, 16291–16299. doi: 10.1021/ja207285b
Shannon, R. (1976). Revised effective ionic radii and systematic studies of interatomic distances in halides and chalcogenides. Acta Crystallogr. Sect. A 32, 751–767. doi: 10.1107/S0567739476001551
Shao, Y., Xiao, J., Wang, W., Engelhard, M., Chen, X., Nie, Z., et al. (2013). Surface-driven sodium ion energy storage in nanocellular carbon foams. Nano Lett. 13, 3909–3914. doi: 10.1021/nl401995a
Share, K., Cohn, A. P., Carter, R., Rogers, B., and Pint, C. L. (2016). Role of nitrogen doped graphene for improved high capacity potassium ion battery anodes. Acs Nano 10, 9738–9744. doi: 10.1021/acsnano.6b05998
Shin, J. Y., Samuelis, D., and Maier, J. (2011). Sustained lithium-storage performance of hierarchical, nanoporous anatase TiO2 at high rates: emphasis on interfacial storage phenomena. Adv. Funct. Mater. 21, 3464–3472. doi: 10.1002/adfm.201002527
Su, D., Mcdonagh, A., Qiao, S. Z., and Wang, G. (2016). High-capacity aqueous potassium-ion batteries for large-scale energy storage. Adv. Mater. 29:1604007. doi: 10.1002/adma.201604007
Suo, L., Borodin, O., Wang, Y., Rong, X., Sun, W., Fan, X., et al. (2017). “Water-in-salt” electrolyte makes aqueous sodium-ion battery safe, green, and long-lasting. Adv. Energy Mater. 7:1701189. doi: 10.1002/aenm.201701189
Tarun, B., Aleks, A., Barbara, P., Veronica, B., and Fahlman, B. D. (2010). Enhanced electrochemical lithium storage by graphene nanoribbons. J. Am. Chem. Soc. 132, 12556–12558. doi: 10.1021/ja106162f
Torsten, B., John, W., Tolbert, S. H., and Bruce, D. (2010). Ordered mesoporous alpha-MoO3 with iso-oriented nanocrystalline walls for thin-film pseudocapacitors. Nat. Mater. 9, 146–151. doi: 10.1038/nmat2612
Veronica, A., Jérémy, C., Lowe, M. A., Jong Woung, K., Pierre-Louis, T., Tolbert, S. H., et al. (2013). High-rate electrochemical energy storage through Li+ intercalation pseudocapacitance. Nat. Mater. 12, 518–522. doi: 10.1038/nmat3601
Wang, G., Xiong, X., Xie, D., Lin, Z., and Liu, M. (2018). Chemical activated hollow carbon nanospheres as a high-performance anode material for potassium ion batteries. J. Mater. Chem. A 6, 24317–24323. doi: 10.1039/C8TA09751H
Wang, J., Polleux, J., Lim, J., and Dunn, B. (2007). Pseudocapacitive contributions to electrochemical energy storage in TiO2 (anatase) nanoparticles. J. Phys. Chem. C 111, 14925–14931. doi: 10.1021/jp074464w
Wang, L., Yang, J., Li, J., Chen, T., Chen, S., Wu, Z., et al. (2019). Graphite as a potassium ion battery anode in carbonate-based electrolyte and ether-based electrolyte. J. Power Sources 409, 24–30. doi: 10.1016/j.jpowsour.2018.10.092
Wang, X., Han, K., Qin, D., Li, Q., Wang, C., Niu, C., et al. (2017). Polycrystalline soft carbon semi-hollow microrods as anode for advanced K-ion full batteries. Nanoscale 9, 18216–18222. doi: 10.1039/C7NR06645G
Wang, X., Weng, Q., Liu, X., Wang, X., Tang, D. M., Tian, W., et al. (2014). Atomistic origins of high rate capability and capacity of N-doped graphene for lithium storage. Nano Lett 14, 1164–1171. doi: 10.1021/nl4038592
Wang, X., Xu, X., Niu, C., Meng, J., Huang, M., Liu, X., et al. (2016). Earth abundant Fe/Mn-based layered oxide interconnected nanowires for advanced K-ion full batteries. Nano Lett. 17, 544–550. doi: 10.1021/acs.nanolett.6b04611
Wang, Y. (2017). Practical challenges in employing graphene for lithium-ion batteries and beyond. Small 1:1700099. doi: 10.1002/smtd.201700099
Wang, Y., Ding, Y., Pan, L., Shi, Y., Yue, Z., Shi, Y., et al. (2016). Understanding the size-dependent sodium storage properties of Na2C6O6-based organic electrodes for sodium-ion batteries. Nano Lett. 16, 3329–3334. doi: 10.1021/acs.nanolett.6b00954
Wang, Y., Feng, Z., Yang, S.-Z., Gagnon, C., Gariépy, V., Laul, D., et al. (2018a). Layered oxides-LiNi1/3Co1/3Mn1/3O2 as anode electrode for symmetric rechargeable lithium-ion batteries. J. Power Sources 378, 516–521. doi: 10.1016/j.jpowsour.2017.12.043
Wang, Y., Yang, S., You, Y., Feng, Z., Wen, Z., Gariepy, V., et al. (2018b). A high-capacity and long cycle life aqueous rechargeable lithium-ion battery with FePO4 anode. ACS Appl. Mater. Interfaces 10, 7061–7068. doi: 10.1021/acsami.7b18058
Wang, Z., Selbach, S. M., and Grande, T. (2013). Van der Waals density functional study of the energetics of alkali metal intercalation in graphite. RSC Adv. 4, 4069–4079. doi: 10.1039/C3RA47187J
Wen, Y., He, K., Zhu, Y., Han, F., Xu, Y., Matsuda, I., et al. (2015). Expanded graphite as superior anode for sodium-ion batteries. Nat. Commun. 5:4033. doi: 10.1038/ncomms5033
Wu, L., Buchholz, D., Vaalma, C., Giffin, G. A., and Passerini, S. J. C. (2016). Apple biowaste-derived hard carbon as powerful anode material for Na-ion batteries. ChemElectroChem 3, 292–298. doi: 10.1002/celc.201500437
Wu, X., Leonard, D. P., and Ji, X. (2017). Emerging non-aqueous potassium-ion batteries: challenges and opportunities. Chem. Mat. 29, 5031–5042. doi: 10.1021/acs.chemmater.7b01764
Xie, Y., Chen, Y., Liu, L., Tao, P., Fan, M., Xu, N., et al. (2017). Ultra-high pyridinic N-doped porous carbon monolith enabling high-capacity K-ion battery anodes for both half-cell and full-cell applications. Adv. Mater. 29:1702268. doi: 10.1002/adma.201702268
Xing, Z., Qi, Y., Jian, Z., and Ji, X. (2017). Polynanocrystalline graphite: a new carbon anode with superior cycling performance for K-ion batteries. ACS Appl. Mater. Interfaces 9, 4343–4351. doi: 10.1021/acsami.6b06767
Xu, Y., Zhang, C., Zhou, M., Fu, Q., Zhao, C., Wu, M., et al. (2018). Highly nitrogen doped carbon nanofibers with superior rate capability and cyclability for potassium ion batteries. Nat. Commun. 9:1720. doi: 10.1038/s41467-018-04190-z
Xu, Z., Liu, J., Chen, C., Potapenko, H., and Wu, M. (2019a). Hydrophilic binder interface interactions inducing inadhesion and capacity collapse in sodium-ion battery. J. Power Sources 427, 62–69. doi: 10.1016/j.jpowsour.2019.04.063
Xu, Z., Wu, M., Chen, Z., Chen, C., Yang, J., Feng, T., et al. (2019b). Direct structure–performance comparison of all-carbon potassium and sodium ion capacitors. Adv. Sci. 6:1802272. doi: 10.1002/advs.201970075
Yang, J., Ju, Z., Jiang, Y., Xing, Z., Xi, B., Feng, J., et al. (2018). Enhanced capacity and rate capability of nitrogen/oxygen dual-doped hard carbon in capacitive potassium-ion storage. Adv. Mater. 30:1700104. doi: 10.1002/adma.201700104
Zheng, Y., Wang, Y., Lu, Y., Hu, Y.-S., and Li, J. (2017). A high-performance sodium-ion battery enhanced by macadamia shell derived hard carbon anode. Nano Energy 39, 489–498. doi: 10.1016/j.nanoen.2017.07.018
Keywords: potassium-ion batteries, carbonaceous anodes, pseudo-capacitance adsorption, surface doping activation, kinetic analysis
Citation: Liu J, Xu Z, Wu M, Wang Y and Karim Z (2019) Capacity Contribution Induced by Pseudo-Capacitance Adsorption Mechanism of Anode Carbonaceous Materials Applied in Potassium-ion Battery. Front. Chem. 7:640. doi: 10.3389/fchem.2019.00640
Received: 08 July 2019; Accepted: 05 September 2019;
Published: 02 October 2019.
Edited by:
Min Zeng, Lanzhou Institute of Chemical Physics (CAS), ChinaReviewed by:
Dongliang Chao, University of Adelaide, AustraliaChao Wang, Massachusetts Institute of Technology, United States
Copyright © 2019 Liu, Xu, Wu, Wang and Karim. This is an open-access article distributed under the terms of the Creative Commons Attribution License (CC BY). The use, distribution or reproduction in other forums is permitted, provided the original author(s) and the copyright owner(s) are credited and that the original publication in this journal is cited, in accordance with accepted academic practice. No use, distribution or reproduction is permitted which does not comply with these terms.
*Correspondence: Mengqiang Wu, bXd1JiN4MDAwNDA7dWVzdGMuZWR1LmNu; Yuesheng Wang, d2FuZy55dWVzaGVuZyYjeDAwMDQwO2lyZXEuY2E=; Zaghib Karim, WmFnaGliLkthcmltJiN4MDAwNDA7aHlkcm8ucWMuY2E=
†These authors have contributed equally to this work